Abstract
Watermelon (Citrullus lanatus) is an important vegetable fruit crop, widely grown in many countries in the tropical and subtropical regions around the world. The narrow genetic base of cultivated watermelon makes it susceptible to many pests and diseases, especially viruses. However, genetic sources of resistance are available in wild relatives that could potentially be used to incorporate the resistance genes into watermelon cultivars. Ribosome-inactivating proteins (RIPs) are prevalent in many plant species and are known to have an important role in defence against bacteria, fungi, and viruses. The objective of this study was to identify RIP-like sequences in the watermelon genome and to conduct comparative measurement of RIP gene expression between the potyvirus-resistant Citrullus amarus ‘PI 244019ʹ versus the susceptible watermelon (C. lanatus) ‘Charleston Gray’ in response to infection by papaya ringspot virus (PRSV). Taking advantage of the recently available genome sequences of ‘PI 244019ʹ and ‘Charleston Gray’, in this study we mined out watermelon RIP genes and conducted comparative sequence and expression analyses of RIP-I and RIP-II between the genotypes. The RIP gene expression analysis revealed elevated RIP gene expression in resistant PI 244019 plants, but not in susceptible ‘Charleston Gray’ plants, in response to inoculation with PRSV, indicating a potential association of RIP gene expression and resistance to potyviruses in watermelon. This elevated expression of RIP genes in association with potyvirus resistance opens a new avenue to investigate the underlying mechanism of potyvirus resistance in watermelon.
Résumé
La pastèque (Citrullus lanatus) est une importante culture de légumes-fruits pratiquée extensivement dans plusieurs pays des régions tropicales et subtropicales. La base génétique étroite de la pastèque cultivée la rend sujette aux attaques de plusieurs ravageurs et maladies, spécialement des virus. Toutefois, il existe des sources de résistance chez les parents sauvages qui pourraient être incorporées aux gènes de résistance des cultivars de pastèque. On trouve des protéines inactivant le ribosome (PIR) chez plusieurs espèces de plantes et elles sont reconnues pour le rôle important qu’elles jouent dans les mécanismes de défense contre les bactéries, les champignons et les virus. Le but de cette étude était de répertorier les séquences analogues à celles des PIR dans le génome de la pastèque et de procéder à des mesures comparatives de l’expression des gènes des PIR chez le cultivar résistant au potyvirus Citrullus amarus ‘PI 244019ʹ et la pastèque réceptive (C. lanatus) ‘Charleston Gray’, en réaction à l’infection causée par le virus de la tache annulaire de la papaye (VTAP). Profitant des séquences nouvellement disponibles du génome de ‘PI 244019ʹ et de ‘Charleston Gray’, nous avons extrait des gènes des PIR de la pastèque et effectué des analyses comparatives des séquences et de l’expression des PIR-I et des PIR-II des génotypes. En réaction à l’inoculation avec le VTAP, l’analyse de l’expression des gènes des PIR a révélé une expression génique élevée des PIR chez les plants résistants ‘PI 244019ʹ, mais pas chez les plants réceptifs ‘Charleston Gray’, indiquant une possible association entre l’expression des gènes des PIR et la résistance aux potyvirus chez la pastèque. Cette expression élevée des gènes de PIR associée à la résistance au potyvirus ouvre une nouvelle voie quant à l’étude du mécanisme sous-jacent de la résistance aux potyvirus chez la pastèque.
Introduction
Watermelon (Citrullus lanatus) originated in Africa and is a member of the Cucurbitaceae family. The United States produced an average of 1.77 million tons of watermelon in 2018 and ranks among the top 10 watermelon-producing countries (FAOSTAT, http://www.fao.org/faostat/en/#home). The sweet watermelon cultivars developed in North America share a narrow genetic base (Levi et al. Citation2001; Wu et al. Citation2019a) and are susceptible to many diseases. Plants deploy numerous defence mechanisms against pathogens, including receptor mediated signaling, protein degradation, RNA silencing, and translational factors (Wu et al. Citation2019b).
Ribosome-inactivating proteins (RIPs) are toxic N-glycosidases that inhibit protein synthesis by depurinating the alpha-sarcin loop of the conserved rRNA subunit (Nielsen and Boston Citation2001). Thus, the catalytically inactive ribosomes are incapable of binding to the elongation factors, leading to termination of the translation machinery (Aitbakieva and Domashevskiy Citation2016). Based on their physical activities, RIPs are divided into three groups. The most widely distributed Type I RIPs are composed of a single polypeptide chain A with a RIP-like enzymatic activity. Some examples of Type I RIPs (RIP1) are pokeweed antiviral protein (PAP), saporin from Saponaria officinalis, and trichoanguin from Trichosanthes anguina (Nielsen and Boston Citation2001). The Type II RIPs are heteromeric proteins containing chain A (functionally same as Type I) linked to chain B by a galactose binding lectin through a disulphide bond. This class is reported to have more cytotoxic capacity, as the B chain has the ability to bind to the glycoproteins/lipids (sugar moieties) on the surface of eukaryotic cells. Some examples of cytotoxic Type II RIPs identified in plants are volkensin, viscumin, abrin and ricin (Bolognesi et al. Citation2016; Zhu et al. Citation2018). The third type of RIPs is similar to Type I RIPs, except that a Type III RIP is linked to a C-terminal domain with unknown function. The Type III RIPs have been discovered in maize (b-32_ and barley (JIP60) (Wytynck et al. Citation2017).
The expression of RIPs is known to be altered under abiotic and biotic stresses. Salinity and osmotic stress result in an up regulation of alpha-momorcharin gene in Momordica charantia (Bolognesi et al. Citation2016; Wang et al. Citation2016). Similarly, overexpression of the pokeweed PAP gene was shown to improve resistance in plants to both viruses and fungi (Lodge et al. Citation1993; Di and Tumer Citation2015). Transgenic plants expressing Phytolacca insularis antiviral protein (PIP) were found to show broad-spectrum resistance against plant viruses (Moon et al. Citation1997). A tobacco RIP protein (TRIP), isolated from Nicotiana tabacum leaves was demonstrated to have antibacterial properties against Pseudomonas solanacearum, Erwinia amylovora, Shigella asonei, Salmonella typhimurium, and Rhizobium leguminosarum (Zhu et al. Citation2018). The RIPs isolated from Cucurbita moschata showed antifungal activity against Phytophthora infestans (Barbieri et al. Citation2006).
There has been a vast amount of research documenting the antiviral activity of RIPs in plants, but the mechanism of their activity is poorly understood (Domashevskiy and Goss Citation2015). RIPs are known to act directly on the virus through depurination or indirectly by restricting virus replication and/or movement or by activating the plant defence through the hypersensitive response and cell death (Zhu et al. Citation2018). PAP, a Type I RIP, is shown to inhibit translation of both capped and uncapped viruses, including tobacco mosaic virus (TMV) and tobacco etch virus (Di and Tumer Citation2015). Beetin, a Type I RIP from Beta vulgaris, was shown to provide resistance against artichoke mottled crinkle virus (AMCV) and TMV by mediating systemic acquired resistance (Iglesias et al. Citation2005). Squash plants were shown to develop resistance against zucchini yellow mosaic virus (ZYMV) when they were pretreated exogenously with the recombinant purified PAP protein (Chen et al. Citation2002). Similarly, RIP-I extracted from leaves of Phytolacca dioica was shown to possess strong antiviral activity against tobacco necrosis virus (TNV) in Phaseolus vulgaris (Bulgari et al. Citation2020).
Watermelon production in the U.S. is threatened by a number of devastating viral diseases. The most economically damaging are aphid-transmitted potyviruses, including the watermelon strain of papaya ringspot virus (PRSV-W), watermelon mosaic virus (WMV), and ZYMV (Keinath et al. Citation2017). Previously, the USDA watermelon germplasm was screened to identify Plant Introductions (PIs) for their resistance against potyviruses: PRSV-W and ZYMV. PIs identified to have resistance to PRSV-W PI 244018, PI 244019, PI 255137, and PI 482299 (Strange et al. Citation2002). Several of these potyvirus-resistant Citrullus amarus PIs have recently been sequenced (http://cucurbitgenomics.org), which allows us to conduct a comparative gene sequence analysis with those of the virus-susceptible watermelon ‘Charleston Gray’ (Wu et al. Citation2019a). In this study, we attempted to mine out RIP genes from ‘Charleston Gray’ and ‘PI 244019ʹ from the ‘cucurbitgenomics’ database followed by comparative gene sequence analysis. We also tried to establish a link between RIP gene expression and potyvirus resistance in watermelon in response to PRSV-W infection.
Materials and methods
Identification of watermelon RIP sequences and phylogenetic analysis
The watermelon RIP sequences were identified from the cucurbit genome database (http://cucurbitgenomics.org//) and the other RIPs were retrieved from NCBI database (https://www.ncbi.nlm.nih.gov/) using BLAST searches. All pairwise alignment was done with the MegAlign Pro programme of Lasergene 16 (DNASTAR, Madison, WI) using default parameters. The multiple sequence alignment was done through ClustalW. The phylogenetic analysis was performed with the BioNJ algorithm. Geneious Prime v. 2020.2 (Biomatters Inc.) was used for RIP annotation and motif prediction. The Geneious alignment programme (Geneious Prime® 2021.2.2) was used to align the sequences of the RIP I and II of C. lanatus and C. amarus.
Plant materials and growth conditions
The USDA Plant Introduction (PI 244019) seeds were obtained from the USDA Southern Regional Plant Introduction Station (Griffin, GA, USA) and self-pollinated for two subsequent generations. The ‘Charleston Gray’ seeds were from a line increased at the USDA-ARS, U.S. Vegetable Laboratory in Charleston, SC, USA. A commercial soil mix (MetroMix 360, Sun Gro Horticulture, USA) was used to germinate the watermelon seeds. The plants were maintained in an environment-controlled greenhouse with 14 h natural sunlight at 25°C.
Virus propagation and inoculation
An isolate of the watermelon strain of PRSV collected from infected watermelon in South Carolina was propagated and maintained on susceptible melon or bottle gourd plants in a BugDome-2 insect tent (BioQuip Products, USA). Virus inoculum was prepared by macerating virus-infected leaves in ice-cold 0.1 M phosphate buffered saline solution (pH 7.0) at a ratio (1:5 w/v) using a Homex 6 tissue homogenizer (BioReba, Switzerland) or in a plastic sampling bag using a hand-held roller. The virus inoculum was maintained on ice during inoculation to preserve virus infectivity. Six plants per cultivar per treatment were used for each experiment, with a total of three independent experiments. Seedlings at the 1–2 leaf stage, lightly dusted with carborundum (320 grit, Thermo Fisher Scientific, USA), were gently rub-inoculated with a cotton swab soaked in the prepared virus inoculum. The inoculated plants were maintained in a greenhouse for symptom expression. Rating of resistance and susceptibility was based on the severity of symptom expression (mosaic or asymptomatic) on the inoculated plants 3 weeks post-inoculation (wpi). The plants were rated on a scale of 1–5 based on disease severity, where 1: no symptoms; 2: mild mosaic; 3: slightly deformed leaves with mild mosaic and chlorotic patches; 4: deformed leaves with mosaic, chlorotic and necrotic spots; and 5: basal leaves dying, leaf deformation with severe chlorosis and necrosis. The presence of the virus was confirmed with an appropriate serological test using enzyme-linked immunosorbent assay (ELISA) following the manufacturer’s instructions (Agdia) (). Leaf tissue samples were collected from PRSV-inoculated or mock-inoculated plants for ELISA testing and the mean absorbent value (OD405nm) and standard deviation (SD) were calculated.
Fig. 1 Disease response in watermelon against PRSV-W. Symptom expression on a resistant (PI 244019) and susceptible plant (‘Charleston Gray’) 3 weeks following virus inoculation. The susceptible plant shows mosaic, chlorosis, and distorted leaf symptoms in (a) detached leaves; results from an (b) showed absorbance associated with the virus titre using a PRSV-W ELISA kit (Agdia).
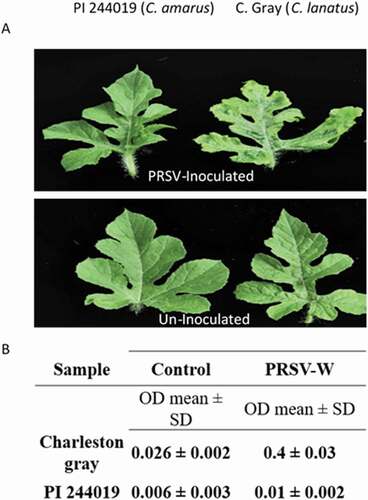
RNA extraction and real-time PCR
To evaluate the level of target RIP-I and RIP-II gene transcript expression in watermelon plants upon PRSV-W infection, a quantitative real-time reverse transcription polymerase chain reaction (qRT-PCR) was used. Total plant RNA was isolated from freshly collected or liquid nitrogen flash-frozen leaf tissues. Leaf tissues were lysed first using TRIzol reagent [(Invitrogen, USA). Total RNA purification and in-column DNase digestion were conducted using the QIAGEN RNeasy Mini Kit following the manufacturer’s instructions (Qiagen, Germany). RT-PCR was performed on total RNA preparation using SuperScript® III Reverse Transcriptase (Invitrogen, USA) following the manufacturer’s instructions. To quantify the expression of the target gene transcript expression level, qRT-PCR was conducted using appropriate primer combinations () in a reaction volume of 25 μL with a Brilliant II SYBR green Q RT PCR master mix (Applied Biosystems, USA). The reaction was run on an Applied Biosystems Real-Time PCR System with a thermal cycling profile of 95°C for 60s followed by 40 cycles of 95°C for 10s and 60°C for 30s. The watermelon actin gene was used as an internal control. This experiment was repeated twice to validate the results. The primers employed for examining the relative expression of the selected genes are listed in (). The primers were designed based on the sequence homology between the RIPs, taking C1CG08G004130 as a reference sequence for RIP I and C1CG09G009010 for RIP II.
Table 1. List of primers used for the study of ribosome-inactivating protein (RIP) genes in watermelon.
Statistical analysis
Statistical analysis using two-way ANOVA was followed by Fisher’s LSD (α = 0.05) test to analyse the level of significance between mock controls and the treatments.
Results
Identification and characterization of candidate RIP-like sequences in watermelon ‘Charleston Gray’ genome and analysis of their phylogenetic relationship
Using the cucurbit genomics database (Zheng et al. Citation2019), we identified the RIP gene sequences in the watermelon genome and characterized their expression in response to virus infection, specifically by PRSV-W. We identified eight putative RIP-like genes in the ‘Charleston Gray’ genome.
To elucidate the functional domains of the eight RIP-like sequences, sequence analysis and annotation was carried out with the Geneious prime software (). Based on the secondary structure and domain analysis, these eight-RIP-like proteins were assigned to three distinct RIP type groups. Four genes encoded Type I RIPs: including ClCG08G004120-(265 AA); ClCG08G004130-(307 AA); ClCG11G001040-(256 AA); and ClCG08G004150-(250 AA), which are composed of a RIP domain and a signal peptide (). One exception was ClCG08G004150, which lacked a signal peptide. Two genes encoded Type II RIPs: ClCG09G009010-(537 AA) and ClCG08G004200-(546 AA), which consisted of a signal peptide, RIP domain, lectin domain and a C terminal domain of unknown function (). Two other genes were considered as non-RIP: ClCG08G004160-(287 AA) and ClCG05G009670-(292 AA), which consisted of only a signal peptide, a lectin domain and a C-terminal domain of unknown function (). This latter group of non-RIP lectin proteins are like SNAII and SNAIV of Sambucus nigra (elderberry), which are likely less toxic than type I and type II RIP proteins (Chen et al. Citation2002). The toxicity of RIPs is based on substrate-specific enzymatic/catalytic activity (Barbieri et al. Citation2004; Vandenbussche et al. Citation2004).
Table 2. The types of RIP-like sequences, their associated genes and chromosomal locations as identified in the genome of the watermelon cv. ‘Charleston Gray’.
Fig. 2 Alignment of RIP-like sequences identified in the genome of the watermelon cv. ‘Charleston Gray’ and their predicted secondary structures (pink and the blue regions). The top bar indicates the consensus sequence. All the disagreements with the consensus sequence are indicated with colours, with A: red; C: blue; G: yellow and T: green. The grey regions indicate the sequences in agreement. The red bar indicates the signal peptide, and the green bars indicate RIP domain, lectin domain and unknown C-terminal domain. Eight-RIP-like proteins were identified in the genome of ‘Charleston Gray’ and were assigned to three distinct RIP type groups: RIP-I (C1CG11G001040, C1CG08G004120, C1CG08G004130, C1CG08G004150); RIP-II (C1CG09G009010, C1CG08G004200) and non RIP (C1CG08G004160, C1CG05G009670).
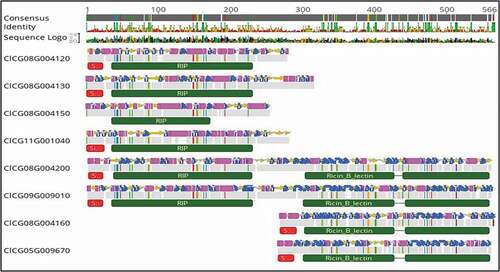
To evaluate potential evolutionary relationships of the identified watermelon RIP-I, RIP-II and the non-RIPs with other well-known RIPs, we conducted a phylogenic analysis with the eight watermelon RIP proteins and selected representative RIPs (). The four RIP-I proteins from watermelon, including ClCG08G004120, ClCG08G004130, ClCG11G001040 and ClCG08G004150, were clustered with a representative RIP-I identified from the cucurbit Trichosanthes anguina (Snake gourd) Trichoanguin.pro (GenBank Accession No. P56626.2). The two watermelon RIP-II proteins, ClCG09G009010 and ClCG08G004200, were grouped with the representative RIP-II, Nigrin b (Sambucus nigra) GenBank Accession No. U41299.1. As expected, the two watermelon non-RIP lectin domain RIPs, including ClCG08G004160-(287 AA) and ClCG05G009670-(292 AA), were on their own as a separate branch ().
Fig. 3 Phylogenetic analysis of watermelon RIPs in relationship with some selected representative RIPs from other plant species. A phylogenetic tree was constructed using the neighbour joining (NJ) algorithm based on the amino acid sequence of RIP-like sequences identified from the genome of the watermelon ‘Charleston Gray’ (http://cucurbitgenomics.org/). The watermelon RIP genes are represented by their respective sequence IDs. Amino acid sequences of the selected representative RIP-I and RIP-II were derived from GenBank (https://www.ncbi.nlm.nih.gov/genbank/), Trichosanthes anguina (snake gourd) RIP-I Trichoanguin (GenBank Accession No. P56626.2) and RIP-II Nigrin b (Sambucus nigra; GenBank Accession No. U41299.1).
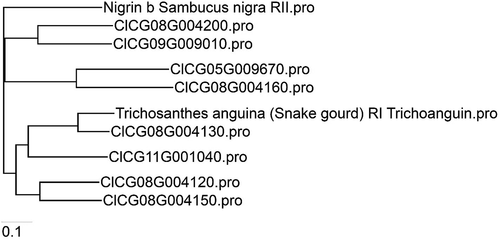
Pairwise alignment of the four RIP-I proteins revealed 60–65% amino acid (aa) sequence identities among them, whereas two RIP-II proteins shared 65% aa sequence identity between them (). BLAST searches to the NCBI database using watermelon ‘Charleston Gray’ RIP-I proteins showed aa sequence identity in the range of 47% to 74% with the other known RIP-I proteins, including Trichosanthin from Trichosanthes kirilowi and Trichoanguin from Trichosanthes anguina (snake gourd). Similarly, watermelon RIP-II proteins shared around 34% sequence identity with other known RIP-II proteins, including Nigrin b from Sambucus nigra and Ricin from Ricinus communis. Similarity between watermelon RIPs and their representative RIP-I, Trichoanguin from Trichosanthes anguina (Snake gourd) or RIP-II, ricin (Ricinus communis), were conducted by pairwise comparisons of amino acid sequences between the individual watermelon RIP and its representative member (). The watermelon C1CG08G004130 (Type I RIP) showed the highest aa sequence identity of 72.5% (similarity of 84.2%) with the well-known Type I RIP representative, trichoanguin. Similarly, the watermelon C1CG09G009010 (Type II RIP) showed a higher aa sequence identity of 34.9% (similarity of 53.2%) with the representative ricin (a Type II RIP) ().
Table 3. Pairwise sequence analysis indicating the similarity in the amino acid sequences of the RIP-I and RIP-II proteins.
Table 4. Pairwise analysis of amino acid sequence identity and similarity between the watermelon RIPs with their representative RIP-I, Trichoanguin (Trichosanthes anguina) or RIP-II, Ricin (Ricinus communis).
Identification of candidate RIP-like sequences in the genome of Citrullus amarus, a wild relative of watermelon
Citrullus amarus is a wild relative of cultivated watermelon (C. lanatus) and is a source of disease resistance genes, including resistance to viral diseases (Branham et al. Citation2020). Taking advantage of the availability of the genome sequence of C. amarus in the cucurbit genomics database ‘CuGenDB’ (http://cucurbitgenomics.org/) and using watermelon ‘Charleston Gray’ RIP-I and RIP-II genes, we were able to identify the orthologous RIP-I and RIP-II in C. amarus (PI 244019), which has been shown to have resistance to potyviruses, including PRSV-W (Strange et al. Citation2002). In a pairwise aa sequence comparison, the RIP-I gene of C. amarus (PI 244019) is nearly identical (99.2%) to the 924 bp watermelon RIP-I gene (C1CG08G004130), with only seven single nucleotide polymorphisms (SNPs) differentiating them (). Similarly, the RIP-II gene of C. amarus shared 93.5% nucleotide sequence identity, with the presence of one indel of 96-bp deletion, in comparison with the 1614 bp watermelon ‘Charleston Gray’ RIP-II sequence (C1CG09G009010).
Fig. 4 Identification of RIP-I and RIP-II in Citrullus amarus. A. Gene orthologues of RIP-I gene (C1CG08G004130) and RIP-II (C1CG09G009010) from the watermelon cv. ‘Charleston Gray’ were identified in C. amarus (PI 244019) in the cucurbit genomics database ‘CuGenDB’ (http://cucurbitgenomics.org/) and were aligned with Geneious Prime software with the Geneious alignment tool to compare the similarity between the genes visually. B. Pairwise analysis of nucleotide sequence identity between C. lanatus and C. amarus RIPs.
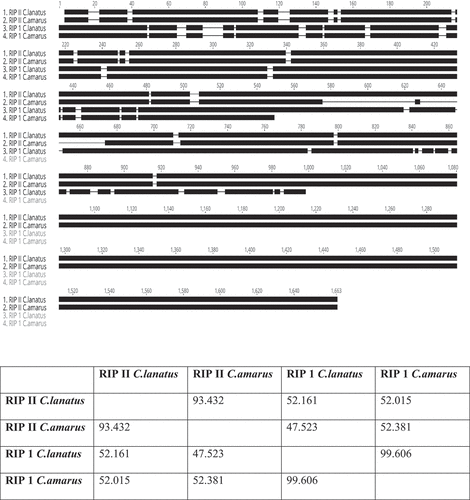
Assessing the tissue-specific expression of RIP gene transcripts in watermelon using quantitative RT-PCR
To determine the expression profiles of RIPs in various watermelon tissue types (including shoot tip, stem, mature leaf, young leaf), fresh tissues were harvested from young seedlings (4 weeks post germination) of ‘Charleston Gray’. Quantitative RT-PCR was performed using total plant RNA extracted from these tissues. The RNA extracted from the germinating seed was used as a baseline reference to estimate the levels of RIP gene expression in different tissue types. Both types of RIPs (RIP-I and RIP-II) were present in detectable levels in all tissues examined. Expression of RIP-I was higher than RIP-II in all tissue types. The highest level of expression was found in samples from mature leaves, followed by shoot tips, young leaves and stem. RIP-II, on the other hand, had very weak levels of expression in all tissues, except in the mature leaves ().
Fig. 5 Comparative evaluation of tissue-specific expression of RIP-I and RIP-II in watermelon. Quantitative real time RT-PCR was used to compare the relative expression of RIP-I and RIP-II gene transcripts in different tissues in the watermelon ‘Charleston Gray’. The levels of the RIP-I and RIP-II mRNA was normalized with actin in each sample. The standard deviation (SD) was derived from three biological replicates. Statistical analysis using two-way ANOVA was followed by Fisher’s LSD (α = 0.05) test to analyse the level of significance between different tissues and the control germinating seed. Significantly different treatments are designated with asterisks; ‘***’, adjusted p-value ≤ 0.0001; ‘****’, adjusted p-value < 0.00001; and ‘ns’, not significant.
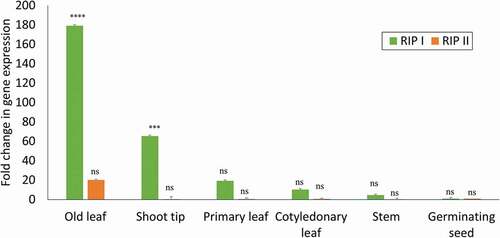
Comparative analysis of expression of RIPs genes in watermelon cv ‘Charleston Gray’ and C. amarus plants in response to infection by PRSV-W
To evaluate differential gene expression of RIP-I and RIP-II genes in watermelon plants for their responses upon infection by PRSV-W, we selected two types of watermelon plants for inoculation, the PRSV-susceptible cv. ‘Charleston Gray’ and the PRSV-resistant germplasm, C. amarus (PI 244019). After mechanical inoculation, leaves of the test plants were examined weekly for symptom development, with a final rating at 21 dpi. Disease symptoms such as plant stunting, yellow mosaic and leaf deformation were observed on the susceptible watermelon ‘Charleston Gray’ plants, whereas the inoculated PRSV-resistant C. amarus ‘PI 244019ʹ plants showed normal plant development without visible disease symptoms. To confirm virus infection, samples were taken from a systemic leaf of both resistant and susceptible plants to test for the presence of the virus using PRSV-specific ELISA. Virus titres as measured in absorbance values were much higher in the susceptible watermelon ‘Charleston Gray’ plants compared with the PRSV-resistant C. amarus ‘PI 244019ʹ plants ().
To study the steady state expression of RIPs transcripts during the disease development process in plants resistant or susceptible to PRSV-W infection, samples from inoculated and mock-inoculated control leaves were evaluated at the early infection stage (1 dpi and 3 dpi) or at a late-infection stage (8 dpi). Based on the genome search and expression analysis of RIP genes, two watermelon RIPs genes representing RIP-I (C1CG08G004130) and RIP-II (C1CG09G009010) were chosen to examine their expression in association with resistance and susceptibility to PRSV-W infection. Quantitative RT-PCR was carried out on extracted RNA samples with primers specific to watermelon RIP I and RIP II. In order to be able to make comparative quantitative analysis, Ct values measuring changes in the expression of RIP I and RIP II were normalized to that of the housekeeping gene actin at each time measurement point. It is worth noting that at 1 dpi, there was no change in the fold expression of either RIP-I and RIP-II in both the resistant and the susceptible plants in comparison with the mock control (). For the virus-susceptible watermelon plants, although there was a small increase in the expression of RIP-I and RIP-II in comparison with the mock controls at the early stage (3 dpi), there was no difference in the expression of RIP genes at 8 dpi. In contrast, in the virus-resistant C. amarus plants, a significant 4-fold increase in the expression of the RIP-I gene was observed at 3 dpi and then a 6-fold increase in the expression of the RIP-II gene at 8 dpi in the resistant-inoculated plants, in comparison with the mock-inoculated plants ().
Fig. 6 Comparison of differential plant responses in watermelon plants resistant or susceptible to PRSV-W infection. Real-time quantitative PCR expression analysis of RIP-I and RIP-II genes in the PRSV-W-inoculated-resistant Citrullus amarus ‘PI 244019ʹ and the susceptible watermelon ‘Charleston gray’ at 1, 3- and 8-days post inoculation (dpi). The data were normalized against the actin gene. The standard deviation (SD) was derived from three biological replicates. Statistical analysis using two-way ANOVA was followed by Fisher’s LSD (α = 0.05) test to analyse the level of significance between the mock-control and the different treatments. Significantly different treatments are designated with asterisks; ‘*’, adjusted p-value ≤ 0.01; ‘**’, adjusted p-value ≤ 0.001; and ‘ns’, not significant.
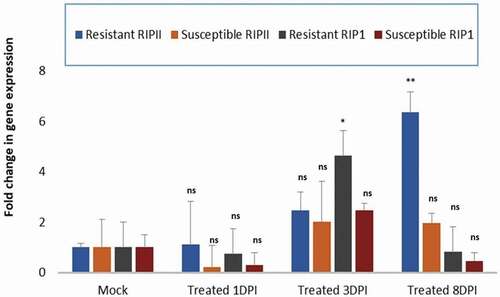
Discussion
In this study, through bioinformatic analysis, we identified eight RIP-like sequences in the watermelon genome, which could be classified into three types: RIP-I, RIP-II and a non-RIP lectin domain protein. Through orthologous implication, we also identified the same RIP-like sequences in a published genome, available in the Cucurbit Genomics Database (CuGenDB, http://cucurbitgenomics.org/), of C. amarus, a wild relative of watermelon that has been shown to possess disease resistance genes against multiple pathogens, including PRSV-W (Strange et al. Citation2002).
RIPs are well known for their antibacterial, antifungal and anticancer activities (Walsh et al. Citation2013; Zeng et al. Citation2015; Zhu et al. Citation2018). We were interested in evaluating the expression of RIPs in various watermelon tissues and to determine whether their expression could be enhanced under biotic stress conditions. The expression of RIP genes in watermelon was found to be developmentally regulated and most abundant in the mature leaves. RIPs are known to be differentially regulated; PAP, a well-known RIP of pokeweed, is expressed in nine different isoforms in young and mature leaves and seeds, roots, and callus culture (Domashevskiy and Goss Citation2015).
We also found that RIPs, both I and II from C. amarus (PI244019), a wild relative of the cultivated sweet dessert watermelon, were induced upon PRSV-W infection, even though the cultivated watermelon ‘Charleston Gray’ had little change. In the PRSV-resistant C. amarus (PI244019), the level of expression of RIP-II was found to be six-fold higher than in mock-inoculated plants at 8 dpi (late response). In contrast, RIP-I of the resistant C. amarus ‘PI 244019ʹ showed a four-fold higher expression than the mock-inoculated control at 3 dpi (early response). These results suggest that the mode of antiviral action for the two types of RIPs in watermelon could be temporally different. Unlike the Type I RIPs, Type II RIPs are reported to be more cytotoxic because they possess a lectin domain that has the capability to bind to the cell surface and enters the cell through endocytosis (Bolognesi et al. Citation2016). Previous studies have shown the relationship between RIP expression and antiviral activity. Transgenic pokeweed plants (P. americana) expressing the RIP PAP showed resistance to potato virus X (PVX), potato virus Y (PVY) and cucumber mosaic virus (CMV) in tobacco, potato and N. benthamiana (Lodge et al. Citation1993). Similarly, transgenic potato plants expressing RIP from P. insularis showed protection against PVX and PVY (Moon et al. Citation1997). In addition, transgenic Brassica napus expressing PAP showed resistance against turnip mosaic virus (TuMV) (Zhang et al. Citation1999). Zhu et al. (Citation2013) demonstrated that tobacco plants pretreated with a recombinant α-momorcharin (α-MMC), a RIP-I, inhibited the systemic spread of a wide range of phytopathogenic viruses, including chilli veinal mottle virus (ChiVMV), CMV and TMV. Pre-treated plants showed fewer symptoms, accumulated less reactive oxygen species and induced salicylic acid responsive defence associated PR proteins. In another example, transgenic tobacco (Nicotiana tabacum) plants expressing the Type II RIP from Sambucus nigra (SNA-1) also showed antiviral activity against TMV without the expression of PR genes (Chen et al. Citation2002). Thus, both Type I and II RIPs could exhibit antiviral activities but with different modes of action.
The antiviral activity of RIPs may also be dosage-dependent. Recombinant PAP-1 from pokeweed and recombinant PIP 2 from Phytolacca insularis acted in a dose-dependent manner against ZYMV and TMV in squash and tobacco (Song et al. Citation2000; Sipahioglu et al. Citation2017). This could imply that the resistance response in ‘PI 244019ʹ is a consequence of higher expression of RIPs, leading to increased protein accumulation compared with the small increase in gene expression of the RIPs in the susceptible cultivar.
PRSV belongs to the family Potyvirideae. Potyviruses have been shown to use eIF4E/eIFiso4E, cap binding protein/eukaryotic elongation factor to complete their infection cycle. Our previous study (Ling et al. Citation2009) showed that a SNP mutation in the eIF4E gene, a critical site for cap recognition and binding of the virus, was responsible in conferring resistance to ZYMV in PI 595203 (Citrullus mucosospermus), an Egusi-type watermelon of African origin. Whether the presence of same mutation in eIF4E of C amarus ‘PI 244019ʹ is involved in conferring resistance to PRSV is the goal of our future investigation. The enhanced level of expression of RIP genes in response to PRSV infection opens the scope to investigate global gene expression through RNA-seq during PRSV-W infection. This will help to identify genes and pathways involved in mediating resistance to potyviruses in watermelon and other crops.
Acknowledgements
We thank Laura Massey and Andrea Gilliard for their excellent technical assistance and Drs. Omotola Olaniyi and Sharon Andreason for the critical review of the manuscript.
Disclosure statement
No potential conflict of interest was reported by the author(s).
Additional information
Funding
References
- Aitbakieva VR, Domashevskiy AV. 2016. Insights into the molecular antiviral mechanism of pokeweed protein from Phytolacca Americana. Biochem Pharmacol. 5:210. doi:https://doi.org/10.4172/2167-0501.1000210.
- Barbieri L, Ciani M, Girbés T, Liu WY, Van Damme EJ, Peumans WJ, Stirpe F. 2004. Enzymatic activity of toxic and non-toxic type 2 ribosome-inactivating proteins. FEBS Lett. 563(1–3):219–222. doi:https://doi.org/10.1016/S0014-5793(04)00286-8.
- Barbieri L, Polito L, Bolognesi B, Ciani M, Pelosi E, Farini V, Jha AK, Sharma N, Jorge M, Chambery A, et al. 2006. Ribosome-inactivating proteins in edible plants and purification and characterization of a new ribosome-inactivating protein from Cucurbita moschata. Biochim Biophys Acta. 1760:783–792. doi:https://doi.org/10.1016/j.bbagen.2006.01.002.
- Bolognesi A, Bortolotti M, Maiello S, Battelli MG, Polito L. 2016. Ribosome-inactivating proteins from plants: a historical overview. Molecules. 21:1627. doi:https://doi.org/10.3390/molecules21121627.
- Branham SE, Wechter WP, Ling K, Chanda B, Massey L, Zhao G, Guner N, Bello M, Kabelka E, Fei Z, et al. 2020. QTL mapping of resistance to Fusarium oxysporum f. sp. niveum race 2 and Papaya ringspot virus in Citrullus amarus. Theor Appl Genet. 133:677–687. doi:https://doi.org/10.1007/s00122-019-03500-3.
- Bulgari D, Landi N, Ragucci S, Faoro F, Di Maro A. 2020. Antiviral activity of PD-L1 and PD-L4, type 1 ribosome inactivating proteins from leaves of Phytolacca dioica L. in the pathosystem Phaseolus vulgaris–Tobacco Necrosis Virus (TNV). Toxins. 12(8):524. doi:https://doi.org/10.3390/toxins12080524.
- Chen Y, Peumans WJ, Van Damme EJ. 2002. The Sambucus nigra type-2 ribosome-inactivating protein SNA-I’ exhibits in planta antiviral activity in transgenic tobacco. FEBS Lett. 516:27–30. doi:https://doi.org/10.1016/S0014-5793(02)02455-9.
- Di R, Tumer NE. 2015. Pokeweed antiviral protein: its cytotoxicity mechanism and applications in plant disease resistance. Toxins. 7:755–772. doi:https://doi.org/10.3390/toxins7030755.
- Domashevskiy AV, Goss DJ. 2015. Pokeweed antiviral protein, a ribosome inactivating protein: activity inhibition and prospects. Toxins. 7:274–298. doi:https://doi.org/10.3390/toxins7020274.
- Iglesias R, Perez Y, de Torre C, Ferreras JM, Antolin P, Jimenez P, Rojo MA, Mendez E, Girbes T. 2005. Molecular characterization and systemic induction of single-chain ribosome-inactivating proteins (RIPs) in sugar beet (Beta vulgaris) leaves. J Exp Bot. 56:1675–1684. doi:https://doi.org/10.1093/jxb/eri164.
- Keinath AP, Wintermantel WM, Zitter TA, editors. 2017. Compendium of cucurbit diseases and pests. 2nd ed. St. Paul (MN): APS Press.
- Levi A, Thomas CE, Wehner TC, Zhang X. 2001. Low genetic diversity indicates the need to broaden the genetic base of cultivated watermelon. HortScience. 36:1096–1101. doi:https://doi.org/10.21273/HORTSCI.36.6.1096.
- Ling KS, Harris KR, Meyer JD, Levi A, Guner N, Wehner TC, Bendahmane A, Havey MJ. 2009. Non-synonymous single nucleotide polymorphisms in the watermelon eIF4E gene are closely associated with resistance to Zucchini yellow mosaic virus. Theor Appl Genet. 120:191–200. doi:https://doi.org/10.1007/s00122-009-1169-0.
- Lodge JK, Kaniewski WK, Tumer NE. 1993. Broad-spectrum virus resistance in transgenic plants expressing pokeweed antiviral protein. Proc Natl Acad Sci USA. 90:7089–7093. doi:https://doi.org/10.1073/pnas.90.15.7089.
- Moon YH, Song SK, Choi KW, Lee JS. 1997. Expression of a cDNA encoding Phytolacca insularis antiviral protein confers virus resistance of transgenic potato plants. Mol Cells. 7:807–815.
- Nielsen K, Boston RS. 2001. Ribosome-inactivating proteins: a plant perspective. Annu Rev Plant Biol. 52:785–816. doi:https://doi.org/10.1146/annurev.arplant.52.1.785.
- Sipahioglu H, Kaya I, Usta M, Unal M, Özcan D, Özer M, Güller A, Pallás V. 2017. Pokeweed (Phytolacca americana L) antiviral protein inhibits Zucchini yellow mosaic virus infection in a dose-dependent manner in squash plants. Turk J Agric For. 41:256–262. doi:https://doi.org/10.3906/tar-1612-30.
- Song SK, Choi Y, Moon YH, Kim SG, Choi YD, Lee JS. 2000. Systemic induction of a Phytolacca insularis antiviral protein gene by mechanical wounding jasmonic acid and abscisic acid. Plant Mol Biol. 43:439–450. doi:https://doi.org/10.1023/A:1006444322626.
- Strange EB, Guner N, Pesic-vanesbroeck Z, Wehner TC. 2002. Screening the watermelon germplasm collection for resistance to Papaya Ringspot Virus Type-W. Crop Sci. 42:1324–1330. doi:https://doi.org/10.2135/cropsci2002.1324.
- Vandenbussche F, Desmyter S, Ciani M, Proost P, Peumans WJ, Van Damme EJ. 2004. Analysis of the in planta antiviral activity of elderberry ribosome-inactivating proteins. Eur J Biochem. Apr 271(8):1508–1515. doi:https://doi.org/10.1111/j.1432-1033.2004.04059.x.
- Walsh MJ, Dodd JE, Hautbergue GM. 2013. Ribosome-inactivating proteins: potent poisons and molecular tools. Virulence. 4(8):774–784. doi:https://doi.org/10.4161/viru.26399.
- Wang S, Zhang H, Zheng Y, Li Z, Xiang F, Ding Y, Xiang J. 2016. Environmental factors and phytohormones enhancing expression of α-momorcharin gene in Momordica charantia. Biologia. 71:155–160. doi:https://doi.org/10.1515/biolog-2016-0018.
- Wu S, Wang X, Reddy U, Sun H, Bao K, Gao L, Mao L, Patel T, Ortiz C, Abburi VL, et al. 2019a. Genome of ‘Charleston Gray’ the principal American watermelon cultivar and genetic characterization of 1365 accessions in the US National Plant Germplasm System watermelon collection. Plant Biotechnol J. 17:2246–2258. doi:https://doi.org/10.1111/pbi.13136.
- Wu X, Valli A, García JA, Zhou X, Cheng X. 2019b. The tug-of-war between plants and viruses: great progress and many remaining questions. Viruses. 11(3):203. doi:https://doi.org/10.3390/v11030203.
- Wytynck P, Rouge P, Van Damme EJM. 2017. Genome-wide screening of Oryza sativa ssp japonica and indica reveals a complex family of proteins with ribosome-inactivating protein domains. Phytochemistry. 143:87–97. doi:https://doi.org/10.1016/j.phytochem.2017.07.009.
- Zeng M, Zheng M, Lu D, Wang J, Jiang W, Sha O. 2015. Anti-tumor activities and apoptotic mechanism of ribosome-inactivating proteins. Chin J Cancer. 34(8):325–334. doi:https://doi.org/10.1186/s40880-015-0030-x.
- Zhang H, Tian Y, Zhou Y, Dang B, Lan Y, Song G, Wang L, Liu G, Zhang L, and Chen Z. 1999. Introduction of pokeweed antiviral protein cDNA into Brassica napus and acquisition of transgenic plants resistant to viruses. Chin Sci Bull. 44:701–704. https://doi.org/https://doi.org/10.1007/BF02909706.
- Zheng Y, Wu S, Bai Y, Sun H, Jiao C, Guo S, Zhao K, Blanca J, Zhang Z, Huang S, et al. 2019. Cucurbit Genomics Database (CuGenDB): a central portal for comparative and functional genomics of cucurbit crops. Nucleic Acids Res. 47:D1128–D1136. doi:https://doi.org/10.1093/nar/gky944.
- Zhu F, Zhang P, Meng YF, Xu F, Zhang DW, Cheng J, Lin HH, Xi DH. 2013. Alpha-momorcharin a RIP produced by bitter melon enhances defense response in tobacco plants against diverse plant viruses and shows antifungal activity in vitro. Planta. 237:77–88. doi:https://doi.org/10.1007/s00425-012-1746-3.
- Zhu F, Zhou YK, Ji ZL, Chen XR. 2018. The plant ribosome-inactivating proteins play important roles in defense against pathogens and insect pest attacks. Front Plant Sci. 9:146. doi:https://doi.org/10.3389/fpls.2018.00146.