Abstract
Submarine groundwater discharge (SGD) is now recognized as an important coastal process affecting local and regional sources of solutes to coastal oceans. The objective of this study was to compare SGD estimates through Martinique Beach (Îles-de-la-Madeleine, QC, Canada) using different sampling techniques in order to quantify SGD fluxes of dissolved organic and inorganic carbon (DOC, DIC) exported to a coastal embayment. These fluxes were estimated using hydrogeological and geochemical methods, including direct measurement via seepage meters and hydrogeological estimation using Darcy’s law. Darcy estimates led to a fresh SGD flow ranging from 0.020 m3/s at the shoreline to 0.030 m3/s at the beach face. Direct measurements by seepage meters revealed higher SGD flows, with a mean value of 0.090 m3/s. These SGD flows were mainly composed of recirculating seawater flushing by the falling tide. The unconfined aquifer of Martinique Beach is a biogeochemically reactive zone, where DOC and DIC are nonconservative. The groundwater residence time (~32 days), low oxygen conditions (~20%) and high DOC concentrations (~ 2 mmol/L) represent a suitable environment for biogeochemical reactions to occur, and subsequently alter groundwater-borne carbon concentrations. We calculated a fresh-groundwater carbon load of 147 kg/d of DIC and 27 kg/d of DOC at the beach face. Even though recirculating seawater dominated the volume of total SGD, fresh SGD was an important carbon pathway, accounting for 12 and 20% of total DIC and DOC, respectively. This site-specific study is the first attempt to estimate volumetric and chemical groundwater fluxes to a coastal Canadian ocean, and demonstrates the strong interaction between fresh groundwater and coastal systems.
Les décharges d’eau souterraine (SGD pour submarine groundwater discharge) sont courantes en milieu côtier et sont maintenant reconnues comme des sources locales et régionales de solutés à l’océan côtiers. L’objectif de cette étude était de comparer différentes techniques pour estimer les SGD à travers la plage de la Martinique (Îles-de-la-Madeleine, QC, Canada) et ainsi pouvoir évaluer les flux de carbone organique et inorganique dissous (DOC et DIC) exportés à la côte. Des données aussi bien hydrogéologiques que géochimiques ont été utilisées pour évaluer ces flux, en utilisant notamment des mesures directes avec des chambres d’exfiltration (seepage meter) et des mesures hydrogéologiques en se basant sur la loi des écoulements de Darcy. Les estimations faites à partir de Darcy donnent des flux d’eau douce entre 0,020 m3/s à la ligne de rivage et 0,030 m3/s dans la zone de décharge de la plage. Les mesures directes donnent des valeurs plus élevées, autour de 0,090 m3/s mais ces flux incluent l’eau de mer de recirculation advectée par la pompe tidale. L’aquifère non confiné de la plage est aussi une zone biogéochimiquement active où le DOC et DIC montrent un comportement non-conservatif. Le temps de résidence de l’eau douce (autour de 32 jours), les faibles concentrations en oxygène dissous (DO ~20% de saturation) et les fortes concentrations en DOC (~2 mmol/L) permettent le développement de conditions idéales aux processus de respiration et à la transformation du carbone apporté par les écoulements souterrains. Ces transformations mènent à des flux souterrains de 147 kg DOC/j et 27 kg DIC/j dans la zone de décharge. Et même si l’eau de mer de recirculation domine le volume total de SGD dans ce site, l’écoulement d’eau douce est une importante voie de transport de carbone à l’océan et représente respectivement 12 et 20% des apports en DIC et DOC. Bien que ces résultats soient spécifiques au site d’étude, ils représentent la première tentative d’estimation des flux, qu’ils soient volumétriques ou chimiques, dans l’océan côtier canadien et soulignent la forte interaction entre les eaux souterraines et les milieux côtiers.
Introduction
Coastal aquifers constitute the major source of potable water for many regions throughout the world (Bear et al. Citation1999). Their importance as a fresh water source for coastal populations as well as the impacts of their submarine discharges to coastal zones highlight the need to implement an integrated water resource management plan in coastal zones. This is particularly important in island systems, where the connectivity between groundwater and seawater is strong. Submarine groundwater discharge (SGD) has been increasingly identified as an important process that coastal managers should consider in any water resources management plan (Moore Citation1996; Taniguchi et al. Citation2002; United Nations Educational, Scientific and Cultural Organization (UNESCO) Citation2004; McCoy and Corbett Citation2009). This is particularly important where booming coastal populations induce numerous changes in landscapes and ecosystems as well as increase pressure on coastal resources. In locations around the world, deteriorations in coastal water quality (e.g. bacterial proliferation, harmful algal blooms, hypoxia, acidification, fish and shellfish mortality) have been attributed to the quality and volume of groundwater discharge to the ocean (Valiela et al. Citation1978, 1980; Johannes Citation1980; Laroche et al. Citation1997; Paerl Citation1997; Gobler and Boneillo Citation2003; Hwang et al. Citation2005; Y.W. Lee and Kim Citation2007; Y.W. Lee et al. Citation2009; G. Kim et al. Citation2011). These disturbances alter coastal ecosystems and endanger species that have ecological, cultural and economic importance.
Moore (Citation2010, p. 60) defined SGD as “any and all flow of water on continental margins from the sea bed to the coastal ocean, with scale lengths of metres to kilometres, regardless of fluid composition or driving force.” SGD includes both fresh groundwater flowing directly to the sea and seawater recirculating through the seabed. SGD occurs in many environments along continental margins, usually as submarine springs or scattered seeps through permeable seafloor and beach sediments. This seeping occurs whenever the water table remains above sea level and coastal aquifer recharge is efficient (see Taniguchi et al. Citation2006 and references therein).
Sandy sediments make up more than 40% of the world’s coastlines (Riedl et al. Citation1972; Brown and McLachlan Citation2002) and are characterized by high to very high biogeochemical reaction rates despite their low particulate organic carbon content (Boudreau et al. Citation2001; Anschutz et al. Citation2009). Multiple physical forces that drive subsurface flow and residence times control the reaction rates and thus the transformations in this active biogeochemical zone (e.g. Boufadel Citation2000; Horn Citation2002, 2006; Michael et al. Citation2005, 2011; Charette et al. Citation2007; Robinson et al. Citation2007; Cable and Martin Citation2008). Santos et al. (Citation2012) identified at least 12 independent drivers that include both terrestrial (e.g. hydraulic gradient, seasonal oscillation of the water table) and marine (e.g. wave and tidal pumping, ripple and bed form migration, bioirrigation) processes that interact in complex ways. All of these processes force flow across the water–sediment interface, albeit at different spatial and temporal scales, and consequently influence the export of groundwater-borne, recirculated and newly formed compounds to the sea. The chemistry of SGD changes during its passage through the aquifer and permeable coastal sediments, but how these transformations affect the concentrations and exported fluxes of groundwater-borne carbon is not yet well understood. In particular, carbon is a key element of coastal eutrophication, and the exported fluxes of dissolved inorganic and organic carbon (DIC and DOC, respectively) via SGD are still not well quantified (Caï et al. Citation2003; Goñi et al. 2003; Beck et al. Citation2007; Dorsett et al. Citation2011; Moore et al. Citation2011; Cyronak et al. Citation2013. Kim et al. Citation2013; Maher et al. Citation2013). Such quantifications are vital for managing coastal ecosystems, which include the groundwater–ocean continuum. This is particularly important in northern systems because these regions are experiencing – and are expected to continue to experience – important changes in hydroclimatic and terrestrial carbon storage (Hinzman et al. Citation2005; Roulet et al. Citation2007).
In the Îles-de-la-Madeleine archipelago, which is located in the southern Gulf of St. Lawrence (QC, Canada; Figure a, b), groundwater is the only source of fresh and drinking water. The absence of major streams limits the discharge of groundwater to continental surficial ecosystems, and groundwater rapidly flows to coastal ecosystems (residence time < 1 year; Madelin’Eau Citation2004). This is a typical coastal aquifer system where the quality of groundwater can affect the ecological health of adjacent embayments.
Figure 1. (a) Location of Quebec, (b) the Îles-de-la-Madeleine and (c) the archipelago’s main island (Cap-aux-Meules). The study area (from le Chemin des Chalets to Gros-Cap) and Martinique Beach are shown. The dashed line corresponds to the cross section presented in Figure . The simplified geology was adapted from Brisebois (Citation1981) (data source ©Gouvernement du Québec).
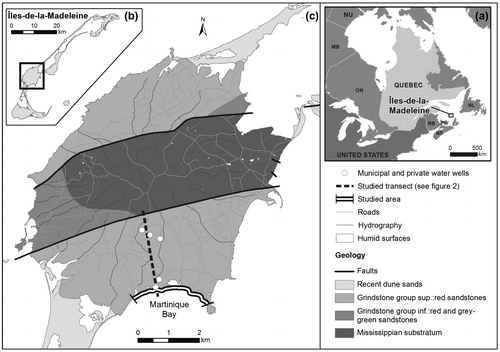
The objective of this study was to compare SGD estimates using two different sampling techniques in order to quantify SGD fluxes of DOC and DIC exported to the coastal zone through a sandy beach. More specifically, hydrogeological and geochemical data are used to estimate carbon fluxes. Data were collected by direct measurement via seepage meters, and hydrogeological estimations were made using Darcy’s law. This study combines knowledge of marine chemistry and hydrogeology, and proposes an integrated view of water resources in the groundwater–ocean continuum.
Material and methods
Study area
The Îles-de-la-Madeleine are part of the Maritimes Permo–Carboniferous Basin included in the Northeastern Appalachian Geological Province (Figure ; Brisebois Citation1981). The archipelago comprises a group of 15 islands, seven of which are joined as tombolos. The main island is part of the Mississippian–Windsor group, which is an interbedded assemblage of volcanic and volcanoclastic rocks flanked by sandstones of the Permian Inferior period (the Cap-aux-Meules Formation; Brisebois Citation1981). This formation constitutes the main aquifer in the archipelago; the mean transmissivity (~4 × 10−3 m2/s) and mean recharge (~230 mm/y, i.e. ~25 to 30% of the annual snow and rain precipitation; Leblanc Citation1994; Madelin’Eau Citation2004) support high pumping rates. Groundwater flows from the central volcanic core recharge through the unconfined Permian sandstone aquifer (Figure ) and discharges to the sea, both directly and through overlying Quaternary deposits.
Figure 2. (a) Schematic cross section through the main geologic units of the main island (Cap-aux-Meules). The municipal (P4, P5, PU6) and private (PM) water wells are located along the geological transect. The position of the salt water/fresh water interface (shaded line) was estimated according to the Ghyben–Herzberg relation based on mean annual piezometric levels recorded in the municipal and private wells (corrected to mean sea level). (b) View of the sampling transect in Martinique Beach showing locations of piezometers (Pz1–3), multi-level samplers (M1–7), and seepage chamber deployments. The different morphology units of the beach also are reported.
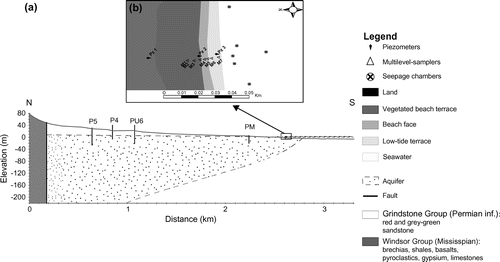
Most of the archipelago’s coasts are sandy barrier systems with fine-grained sands resulting from erosion of Holocene deposits and eolian transport. Sand grain size is about 300 μm, and sediments are mainly composed of silicate fragments (Chaillou et al. Citation2014). The underlying sandstone aquifer consists of fine red-orange sands (~100 μm) composed of silicate and aluminosilicate with iron (Fe)-coated silicate grains. The archipelago is located near an amphidromic point (mean and maximum tidal ranges are 0.7 m and 1.1 m, respectively), with mixed semi-diurnal and diurnal tides. The wave regime is characterized by locally generated waves with a mean height of 0.87 m (Owens and Frobel Citation1977).
Martinique Beach is a typical sandy barrier system on the southwest coast of the archipelago. It has been classified as a low-energy beach with a low relative tidal range, indicating wave control of beach morphology (Masselink and Short Citation1993; Jackson et al. Citation2002). A high-definition elevation profile resulting from differential global positioning system (DGPS) surveys revealed three morphologic zones (see beach morphology in Figure b). These zones include a vegetated beach terrace on the backshore (~10 m wide) composed of fine-grained sands, a beach face composed of fine-grained sands with a mean slope of 6° (~15 m wide), and a large low-tide terrace (~50 m) composed of very fine sands with a lower gradient (~2°) and persistent micro-topographic features under non-storm wave conditions; all of these are characteristic of low-energy beaches (Jackson et al. Citation2002). The discharge zone is not well characterized, but a recent radon-222 (222Rn) survey suggests a narrow discharge zone (~100 m) from the beach face (Lemay-Borduas et al. Citation2014). In the absence of surface streams, a recently developed groundwater flow model shows that ~96% of the fresh groundwater volume seeps to the coastal ocean via SGD each year, leading to discharges of around 4300 m3/d in Martinique Bay (Madelin’Eau Citation2004; Chaillou et al. Citation2013).
Approach
Two approaches were used to investigate SGD through the sandy beach of Martinique Bay to quantify exported fluxes of DIC and DOC. These techniques included groundwater flow estimations using Darcy’s law and direct measurements via seepage meters installed in the low-tide terrace between 20 May and 7 June 2013. Hydrogeological data from municipal and private water wells were used to estimate the regional groundwater flow perpendicular to the shoreline (Figure a; Madelin’Eau Citation2004, Citation2007, Citation2011). Based on Darcy’s law, and assuming the hydraulic gradient component parallel to the coastline is null, groundwater fluxes in the Permian aquifer (qinland in cm/d) were calculated as:(1)
where K is the hydraulic conductivity of the Permian aquifer and gradh is the component of the hydraulic gradient perpendicular to the shoreline. The fresh groundwater fluxes were also estimated by Darcy’s law in the beach aquifer (qbeach in cm/d) using data from three monitoring piezometers placed perpendicular to the shoreline (Figure b). Seepage meter experiments were conducted in the low-tide terrace of the beach (see locations in Figure b) to measure the volume of water that seeps from the seabed. Despite criticisms concerning pressure-induced flow (see Shinn et al. Citation2002 and references therein), this technique provides a flux at a specific time and site from a limited seabed area (Corbett and Cable Citation2003; Burnett et al. Citation2006). The two techniques have advantages and disadvantages, but their combination provides valuable insight into the SGD regime, which includes both fresh groundwater and recirculating seawater (see Burnett et al. Citation2006 and references therein).
Sample collection
Three piezometers were inserted, in the beach terrace (PZ1), the beach face (PZ2) and the low-tide terrace (PZ3; Figure b). Piezometers were constructed of 2.5-cm polyvinyl chloride (PVC) pipes (Ø = 5 cm) and were installed to a depth of 2.2 m using a manual auger; a 10-cm solid PVC riser was used for the casing. Water levels were monitored manually and with automated level loggers (HOBO models). Tides and waves were recorded at Pz3 and Pz2, and water table levels were corrected to obtain mean values of the water table over the sampling period and to estimate the component of the hydraulic gradient perpendicular to the shore. Guelph permeameter measurements (N = 15) were conducted to estimate local hydraulic conductivity of the beach’s surficial sandy aquifer. Values are reported in Table .
Table 1. Summary of hydrogeological values used to estimate groundwater fluxes and submarine groundwater discharges assuming a 1200-m sandy beach shoreline. K is the hydraulic conductivity (in m/d); gradh is the change in head per unit length in the direction of flow considering an isotropic aquifer. The groundwater fluxes are reported in term of q (in cm/d) and Q (in m3/s) based on Darcy’s law. Mean values and standard deviations (mean ± SD) are presented. Data from Madelin’Eau (Citation2004) were used to estimate groundwater fluxes in Permian aquifer, in inland water wells.
The seepage meter design followed D.R. Lee (Citation1977), using the top 30 cm of a standard 55-US gallon (208-L) plastic drum fitted with a sample port and a plastic collection bag. Seepage meters were carefully pushed to a depth of 15 cm into the seabed and allowed to equilibrate for ~4 hours. Seepage bags (2000 mL) were prefilled with 100 mL of seawater and the air was removed; these bags were deployed for 1.5 to 4.0 hours and then carefully removed. Collected water volumes were measured to the nearest millilitre (using 1000-mL graduated cylinders) to determine the groundwater seepage fluxes (i.e. the collected volume divided by the time interval and the seepage meter area). Because seepage meters can be deployed only in calm wave and wind conditions, only seven experiments were performed (22, 24, 28, 31 May; 2, 3, 5 June 2013). Seepage meters were deployed at different locations in the low-tide terrace of the beach, a few metres from the shoreline (Figure b).
The flux of exported carbon associated with groundwater discharge was calculated based on DIC and DOC concentrations measured during the transit of groundwater to the ocean. Water samples were collected during the low spring tide period in May and June 2012 and 2013. A ~35-m transect of multi-level samplers (M1–7; Figure ) was installed on the beach. The multi-level samplers consisted of 2.5-m PVC pipes with eight pores connected to flexible Tygon® tubing, as described in Martin et al. (Citation2003). Multi-level samplers were designed to collect water at 10, 30, 50, 80, 110, 150, 190 and 230 cm below the beach surface. In 2012, beach groundwater samples were collected at M1, M2, M3 and M6 (Figure b). In 2013, the focus was the intertidal zone, where beach groundwater and recirculating seawater most likely discharge (M3 to M7; Figure b). Beach groundwater was pumped with a peristaltic pump into an on-line flow cell where specific conductivity (SpCond) and dissolved oxygen saturation (DO) were monitored using a calibrated multiparametric probe (600QS, YSI Inc.). After these parameters stabilized, groundwater samples were collected and preserved for DOC and DIC analysis. DOC samples were taken using 60-mL acid-clean syringes and rapidly filtered (Whatman GF/F 0.7-μm filters), acidified to pH = 2, and preserved in acid-clean glass tubes. All filters and glass tubes had been pre-combusted at 500°C for 4 h. Samples were kept in the dark at 4°C until analysis. Samples for δ13C-DIC were collected by filling 250-mL glass bottles with no headspace, preserved with 0.5 mL of saturated HgCl2 and stored at 4°C. δ13C-DIC was analyzed for the 2012 and 2013 samples while DIC concentrations were measured only from samples collected at M3, M4 and M7 in 2013.
The fresh and marine end-member concentrations of DOC and DIC were systematically measured. Samples from the Permian sandstone aquifer were collected as described above from private and municipal water wells located 50 to 2000 m inshore from the multi-sampler transect (see PM, P5, P4 and PU6 wells in Figure a). Deep samples of bay water (~50 cm above the seabed) were collected 1 km offshore in Martinique Bay. The water samples were collected from a small boat using a submersible pump connected to an on-line flow cell.
DOC samples were analyzed by high temperature combustion (HTC) using a Total Organic Carbon (TOC) analyzer (TOC-Vcpn; Shimadzu) based on the method proposed by Wurl and Sin (2009). The detection limit is usually 0.05 mg/L and the analytical uncertainties were < 2% for concentrations higher than 1 mg/L. Dissolved inorganic carbon (DIC or ΣCO2 = CO2 + HCO3– + CO32–, also called total carbonate) were analyzed using the flow injection analysis (FIA) technique based on the method proposed by Hall and Aller (Citation1992). A gas-permeable membrane is used to move CO2 from acidic reagent streams to a basic receiving stream and conductivity detector. The calibration was done using a reagent-grade sodium bicarbonate (NaHCO3) solution, and the conductivity response was linear for samples over DIC concentrations from < 0.2 to 20 mmol/L with a precision of ~1%. The δ13C-DIC samples were analyzed using a gas chromotography coupled to an isotope ratio mass spectrometry (GC-IRMS) (GV Instruments). An internal calibration was performed using two internal standards to obtain a two-point calibration. Results are reported in parts per thousand in the δ notation relative to relative to Vienna Pee Dee Belemnite (VPDB) (standardized to TS-Limestone [NEBS19] and lithium carbonate [LSVEC] International Atomic Energy Agency (IAEA) referenced materials) with a precision of ± 0.1‰.
Results
Groundwater flux and SGD estimates
The survey of water table levels in the different transect piezometers revealed that the hydraulic head difference perpendicular to the shore is different in the inland part of the transect and in the beach. The multiannual average component of the hydraulic gradient from P5 to PU6 has been estimated at 0.0035 (Madelin’Eau Citation2004, Citation2007). During the survey in June 2013, the head gradient gradh (i.e. the change in head per unit length in the direction of flow considering an isotropic aquifer) is one order of magnitude higher in the beach aquifer, with values between 0.037 and 0.012. The mean conductivity value K of the unconfined Permian sandstone aquifer is 1.8 m/d (Madelin’Eau Citation2004, Citation2007). In surficial beach sand sediments, the K values measured with the Guelph permeameter varied between 7 and 20 m/d, with a mean value of 11.4 ± 4.4 m/d. The spatial variability of K in the beach sediments results from the heterogeneity of surficial sediment: Chaillou et al. (Citation2014) reported the presence of an old forest soil layer on top of the sandstone aquifer caused by a recent sea-level rise. The range of values is consistent with those reported for similar lithology elsewhere on the Îles-de-la-Madeleine archipelago (5–9 m/d; Sylvestre Citation1979).
In the landward part of the studied transect, in the Permian aquifer, qinland varied between 0.7 and 3.6 cm/d, with a mean value of 2.1 cm/d. As fresh water approaches the coastline, groundwater flux is expected to increase because the groundwater flows through a smaller area. Using hydraulic conductivity from the sandy beach, higher qbeach fluxes were calculated: values ranged from 14 to 42 cm/d, with an average of 30.4 ± 11.8 cm/d. Here residence time is defined as the time required for groundwater to transit horizontally through the system, in a manner similar to surficial estuaries. If the beach material is assumed to be homogeneous and isotropic (as is often assumed in flow modeling studies; see e.g. Raubenheimer et al. Citation1999 and Abarca et al. Citation2013), the maximum residence time in the surficial sediment is 32 days for a beach transect of 35 m (from M1 to M7; Figure b).
To convert these results to volumetric flux, which is the discharge per unit width of the aquifer (in m3/s), the cross-sectional flow area was determined using global positioning system (GPS) measurements of 1200 m of shoreline, from the sandy beach east of Le Chemin de la Martinique to the rocky shore of Gros Cap (Figure c). Furthermore, based on the Ghyben–Herzberg and Glover relationship (Cooper Citation1964), the fresh water/salt water interface is reached about 73 m below the water table of the aquifer at the PM well (the well nearest the coastline; Figure a). Hence, a flow depth of 73 m is used to estimate the freshwater flux at the coastline. The volumetric groundwater flux from the Permian aquifer to the shoreline (Qinland) is estimated to be between 0.007 and 0.036 m3/s, with a mean flux of 0.021 m3/s. Also based on the Ghyben–Herzberg and Glover relationship (Cooper Citation1964), the top 3.2 m of the aquifer at the top of the intertidal zone (Pz2) is fresh (except for the narrow surficial saltwater lens). Hence, we used a depth of 3.2 m to estimate the volumetric groundwater flux through the beach face in May 2013. This led to a beach flux (Qbeach) between 0.008 and 0.046 m3/s, with a mean value of 0.029 ± 0.009 m3/s.
Direct measurements revealed a mean seepage flux (qseep) of 7.5 cm/d across the low-tide terrace seabed (Table ). Seepage fluxes are not uniform over time: they tend to increase with the falling tide to values > 10 cm/d, and to decrease to ~3 cm/d at low and rising tides. However, given the limited number of measurements (N = 7), no clear relationship was observed between seepage fluxes and tidal levels. Assuming a 1200-m shoreline and a 100-m discharge area (Lemay-Borduas et al. Citation2014), SGD is estimated to be between 0.041 and 0.130 m3/s, with an average value of about 0.085 ± 0.046 m3/s. Table summarizes these results.
Groundwater chemistry
End-members
Conductivity of the shoreline ocean water, i.e. the marine end-member, was measured at ~47 mS/cm, and the temperature was around 11°C (Chaillou et al. Citation2014). The dissolved oxygen (DO) saturation was > 100%, indicating high saturation due to wave and wind activity. Dissolved carbon concentrations were low, with DOC between 0.13 and 0.16 mmol/L and DIC between 2.1 and 2.5 mmol/L. The δ13C-DIC was slightly more variable, with values from –4.2 to –7.1‰ (mean –5.65‰). Groundwater conductivity in municipal and private wells, i.e. the freshwater groundwater end-member, was around 0.3 mS/cm, and temperature was measured at 7°C (Chaillou et al. 2024). DOC measured in well water was low, 0.30 ± 0.08 mmol/L, and minimal values of DIC were between 0 and 0.4 mmol/L. The δ13C-DIC was variable, with values from +8.0 to –1.9‰ (mean +3.05‰).
Beach groundwater
Two-dimensional profiles of beach groundwater chemistry are presented in Figure . The sample depths in these profiles are relative to mean sea level (i.e. 0 m depth), and contour lines were derived by the linear spatial interpolation (kriging method) of data points. The interpolation model adequately fits the empirical data set (95% confidence level). The two-dimensional conductivity profiles revealed the presence of fresh groundwater (conductivity < 5 mS/cm) in the landward part of the transect. The fresh groundwater lens diminished gradually towards the seaward discharge region below the intruding narrow saltwater lens (conductivity > 20 mS/cm) located high in the intertidal zone. Vertical conductivity profiles revealed limited mixing between the fresh groundwater layer and the surficial saltwater lens, which is characteristic of a microtidal system dominated by fresh groundwater discharge (Moore Citation1999; Robinson et al. Citation2007; Heiss and Michael Citation2014). While groundwater was suboxic, with DO around 20% saturation, the surficial saltwater lens was the most oxygenated layer, with DO saturation > 60%, indicating inputs of oxygen from seawater due to mixing.
Figure 3. Conductivity (mS/cm; a, c), and dissolved oxygen saturation (%; b, d) in the beach groundwater. The left panels (a, b) present data measured in 2012 along a 35-m transect of multi-level samplers; the right panels (c, d) present data measured in 2013 in the intertidal zone (dashed rectangle in a and b panels). The beach morphology has been done by differential global positioning system (DGPS) measurements. The depth is relative to mean sea level (i.e., 0 m depth) and the contour lines were derived by linear spatial interpolation (kriging method) of the data. The interpolation model reproduced the empirical data set very well (95% confidence level). Data were modified from Chaillou et al. (Citation2014).
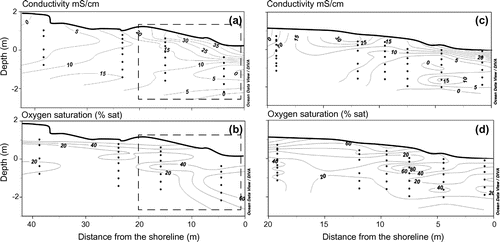
The concentrations of DOC and, DIC, and the δ13C-DIC, are plotted against conductivity (Figure a–c). As in surficial estuaries, carbon distribution in the beach transect can be examined considering the theoretical conservative mixing (or theoretical dilution line, TDL), where salinity (or conductivity) is used as the conservative property and the composition of the two mixing end-members is assumed to be constant (Boyle et al. Citation1974). At the inland end of the groundwater transect, i.e. samples collected at M1 and M2, DOC was > 1.6 mmol/L (maximum 5.5 mmol/L; Figure a), which is an order of magnitude greater than the fresh groundwater end-member. DOC concentrations generally decreased toward the discharge, although the distribution was patchy with some hotspots. For example, high concentrations of DOC were measured in the mid-region of the transect between M3 and M4 at 1 m below surface (DOC ~1.6 to 2.5 mmol/L; Figure a). In the intertidal zone (i.e. M3 to M7), DOC concentrations (~0.2 to 1.2 mmol/L) were also elevated relative to the marine end-member. There was clearly no relationship between DOC concentration and measured conductivity, and most of the samples were higher than the linear mixing model would predict (i.e. the TDL between the two end-members; see Figure a). For DIC, most of the measured concentrations were higher than the end-member concentrations (Figure b), with peaks in the first 30 cm below the beach surface in the intertidal zone (DIC > 10 mmol/L, Figure b). This region corresponded to the position of the surficial saltwater lens. Farther below the surface, concentrations decreased drastically to minimal values (DIC ~0–0.9 mmol/L), which were similar to those of the fresh groundwater end-member. The beach groundwater δ13C-DIC was greatly depleted relative to end-members, although values were highly variable through the transect (Figure c). δ13C-DIC ranged from –34‰ in the upland end of the groundwater transect to values > –5‰ in the top of the intertidal zone. In the bottom part of the transect, at depths deeper than 80 cm below the surface, δ13C-DIC was around –25‰. Data obtained in 2012 and 2013 are consistent, with similar values through the beach transect. Because the DIC concentrations of the two end-members differ substantially, the mixing line is curvilinear (details of the mixing equation are given in the caption of Figure ). In this system, δ13C-DIC values are consistently more negative than would be expected with conservative mixing.
Figure 4. Mixing plot of beach groundwater dissolved organic carbon (DOC, a), dissolved inorganic carbon (DIC, b), and isotopic sigmature of dissolved inorganic carbon (δ13C-DIC, c) relative to beach groundwater conductivity for 2012 (dots) and 2013 (triangles). The value ranges for the two end-members (i.e., fresh groundwater and marine) and the theoretical dilution line (TDL; black line) are also reported. Concerning the isotopic signature of dissolved inorganic carbon (δ13C-DIC): because DIC concentrations of both end-members differ substantially, the conservative mixing between fresh groundwater and seawater is not linear. It follows a curvilinear mixing relationship based on the following equation:
where S is the sample salinity, is the DIC concentration and stable isotope composition of the freshwater end-member, and
is the DIC concentration and stable isotope composition for the marine end-member.
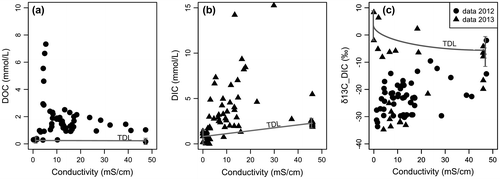
Figure 5. Schematic diagram of the hydrogeologic flow at the Martinique Beach site, indicating the potential end-member contribution to volumetric (in bold, unit: m3/s) SGD. Chemical fluxes of dissolved organic carbon (DOC) and dissolved inorganic carbon (DIC) are reported in kg/d. Diagram is not to scale. The tidal range is reported. The dashed line indicates the position of the fresh/saltwater lens. The dotted line is the position of the water table level. Weight of arrows is representative of relative flow strength.
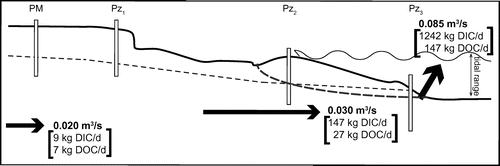
Discussion
A number of techniques have been developed for quantifying SGD (Burnett et al. Citation2006; Moore et al. Citation2006; Martin et al. Citation2007). Here, SGD fluxes have been calculated in two ways: (1) the hydrological approach based on Darcy’s law, which accounts only for the freshwater discharge component in the Permian aquifer and in the unconfined shallow beach aquifer; and (2) using seepage meters, which measure total SGD fluxes at the discharge face of the beach. In general, direct measurements using seepage meters are higher than estimates based on water balance and Darcy’s law calculations. Most of this difference has been attributed to the integration of recirculating seawater. Our hydrological analysis led to a freshwater SGD estimate, Qinland, of 0.021 m3/s across the 1200 m of sandy beach shoreline of Martinique Bay. This rate assumes a uniform hydraulic conductivity (K) and an isotropic shallow aquifer. Based on the same approach, Qbeach was estimated at 0.029 m3/s. These results agree quite well with the fresh groundwater flux estimate based on the mass-balance approach in the same area (i.e. 0.020 m3/s; Madelin’Eau Citation2004). The mass balance was based on an average recharge rate to the water table, assuming 36% of the annual average precipitation. Thus, using both Darcy’s law and a mass-balance approach, the fresh groundwater fluxes to the shoreline are equivalent.
Groundwater flux was also estimated by direct measurements during the spring tide to capture maximum outflow from the beach. Because of the microtidal conditions, the effect of tide on SGD would be expected to be minor. The volumetric fluxes obtained by seepage meters at the Martinique Beach site are the highest fluxes measured over the sampling period (Table ). High seepage rates have also been recorded in several other microtidal sites, including western Australia (Loveless and Odham 2010), the Mediterranean Sea (Weinstein et al. Citation2011), Cape Cod, Massachusetts (Mulligan and Charette Citation2006) and Turkey Point, Florida (Santos et al. Citation2009). At the Martinique Beach site, freshwater SGD estimated using Darcy’s law represents ~25 to 35% of total volumetric additions, indicating that continental drivers (likely water table level) may control SGD at this site. During seepage meter measurements, conductivity was measured in water that had seeped through the seabed. In addition, ambient coastal seawater was collected at the start and end of the deployment. The goal was to capture freshening events and to estimate the volume of seeped fresh groundwater. Conductivity measurements of water collected within seepage bags showed essentially the same values as those from ambient seawater samples. This is not surprising considering the time needed for the exchange of seawater in the seepage meter: with an average Qseep of 7.5 cm/d and assuming a seepage volume of ~65 L (0.24 m × 0.27 m2 = 0.065 m3), it would take more than 3 days. This flushing time seems consistent with our observations and can explain why we observed no change in the salinity. Thus, discrimination of the fresh and marine end-members was not possible in these samples.
Figure proposes a schematic diagram of the hydrologic flow at Martinique Beach, that includes fresh groundwater flows estimated using Darcy’s law and marine SGD derived from tidal pumping and recirculating seawater through the 35-m transect. To compare our SGD estimates with other studies, the volumetric fluxes were divided by the length of shoreline (1200 m). This leads to shore-normal estimates ranging from 1.5 m3/m/d (Qinland) to 2.1 m3/m/d (Qbeach) for fresh SGD and 6.1 m3/m/d (Qseep) for total SGD. These shoreline fluxes show magnitudes similar to those at other sites, such as Waquoit Bay (Cape Cod, Massachusetts, 4–5.6 m3/m/d, Mulligan and Charette Citation2006), the Gulf of Thailand (3.7–7.8 m3/m/d, Burnett et al. Citation2007), western Australia (2–30 m3/m/d, Loveless and Oldham Citation2010; and 2.5–8 m3/m/d, Smith and Nield Citation2003), Shelter Island (Long Island, New York, 0.4–17 m3/m/d, Burnett et al. Citation2006) and Turkey Point (Florida, 0.7–22 m3/m/d, Santos et al. Citation2009). At these locations, however, fresh groundwater discharges are a minor component, accounting for only ~5% of total SGD fluxes.
The site-specific evaluation of SGD provides an opportunity to better assess steep spatial gradients in biogeochemical reactions and the resulting impacts SGD may have on biogeochemical fluxes to the coastal embayment. SGD in the unconfined aquifer of Martinique Beach comprises a combination of fresh groundwater and recirculating seawater; thus, the sources of carbon could be diverse. Carbon in the groundwater in Martinique Beach can be classified by its behaviour relative to theoretical conservative mixing between seawater carbon concentrations as one end-member and inland carbon concentrations from municipal and private wells to represent the other end-member. The measured concentrations of DOC and DIC (Figure a and b) revealed that the gradient in groundwater-borne carbon from land to ocean is not a simple dilution between the two end-members. In other words, both DOC and DIC showed clear nonconservative patterns in Martinique Beach, with significant internal inputs. These nonconservative behaviours along the groundwater flowpath are important in the interpretation of the ecological impact of SGD (Johannes Citation1980; Valiela et al. Citation1980), and the calculation of chemical fluxes using samples from the inland wells probably results in significant deviations from the true chemical flux. Local DOC production has been recorded at other sites, including Turkey Point Beach (Gulf of Mexico, Florida; Santos et al. Citation2009), Hampyeong Bay (Korea; T. Kim et al. Citation2012) and North Inlet (South Carolina; Goñi and Gardner Citation2003). Data on the transit of groundwater-borne DIC are scarce in the literature. Caï et al. (Citation2003) demonstrated that a two end-members mixing model could not explain the high DIC values measured in North Inlet groundwater. More recently, Maher et al. (Citation2013) also reported higher DIC along groundwater transit in an Australian mangrove tidal creek. Other studies have shown that tidal sands are important biogeochemical reactors within which organic matter is mineralized, producing metabolites and DIC (Boudreau et al. Citation2001; Anschutz et al. Citation2009; Moore et al. Citation2011; Charbonnier et al. Citation2013).
DOC was produced at the inland end of the transect, at the same location where organic-rich paleosol was found (Chaillou et al. Citation2014). Forest soils and the stumps of trees killed by seawater are commonly found on the US and Canadian Atlantic coast, on the shores of salt marshes, and on the seaward side of eroding barrier beaches (Grant Citation1970; Dredge et al. Citation1992; Dubois Citation1992; Gehrels Citation1994). The rapid rise in sea level along the coasts of Atlantic Canada during the middle-to-late Holocene buried terrestrial systems with tidal sediments, since sedimentation kept pace with the rising level of high tide. In Martinique Beach, this old enriched horizon of particulate organic carbon (POC) is characterized by a depleted 13C signature (~ –33‰; M. Couturier, unpub. data) and high carbon to nitrogen (C/N) ratio (> 25; M. Couturier, unpub. data). DOC is a key intermediate in the overall process of POC degradation (Kristensen and Hansen Citation1995; Robador et al. Citation2010; Komada et al. Citation2012), but little is known about the mechanism of porewater DOC accumulation in suboxic–anoxic sediment. Here, it is still unclear whether the DOC originated from hydrolysis of old POC from the soil horizon or if it was from other sources, such as marine particles or algal detritus. Isotopic investigations should provide further insight into the origin and reactivity of porewater DOC. Whatever its origin, DOC produced locally was transported seaward by groundwater flow. The vertical decline in oxygen saturation observed below the saltwater lens, the maximum residence time in the beach transect of ~32 days in the beach system and the high DOC concentrations were deemed suitable for biogeochemical reactions to occur. The high DIC concentrations measured in the discharge zone combined with high dissolved Fe concentrations (and other reduced compounds such as NH4+ and Mn2+; M. Couturier, unpub. data) as well as the characteristic H2S odour during sample collection previously reported by Chaillou et al. (Citation2014) support the idea that suboxic to anoxic mineralization processes are likely to occur along the flowpath.
Based on total alkalinity and pH measurements, Chaillou et al. (Citation2014) discussed the different ways that DIC may be produced in Martinique Beach. The introduction of fresh meteoric groundwater and the subsequent dissolution of carbonate minerals in addition to the chemical and biological processes of respiration may lead to local DIC production, as observed in other beach systems (Caï et al. Citation2003; Charbonnier et al. Citation2013 Maher et al. Citation2013). δ13C-DIC was depleted in the beach groundwater (–34 to –25‰) when compared to the marine and fresh groundwater end-members (–5.65 and +3.05‰, respectively). Since carbonate minerals tend to have δ13C values of ~2‰ (Weber and Woodhead Citation1969; Eadie and Jeffrey Citation1973), these depleted values may indicate an organic carbon source and may support the assumption that suboxic respiration, in the form of sulfate reduction, was the main DIC source. As seen in Figure c, the δ13C-DIC data at Martinique Beach largely confirm the nonconservative behaviour of DIC in this subterranean estuary. This pattern is consistent with the earlier hypothesis that intense mineralization in this system may increase DIC and explain DOC loss along the groundwater flow path. Given that the excess DIC should be isotopically similar to the organic matter from which it is derived (which could have various sources, including buried organic-rich horizons, with δ13C values between –32 and –34‰), this should result in the addition of 13C- depleted DIC.
The ratio of organic carbon to inorganic carbon exported to the coastal ocean could have a significant effect on the role as “source” or “sink” of atmospheric CO2. Robust estimates of DIC and DOC fluxes are needed on a site-specific scale to obtain accurate regional and global estimations. In nonconservative systems, however, the determination of appropriate DOC and DIC concentration end-members for flux calculations is not straightforward (Beck et al. Citation2007, 2015; Dorsett et al. Citation2011; Cyronack et al. 2013). The fresh SGD-derived fluxes of carbon are calculated as the product of the concentration of the fresh groundwater end-member and the volume of fresh groundwater discharge (Qinland). Assuming that the well closest to the site of discharge (i.e. PM well) represents the best estimate of the nearshore groundwater flux, the carbon load exported by fresh groundwater was 9 kg/d of DIC and 7 kg/d of DOC. In this calculation, biogeochemical transformation during transit from the aquifer to the ocean is completely neglected. Using the fresh groundwater flow estimated in the beach (Qbeach = 0.029 m3/s) and the mean DOC and DIC concentrations measured in the beach’s discharge zone (i.e. mean concentrations in the first 30 cm of M7), the carbon load was 147 and 27 kg/d of DIC and DOC, respectively. These latter fluxes are 4 to 16 times higher than carbon fluxes derived from nearshore fresh groundwater, and highlight the importance of considering the geochemical behaviour of carbon along the flow path.
For estimating the total SGD carbon fluxes, mean carbon concentrations in the saltwater lens and surficial sediments were used as the recirculating seawater carbon end-member. Total DIC and DOC fluxes exported by total SGD (Qseep = 0.085 m3/s) could be around 1242 and 147 kg/d, respectively. Fresh groundwater discharges in the beach are an important pathway of carbon and account for 12% of the total DIC and 20% of the total DOC exported to seawater. In contrast, groundwater-borne carbon accounted for less than 1 and 5% of total DIC and DOC fluxes, respectively. Fresh groundwater here is clearly not the source of carbon, but it is a pathway for DIC and DOC produced in the beach to reach the coastal ocean. The chemical SGD fluxes are reported in the schematic hydrogeological flow model in Figure .
Only a few papers have considered carbon fluxes in SGD, particularly as it transits through a sandy beach. In Turkey Point (Florida), Santos et al. (Citation2009) reported SGD-derived DOC fluxes 2 times smaller than our values for similar volumetric SGD estimates. In the Okatee Estuary (South Carolina), Moore et al. (Citation2006) measured high inputs of C – 1440 kg DOC/d and 16 800 kg DIC/d – based on flows greater than 1 m3/s. More recently, Liu et al. (Citation2011) measured SGD contributions of DIC that were a hundred times greater than those in Martinique Bay, but their study site was located in a carbonate system on the northern South China Sea, where summer monsoons cause high fresh groundwater flows (~ 2.5 × 103 m3/s). Thus, it is reasonable to assume that sandy-beach SGD-derived fluxes contribute to regional and global carbon budgets. Once reaching coastal waters, carbon could be consumed by biological production; thus, the net effect of SGD-driven DIC and DOC fluxes would be modulated. This modulation will depend on the origin and reactivity of the exported carbon. Further investigations are needed to determine the source of carbon in Martinique Bay, as this may clarify whether carbon in SGD is from recycled marine matter or degraded terrestrial organic carbon.
Conclusion
In the studied zone, Darcy estimates led to an SGD flow that ranged from 0.021 m3/s (or 1.6 m3/m/d) at the shoreline to 0.029 m3/s (or 3.8 m3/m/d) at the beach face. Direct measurements by seepage meters revealed higher SGD flows, with a mean value of 0.085 m3/s (or 6.1 m3/m/d). These fluxes were consistent with each other and with previous mass-balance estimates based on an average recharge rate to the water table and average annual precipitation. Based on total SGD volumetric fluxes, the total DIC and DOC fluxes exported could be around 1242 and 147 kg/d, respectively. Because the unconfined aquifer of Martinique Beach is a biogeochemically reactive zone, carbon is mineralized along the flow path. This explains why groundwater-borne carbon accounted for less than 1 and 5% of total DIC and DOC fluxes, respectively. Fresh groundwater here is clearly not the source of carbon, but it is a pathway for DIC and DOC produced in the beach to reach the coastal ocean. Our results demonstrate that sandy beaches are an important source of organic and inorganic carbon to the coastal ocean. However, to better quantify SGD-derived fluxes, the biogeochemical complexity of sandy beaches must be closely examined over larger areas and greater time scales. In northern regions where snow and ice cover beaches for a few months of the year, assessments of seasonal changes in the beach flow regime and the production and release of DIC and DOC are critical to determine the impact that northern systems have on regional and global carbon budgets. This site-specific study is the first attempt to estimate volumetric and chemical groundwater fluxes to coastal Canadian waters. It highlights the importance of combining knowledge of marine chemistry and hydrogeology in formulating an adequate management approach for coastal aquifers and adjacent coastal ecosystems.
Acknowledgements
The authors thank Gwendoline Tommi-Morin and Mathilde Gauthier-Pinsonneault for their assistance in the field and for DIC analysis; Tarik Toubal for DGPS measures; Marie-Andrée Roy for producing Figure ; and Claude and Kathia Bourque for allowing us access to their beach and for the welcome they gave us. Marie Larocque (UQAM) provided valuable input throughout the study, and Laure Devine revised the English phrasing. I. Santos and two anonymous referees are gratefully acknowledged for their thoughtful and constructive comments on the original manuscript. This research was supported by a grant from the Natural Sciences and Engineering Research Council of Canada, the Canada Research Chair Program, and the Université du Québec à Rimouski (UQAR).
References
- Abarca, E., H. Karam, H. F. Hemond, and C. F. Harvey. 2013. Transient groundwater dynamics in a coastal aquifer: The effects of tides, the lunar cycle, and the beach profile. Water Resources Research 49(5): 2473–2488.
- Anschutz, P., T. Smith, A. Mouret, J. Deborde, S. Bujan, D. Poirier, and P. Lecroart. 2009. Tidal sands as biogeochemical reactors. Estuarine, Coastal and Shelf Science 84(1): 84–90.
- Bear, J., A. H. Cheng, S. Sorek, D. Ouazar, and I. Herrera. 1999. Seawater intrusion in coastal aquifers. Dordrecht, Netherlands: Kluwer Academic.
- Beck, A. J., A. A. Kellum, J. L. Luek and M. A. Cochran. 2015. Chemical flux associated with spatially and temporally variable submarine groundwater discharge, and chemical modification in the subterranean Estuary at Gloucester Point, VA (USA). Estuarine and Coasts: 38: 1–12.
- Beck, A. J., Y. Tsukamoto, A. Tovar-Sanchez, M. Huerta-Diaz, H. J. Bokuniewicz, and S. A. Sanudo-Wilhelmy. 2007. Importance of geochemical transformations in determining submarine groundwater discharge-derived trace metal and nutrient fluxes. Applied Geochemistry 22: 477–490.
- Boudreau, B. P., M. Huettel, S. Forster, R. A. Jahnke, J. J. Middelburg, P. Nielsen, F. Sansone, et al. 2001. Permeable marine sediments: Overturning an old paradigm. EOS, Transactions American Geophysical Union 82(11): 133–140.
- Boufadel, M. C. 2000. A mechanistic study of nonlinear solute transport in a groundwater-surface water system under steady state and transient hydraulic conditions. Water Resources Research 36(9): 2549–2565.
- Boyle, E., R. Collier, A. T. Dengler, J. M. Edmond, A. C. Ng, and R. F. Stallard. 1974. On the chemical mass-balance in estuaries. Geochimica et Cosmochimica Acta 38(11): 1719–1728.
- Brisebois, D. 1981. Lithostratigraphie des strates permo-carbonifères, de l’archipel des Îles-de-la-Madeleine. Ministère de l'Énergie et des Ressources Direction Générale des Energies Conventionnelles, Service de l’Exploration, Québec, Canada, Rep. DPV-796, 1981, 48 p.
- Brown, A. C., and A. McLachlan. 2002. Sandy shore ecosystems and the threats facing them: Some predictions for the year 2025. Environmental Conservation 29: 62–77.
- Burnett, W. C., P. K. Aggarwal, A. Aureli, H. Bokuniewicz, J. E. Cable, M. A. Charette, E. Kontar, et al. 2006. Quantifying submarine groundwater discharge in the coastal zone via multiple methods. Science of the Total Environment 367(2–3): 498–543.
- Burnett, W. C., G. Wattayakorn, M. Taniguchi, H. Dulaiova, P. Sojisuporn, S. Rungsupa, and T. Ishitobi. 2007. Groundwater-derived nutrient inputs to the upper Gulf of Thailand. Continental Shelf Research 27(2): 176–190.
- Cable, J. E., and J. B. Martin. 2008. In situ evaluation of nearshore marine and fresh pore water transport into Flamengo Bay, Brazil. Estuarine, Coastal and Shelf Science 76(3): 473–483.
- Caï, W. J., Y. Wang, J. Krest, and W. S. Moore. 2003. The geochemistry of dissolved inorganic carbon in a surficial groundwater aquifer in north inlet, South Carolina, and the carbon fluxes to the coastal ocean. Geochimica et Cosmochimica Acta 67(4): 631–639.
- Chaillou, G., M. Couturier, G. Tommi-Morin, and A. M. F. Rao. 2014. Total alkalinity and dissolved inorganic carbon production in groundwaters discharging through a sandy beach. Procedia Earth and Planetary Science 10: 88–99.
- Chaillou, G., M. Touchette, A. M. Rémillard, T. Buffin-Bélanger, R. St-Louis, B. Hétu, and G. Tita. 2013. Synthèse de l’état des connaissances sur les eaux souterraines aux Îles-de-la-Madeleine – impacts de l’exploration et de l’exploitation des ressources naturelles sur celles-ci. Rimouski: Université du Québec à Rimouski, 196 pp.
- Charbonnier, C., P. Anschutz, D. Poirier, S. Bujan, and P. Lecroart. 2013. Aerobic respiration in a high-energy sandy beach. Marine Chemistry 155: 10–21.
- Charette, M. A., M. E. Gonneea, P. J. Morris, P. Statham, G. Fones, H. Planquette, I. Salter, and A. N. Garabato. 2007. Radium isotopes as tracers of iron sources fueling a southern ocean phytoplankton bloom. Deep-Sea Research Part II: Topical Studies in Oceanography 54(18–20): 1989–1998.
- Cooper, H. H. 1964. A hypothesis concerning the dynamic balance of fresh water and salt water in coastal aquifer. In: Sea water in coastal aquifers. Water Supply Paper. USGS Numbered Series, ed. USGPO Vol. 1613-C, p.1–11. Washington: United States Government Printing office 1964.
- Corbett, D. R., and J. E. Cable. 2003. Seepage meters and advective transport in coastal environments. Estuaries 25: 126–132.
- Cyronak, T., I. R. Santos, D. V. Erler, and B. D. Eyre. 2013. Groundwater and porewater as major sources of alkalinity to a fringing coral reef lagoon (Muri Lagoon, Cook Islands). Biogeosciences 10(4): 2467–2480.
- Dorsett, A., J. Cherrier, J. B. Martin, and J. E. Cable. 2011. Assessing hydrologic and biogeochemical controls on pore-water dissolved inorganic carbon cycling in a subterranean estuary: A 14C and 13C mass balance approach. Marine Chemistry 127(1–4): 76–89.
- Dredge, L. A., R. J. Mott, and D. R. Grant. 1992. Quaternary stratigraphy, paleoecology, and glacial geology, Îles-de-La-Madeleine, Quebec. Canadian Journal of Earth Science 29: 1981–1986.
- Dubois, J.-M. M. 1992. Le paysage naturel et son évolution. In: Les Îles-de-la-Madeleine : un pays à découvrir, ed, Dubois, J.-M. M. and Gagnon, Journal Info Géo Graphes (Association professionnelle des géographes du Québec et Société des professeurs de géographie du Québec), no 1, p. 41–44.
- Eadie, B. J., and L. M. Jeffrey. 1973. δ13C analyses of oceanic particulate organic matter. Marine Chemistry 3: 199–209.
- Gehrels, W. R. 1994. Determining relative sea-level change from salt-marsh foraminifera and plant zones on the coast of Maine, USA. Journal of Coastal Research 10(4): 990–1009.
- Gobler, C. J., and G. E. Boneillo. 2003. Impacts of anthropogenically influenced groundwater seepage on water chemistry and phytoplankton dynamics within a coastal marine system. Marine Ecology Progress Series 255: 101–114.
- Goñi, M. A., and L. R. Gardner. 2003. Seasonal dynamics in dissolved organic carbon concentrations in a coastal watertable aquifer at the forest-marsh interface. Aquatic Geochemistry 9(3): 209–232
- Grant, D. R. 1970. Recent coastal submergence of the maritime provinces, Canada. Canadian Journal of Earth Sciences 7: 676–689.
- Hall, P. J., and R. C. Aller. 1992. Rapid, small-volume, flow injection analysis for CO2 and NH4+ in marine and freshwaters. Limnology and Oceanography 37(5): 1113–1119.
- Heiss, J. W., and H. A. Michael. 2014. Saltwater-freshwater mixing dynamics in a sandy beach aquifer over tidal, spring-neap, and seasonal cycles. Water Resources Research 50: 6747–6766.
- Hinzman, L. D., N. D. Bettez, W. R. Bolton, F. S. Chapin, M. B. Dyurgerov, C. L. Fastie, B. Griffith, et al. 2005. Evidence and implications of recent climate change in northern Alaska and other arctic regions. Climatic Change 72(3): 251–298.
- Horn, D. P. 2002. Beach groundwater dynamics. Geomorphology 48(1–3): 121–146.
- Horn, D. P. 2006. Measurements and modelling of beach groundwater flow in the swash-zone: a review. Continental Shelf Research 26(5): 622–652.
- Hwang, D.-W., Y.-W. Lee, and G. Kim. 2005. Large submarine groundwater discharge and benthic eutrophication in Bangdu Bay on volcanic Jeju Island, Korea. Limnology and Oceanography 50(5): 1393–1403.
- Jackson, N. L., K. F. Nordstrom, D. R. Smith, and W. Virginia. 2002. Geomorphic–biotic interactions on beach foreshores in estuaries. Journal of Coastal Research 424(36): 414–424.
- Johannes, R. E. 1980. The ecological significance of the submarine discharge of groundwater. Marine Ecology Progress Series 3: 365–373.
- Kim, G., J. Kim, and D.-W. Hwang. 2011. Submarine groundwater discharge from oceanic islands standing in oligotrophic oceans: Implications for global biological production and organic carbon fluxes. Limnology and Oceanography 56(2): 673–682.
- Kim, T., E. Kwon, I. Kim, S. Lee, and G. Kim. 2013. Dissolved organic matter in the subterranean estuary of a volcanic island, Jeju: Importance of dissolved organic nitrogen fluxes to the ocean. Journal of Sea Research 78: 18–24.
- Kim, T., H. Waska, E. Kwon, I. G. N. Suryaputra, and G. Kim. 2012. Production, degradation, and flux of dissolved organic matter in the subterranean estuary of a large tidal flat. Marine Chemistry 142: 1–10.
- Komada, T., J. A. Polly, and L. Johnson. 2012. Transformations of carbon in anoxic marine sediments: Implications from Δ14C and δ13C signatures. Limnology and Oceanography 57: 567–581.
- Kristensen, E., and K. Hansen. 1995. Decay of plant detritus in organic-poor marine sediment: Production rates and stoichiometry of dissolved C and N compounds. Journal of Marine Research 53: 675–702.
- Laroche, J., R. Nuzzi, R. Waters, K. Wyman, P. Falkowski, and D. Wallace. 1997. Brown tide blooms in Long Island’s coastal waters linked to interannual variability in groundwater flow. Global Change Biology 3(5): 397–410.
- Leblanc, Y. 1994. Analyse et modélisation numérique de huit puits de production sur l’île de Cap aux Meules, Îles de La Madeleine. Mémoire de maîtrise, Université du Québec à Rimouski, 95 pp.
- Lee, D. R. 1977. A devise for measuring seepage flux in lakes and estuaries. Limnology and Oceanography 22(1): 140–147.
- Lee, Y. W., and G. Kim. 2007. Linking groundwater-borne nutrients and dinoflagellate red-tide outbreaks in the southern sea of Korea using a Ra tracer. Estuarine, Coastal and Shelf Science 71(1–2): 309–317.
- Lee, Y. W., D. W. Hwang, G. Kim, W. C. Lee, and H. T. Oh. 2009. Nutrient inputs from submarine groundwater discharge (SGD) in Masan Bay, an embayment surrounded by heavily industrialized cities. Korea. Science of the Total Environment 407(9): 3181–3189.
- Lemay-Borduas, F., M. Larocque, and G. Chaillou. 2014. Coastal aquifers: A complex dynamic between groundwater and coastal water. Paper presented at the 48th CMOS Congress, Rimouski, June 1–5.
- Liu, Q., M. Dai, W. Chen, C. A. Huh, G. Wang, Q. Li, and M. A. Charette. 2011. How significant is submarine groundwater discharge and its associated dissolved inorganic carbon in a river-dominated shelf system – the northern South China Sea? Biogeosciences 8(6): 12381–12422.
- Loveless, A. M., and C. E. Oldham. 2010. Natural attenuation of nitrogen in groundwater discharging through a sandy beach. Biogeochemistry 98(1–3): 75–87.
- Madelin’Eau. 2004. Gestion des eaux souterraines aux Îles-de-la-Madeleine un défi de développement durable: Rapport final, 58 pp. www.bape.gouv.qc.ca/sections/mandats/nappes_phreatiques_iles-de-la-madeleine (accessed September, 2015).
- Madelin’Eau. 2007. Secteur sud-est de l’île de Grande Entrée – rapport hydrologique, 53 pp. www.bape.gouv.qc.ca/sections/mandats/nappes_phreatiques_iles-de-la-madeleine (accessed September, 2015).
- Madelin’Eau. 2011. Projet de réalisation d’un forage gazier vertical et conventionnel d'une profondeur de 2500 m, Municipalité des Îles de la Madeleine – rapport d'étape 1, expertise hydrogéologique, 54 pp. www.muniles.ca/images/Upload/5_forum_sur_les_hydrocarbures_rapport_madelin__eau-_etape_1 (accessed September, 2015).
- Maher, D. T., I. R. Santos, J. Gleeson, and B. D. Eyre. 2013. Groundwater-derived dissolved inorganic and organic carbon exports from a mangrove tidal creek: The missing mangrove carbon sink? Limnology and Oceanography 58(2): 475–488.
- Martin, J. B., J. E. Cable, C. Smith, M. Roy, and J. Cherrier. 2007. Magnitudes of submarine groundwater discharge from marine and terrestrial sources: Indian River lagoon, Florida. Water Resources Research 43(5): 1–13.
- Martin, J. B., K. M. Hartl, D. R. Corbett, P. W. Swarzenski, and J. E. Cable. 2003. A multi-level pore-water sampler for permeable sediments. Journal of Sedimentary Research 73(1): 128–132.
- Masselink, G., and A. Short. 1993. The effect of tide range on beach morphodynamics and morphology: A conceptual beach model. Journal of Coastal Research 9(3): 785–800.
- McCoy, C., and D. R. Corbett. 2009. Review of submarine groundwater discharge (SGD) in coastal zones of the southeast and Gulf coast regions of the United States with management implications. Journal of Environmental Management 90(1): 644–651.
- Michael, H. A., M. A. Charette, and C. F. Harvey. 2011. Patterns and variability of groundwater flow and radium activity at the coast: A case study from Waquoit Bay. Massachusetts. Marine Chemistry 127(1–4): 100–114.
- Michael, H. A., A. E. Mulligan, and C. F. Harvey. 2005. Seasonal oscillations in water exchange between aquifers and the coastal ocean. Nature 436: 1145–1148.
- Moore, W. S. 1996. Large groundwater inputs to coastal water revealed by 223Ra enrichments. Nature 380: 612–614.
- Moore, W. S. 1999. The subterranean estuary: A reaction zone of ground water and sea water. Marine Chemistry 65(1–2): 111–125.
- Moore, W. S. 2010. The effect of submarine groundwater discharge on the ocean. Annual Review of Marine Science 2(1): 59–88.
- Moore, W. S., M. Beck, T. Riedel, M. Rutgers Van Der Loeff, O. Dellwig, T. J. Shaw, B. Schnetger, and H. J. Brumsack. 2011. Radium-based pore water fluxes of silica, alkalinity, manganese, DOC, and uranium: A decade of studies in the German Wadden Sea. Geochimica and Cosmochimica Acta 75(21): 6535–6555.
- Moore, W. S., J. O. Blanton, and S. B. Joye. 2006. Estimates of flushing times, submarine groundwater discharge, and nutrient fluxes to Okatee Estuary, South Carolina. Journal of Geophysical Research 111: 1–19.
- Mulligan, A. E., and M. A. Charette. 2006. Intercomparison of submarine groundwater discharge estimates from a sandy unconfined aquifer. Journal of Hydrology 327(3–4): 411–425.
- Owens, E. H., and D. H. Frobel. 1977. Ridge and runnel systems in the Magdalen Islands, Quebec. Journal of Sedimentary Research 47(1): 191–198.
- Paerl, H. W. 1997. Coastal eutrophication and harmful algal blooms: Importance of atmospheric deposition and groundwater as “new” nitrogen and other nutrient sources. Limnology and Oceanography 42(5): 1154–1166.
- Raubenheimer, B., R. T. Guza, and S. Elgar. 1999. Tidal water table fluctuations in a sandy ocean beach. Water Resources Research 35(8): 2313–2320.
- Riedl, R. J., N. Huang, and R. Machan. 1972. The subtidal pump: A mechanism of interstitial water exchange by wave action. Marine Biology 13: 210–221.
- Robador, A., V. Brüchert, A. D. Steen, and C. Arnosti. 2010. Temperature-induced decoupling of enzymatic hydrolysis and carbon remineralization in long-term incubations of Arctic and temperate sediments. Geochimica et Cosmochimica Acta 74: 2316–2326.
- Robinson, C., L. Li, and D. A. Barry. 2007. Effect of tidal forcing on a subterranean estuary. Advances in Water Resources 30(4): 851–865.
- Roulet, N. T., P. M. Lafleur, P. J. H. Richard, T. R. Moore, E. R. Humphreys, and J. Bubier. 2007. Contemporary carbon balance and late Holocene carbon accumulation in a northern peatland. Global Change Biology 13: 397–411.
- Santos, I., W. C. Burnett, T. Dittmar, I. G. N. Suryaputra, and J. Chanton. 2009. Tidal pumping drives nutrient and dissolved organic matter dynamics in a Gulf of Mexico subterranean estuary. Geochimica et Cosmochimica Acta 73(5): 1325–1339.
- Santos, I., B. D. Eyre, and M. Huettel. 2012. The driving forces of porewater and groundwater flow in permeable coastal sediments: A review. Estuarine, Coastal and Shelf Science 98: 1–15.
- Shinn, E. A., C. D. Reich, and T. D. Hickey. 2002. Seepage meters and Bernoulli’s revenge. Estuaries 25(1): 126–132.
- Smith, A. J., and S. P. Nield. 2003. Groundwater discharge from the superficial aquifer into Cockburn Sound, Western Australia: Estimation by inshore water balance. Biogeochemistry 66: 125–144.
- Sylvestre, M. 1979. Étude par modèle mathématique des nappes souterraines de la Grosse Île et de l’île de la Grande Entrée, Îles-de-la-Madeleine. Gouvernement du Québec, Canada, Ministère des Richesses Naturelles, rapport H.G.-12, 34pp.
- Taniguchi, M., W. C. Burnett, J. E. Cable, and J. V. Turner. 2002. Investigation of submarine groundwater discharge. Hydrological Processes 16(11): 2115–2129.
- Taniguchi, M., T. Ishitobi, and J. Shimada. 2006. Dynamics of submarine groundwater discharge and freshwater–seawater interface. Journal of Geophysical Research 111: C01008.
- United Nations Educational, Scientific and Cultural Organization (UNESCO). 2004. Scientific Committee on Oceanic Research (SCOR) and Land-Ocean Interactions in the Coastal Zone (LOICZ). Submarine groundwater discharge: Management implications, measurements and effects for International Hydrological Program (IHP) and Intergovernmental Oceanographic Commission (IOC), United Nations.
- Valiela, I., J. M. Teal, S. Volkmann, C. M. Cogswell, and R. A. Harrington. 1980. On the measurement of tidal exchanges and groundwater flow in salt marshes. Limnology and Oceanography 25(1): 187–192.
- Valiela, I., J. M. Teal, S. Volkmann, D. Shafer, and E. Carpenter. 1978. Nutrient and particulate fluxes in a salt marsh ecosystem: Tidal exchanges and inputs by precipitation and groundwater. Limnology and Oceanography 23(4): 798–812.
- Weber, J. N., and P. M. J. Woodhead. 1969. Factors affecting the carbon and oxygen isotopic composition of marine carbonate sediments: Heron Island, Great Barrier Reef. Australia. Geochimica et Cosmochimica Acta 33(1): 19–38.
- Weinstein, Y., Y. Yechieli, Y. Shalem, W. Burnett, P. W. Swarzenski, and B. Herut. 2011. What is the role of fresh groundwater and recirculated seawater in conveying nutrients to the coastal ocean? Environmental Science & Technology 45: 5195–5200.
- Wurl O. and Min Sin Tsai. 2009. Analysis of Dissolved and Particulate Organic Carbon with the HTCO Technique. In: Practical Guidelines for the analysis of seawater, ed. Wurl O. Boca Raton: CRC Press. 38–40.