Abstract
Recent advances in genomics and associated disciplines like bioinformatics have made it possible to develop genomic resources, such as large-scale sequence data for any crop species. While these datasets have been proven very useful for the understanding of genome architecture and dynamics as well as facilitating the discovery of genes, an obligation for, and challenge to the scientific community is to translate genome information to develop products, i.e. superior lines for trait(s) of interest. We call this approach, “translational genomics in agriculture” (TGA). TGA is currently in practice for cereal crops, such as maize (Zea mays) and rice (Oryza sativa), mainly in developed countries and by the private sector; progress has been slow for legume crops. Grown globally on 62.8 million ha with a production of 53.2 million tons and a value of nearly 24.2 billion dollars, the majority of these legumes have low crop productivity (<1 ton/ hectare) and are in the developing countries of sub Saharan Africa, Asia and South America. Interestingly, the last five years have seen enormous progress in genomics for these legume crops. Therefore, it is time to implement TGA in legume crops in order to enhance crop productivity and to ensure food security in developing countries. Prospects, as well as some success stories of TGA, in addition to advances in genomics, trait mapping and gene expression analysis are discussed for five leading legume crops, chickpea (Cicer arietinum), common bean (Phaseolus vulgaris), groundnut (Arachis hypogaea), pigeonpea (Cajanus cajan) and soybean (Glycine max). Some efforts have also been outlined to initiate/ accelerate TGA in three additional legume crops namely faba bean (Vicia faba), lentil (Lens culinaris) and pea (Pisum sativum).
I. INTRODUCTION
The United Nations Department of Economic and Social Welfare in its demographic estimates and projections, predicts that the global human population will reach 9 billion by 2050. To feed this growing population, agricultural production must be 60% higher than current production. This will be a challenge in the face of climate changes such as higher temperatures, shifting seasons, more frequent and extreme weather events, flooding, and drought. The dramatic increases in food production and land productivity during 1960–1990s, due to the “green revolution”, has reached a plateau (www.fao.org). Scientists have been striving to use conventional breeding approaches to enhance production and productivity of crop plants, thus emphasizing the need for new approaches to meet food and nutritional security for the future (Tester and Langridge, Citation2010).
With the advent of molecular markers and recent advances in genomics research, it has been possible to utilize genomics for enhancing the precision and efficiency of crop breeding. This process has been referred as “genomics-assisted breeding” (GAB; Varshney et al., 2005). GAB includes deployment of structural, functional as well as comparative genomics to identify molecular markers including functional markers, candidate genes, and predictive markers for breeding. Several kinds of molecular markers such as simple sequence repeats (SSR) and single nucleotide polymorphism (SNP) markers have been used for trait dissection and for enhancing precision in selection in plant breeding programs (Xu et al., Citation2012). Further, advanced research institutes (ARIs) and private sector organisations, mainly in developed countries, have made significant progress towards deploying GAB for enhancing yield under abiotic and biotic stresses (Varshney et al., Citation2013a). For instance, downy mildew resistant pearl millet (HHB67-2 improved; http://www.icrisat.org/impacts/impact-stories/icrisat-is-hhb67.pdf), submergence tolerant rice (Swarna; Neeraja et al., 2007), bacterial blight resistant rice (Triguna; Sundaram et al., 2009) and quality protein maize (VivekQPM9; Gupta et al., Citation2009; 2013) are some products of marker-assisted selection (MAS) or GAB in public sector. Improved lines have been developed, in fact, for many different crop plants (see Gupta et al., Citation2009; Kulwal et al., Citation2011).
Understanding the structure, organization and dynamics of genomes in plant species can provide insights into how genes have been adapted by natural and artificial selection to respond to environmental constraints and the potential for their manipulation for crop improvement. In pursuit of these goals, several plant genomes have been sequenced. Because of the high cost of Sanger sequencing and limited expertise in the plant science community, only a few plant species were initially selected as ‘models’ and targeted for both sequencing and the development of functional genomics resources. One purpose of generating genome sequences for model plant species was to better understand genome architecture and to create a ‘parts list’ for a plant. There were high hopes that much of this information could be transferred and utilized in crop species, generally referred as ‘comparative genomics’ (Ahn and Tanksley, 1993; Gale and Devos, Citation1998). The idea was prevalent that ‘model to crop’ translation would be a powerful tool for the application of genetics and genomics in crop plants; occasionally this approach was also referred to as ‘translational genomics’ (Elma et al., Citation2007). In part the idea that ‘model to crop translation’ could solve the problems of agriculture was driven by an idea, prevalent in the 1990s, that transgenic solutions to the constrains that face crop species would be simple to find without the need for extensive genetic and genomic analyses of these target crop species. This was a seductive simplification with obvious attractions for lowered research costs.
As a result of emphasis on model plants, large-scale genome sequence information was first generated for Arabidopsis thaliana (The Arabidopsis Genome Initiative, 2000), followed by rice (Oryza sativa) both as model as well as crop (International Rice Genome Sequencing Project, 2005), poplar (Populus trichophora; Tuskan et al., Citation2006), grape (Vitis vinifera; Jallion et al., 2007), maize (Zea mays; Schnable et al., 2009) and later the first legume genome sequence, of soybean (Schmutz et al., Citation2010). Recent advances in next generation sequencing (NGS) technologies, with increased throughput and reduced sequencing costs, dramatically changed the sequencing scenarios for plant genomes (Deschamps and Campbell, Citation2010; Thudi et al., Citation2012; Varshney et al., Citation2009a). To date, the genome sequences for more than 55 plant species have been produced (Michael and Jackson, Citation2013) and many more are being sequenced (http://www.onekp.com/). The genome sequence information available has enabled the identification and development of genome-wide markers and a range of marker genotyping platforms including KASPar assays, Sequenom, BeadXpress System, GoldenGate assays, Infinium assays, genotyping-by-sequencing, etc. In addition, several organizations have started to re-sequence large-scale germplasm collections (Branca et al., Citation2011; Lam et al., Citation2010; Varshney et al., Citation2013a; Xu et al., Citation2011). It is important to mention that advances have not been limited to genome sequences; data at the transcriptome and epigenome level have also contributed greatly. NGS technology has become routine now for transcriptome sequence (Hiremath et al., Citation2011), gene expression analysis (Hoen et al., Citation2008; Wang et al., Citation2012), and epigenetics (Jackson et al., Citation2011), not only for model plants but in crop species too.
It is evident from the above that we are living in sequence-rich or genome information era. For the majority of crop species, this transition occured during the past 10 years. Before this transition, the breeding community could not initiate genomics-assisted breeding due to lack of genome information. Moreover, it is still difficult for the breeding community today to navigate and interpret sequence data in order to devise strategies for crop improvement. In our opinion, the plant science community has a challenge as well as an obligation to translate this genomic information for improved agriculture to feed the anticipated 9 billion people in the next 35 years. We propose to call the systematic application of genome wide sequence information in support of crop improvement as ‘translational genomics for agriculture’ (TGA). Because of its importance and the attention of global plant research community, including the private sector, TGA has been successfully applied in several cereals; maize and rice are the crop species where TGA is most advanced.
In addition to cereals, legumes play important roles both in food and nutritional security as well as in the sustainability of agricultural systems. Among legume species, major food legumes are chickpea (Cicer arietinum), common bean (Phaseolus vulgaris), cowpea (Vigna unguiculata), faba bean (Vicia faba), groundnut (Arachis hypogaea), lentil (Lens culinaris), pea (Pisum sativum), pigeonpea (Cajanus cajan) and soybean (Glycine max). Globally these legumes are grown on 62.8 million ha with a production of 53.2 million tons and fetch about 24.2 billion dollars (http://www.icrisat.org/crp/CRP3.5_Grain_Legumes_15Aug12.pdf). However, the crop productivity of these legumes crops in farmers’ fields in developing countries is generally less than 1 ton per hectare. These statistics indicate that in parallel to improving agronomic practices, advancing genomics research as well as enhanced deployment of TGA in these legume crops is urgently needed.
This review discusses recent advances in genomics research as well as the prospects and potential of TGA in eight leading legume crops namely chickpea, common bean, faba bean, groundnut, lentil, pea, pigeonpea and soybean.
II. NEXT GENERATION GENOMICS
With an objective to understand basic plant biology and use that information to understand and improve important crop species, a few plant species were considered as “model systems.” Their ease of manipulation, simple genome organization, patterns of inheritance, rapid life cycle, desirable experimental properties etc., were important features for a model system (Koebner and Varshney, Citation2006). Model species were supposed to share reasonably good synteny and orthology with genomes of related crop species and help to facilitate discovery of genes and association of genes with phenotypes.
Arabidopsis was the first model plant species for which a genome sequence was produced (Arabidopsis Genome Initiative, 2000). However, with the argument that Arabidopsis may not be good model (Devos et al., Citation1999) and, in particular, does not represent monocot plant species, rice was selected as a second model system for monocot plant species, especially cereals. As rice is also a crop species, the crop community got excited by the prospect of sequencing the rice genome so that rice genes, especially those involved contributing to yield, could be used for crop improvement in this and other plant species. As a result, two draft genomes, one each for japonica and indica rice, were released simultaneously (Goff et al., Citation2002; Yu et al., Citation2002).
Legumes are quite different from other plant species in certain features such as symbiotic nitrogen fixation, protein rich physiology and secondary metabolism. Therefore it was argued that the model systems, i.e. Arabidopsis and rice, did not serve as good models for legumes (Cook, Citation1999; Li et al., Citation2012). Most notably because of the connection between mycorrhization and nodulation in legumes (Duc et al., Citation1989), the former being of wide significance, but not accessible using A. thaliana as a model, and also for the understanding of plant architecture regulation by strigolactones (Gomez-Roldan et al., Citation2008). For these reasons, two legume plant species, Medicago truncatula (Barker et al., Citation1990) and Lotus japonicus (Handberg and Stougaard, Citation1992) were selected as model systems for legumes. Subsequently after spending several years time, and much money, genome sequences were assembled for Lotus (Sato et al., Citation2008) and Medicago (Young et al., Citation2011).
Although genome sequencing used to be restricted to model systems, the advent of NGS technologies and increased expertise in sequence assembly in the scientific community has led to genome projects for other crops. The main rationale behind targeting crop species for sequencing is the often complex genomic composition of crop species and past experience has shown that comparative genomics, epitomized by the ‘model to crop translation’ approach, was not very useful for crop improvement (Koebner and Varshney, Citation2006). An illustration of the sequencing of some key model and crop plant species is shown in .
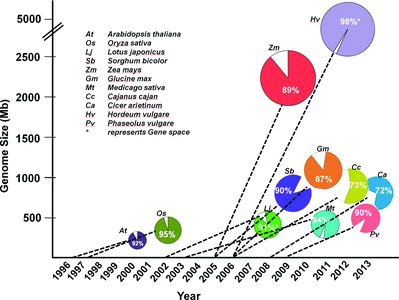
After work began on the model legumes Medicago and Lotus discussed above, soybean was the first crop legume for which reference genome was assembled (Schmutz et al., Citation2010). Legumes like chickpea, common bean and pigeonpea, constitute an important dietary component for the vast majority of populations in many developing countries but did not immediately benefit from the genomic revolution (Varshney et al., Citation2010a). However, as a result of community efforts, draft genome sequences have become available recently for pigeonpea (Varshney et al., Citation2012a) and chickpea (Varshney et al., Citation2013a). These legumes, once considered orphan crops, are no-longer so and now have joined the group of genome resource-rich species (Varshney et al., Citation2010b). Recently the common bean community has developed genome assemblies for two accessions (Scott Jackson, personal communication). Significant efforts are underway in sequencing other important legume genomes such as peanut (http://www.peanutbioscience.com/), pea (http://www.coolseasonfoodlegume.org/pea_genome) and lentil (Judith Burstin, Bert Vandenberg, personal communication). The genome sequences of model legumes, together with those of the above mentioned crops, serve as references to help assemble and organize sequence scaffolds from the related legume crop species (Cannon et al., Citation2009).
In addition to the generation of reference genome sequences, NGS has facilitated re-sequencing of genomes of several varieties/ cultivars/ accessions in order to identify genomic variation, such as SNPs or structural variants, and to develop haplotype maps (Imelfort et al., Citation2009; Lorenc et al., Citation2012). For example in the model legume Medicago, 26 genomes from diverse accessions were sequenced and identified >3 million SNPs and characterized sequence polymorphisms and patterens of linkage disequilibria (LD) (Branca et al., Citation2011). In the case of soybean, 17 wild and 14 cultivated soybean genomes were re-sequenced identifying 205,614 SNPs that revealed patterns of genetic variation between wild and cultivated soybeans (Lam et al., Citation2010). Re-sequencing of 90 chickpea genomes revealed 4.4 million variants (SNPs and INDELs) (Varshney et al., Citation2013a). Re-sequencing enables rapid identification of SNP markers, development of high density genetic maps, and creation of haplotype maps (HapMap) for genome wide association studies (GWAS).
III. TRANSCRIPTOME ASSEMBLIES, FUNCTIONAL MARKERS AND GENE EXPRESSION ATLAS
Global gene expression studies provide insights into the transcriptome at the whole genome level in terms of the response to a particular biological conditions or constraints. These studies, even in the absence of a complete genome sequence, can help in understanding gene function, transcriptional programs, and the molecular basis of various cellular processes. Much work was undertaken in the area of transcriptome and gene expression analysis even before genome sequences became available. Initially, and for the majority of cases, either Sanger sequencing based ESTs or microarray based gene expression analysis was conducted. Using gene expression studies and gene cloning, a number of candidate genes for resistance/ tolerance to several biotic/ abiotic stresses have been isolated or cloned from several legume crops (). In recent years, due to the advent of the NGS technologies, it has become possible to develop de novo transcriptome assemblies with low cost and indeed NGS technologies are being used for gene expression analysis. Transcriptome assemblies developed using either Sanger sequencing or NGS technologies, or a combination of both, have been used for gene annotation for genome sequencing projects. These transcriptome assemblies provide a large number of functional markers (derived from genes/ transcripts) such as SSRs, SNPs, conserved orthologous sequence (COS) and intron spanning region (ISR) markers (Dubey et al., Citation2011; Hiremath et al., Citation2011; Jhanwar et al., Citation2012; Kudapa et al., Citation2012).
TABLE 1 Some candidate genes identified in five legume crops
Using the Roche 454-FLX platform, a transcriptome assembly in M. truncatula was generated with a total of 184,599 contigs (Cheung et al., Citation2006) and this assembly was found to contain 610 SSRs. In the case of Lotus, a tissue-specific transcriptome analysis containing 21,495 transcripts was generated (Takanashi et al., Citation2012). Among legume crop species, several versions of transcriptome assemblies were generated by different research groups (). For instance, in the case of chickpea, several transcriptome assemblies based on different sequencing platforms have been developed (Garg et al., Citation2011a; 2011b; Hiremath et al., Citation2011). These assemblies together with unpublished data from the University of Saskatchewan have been used for defining a comprehensive trasncriptome assembly comprising of 46,369 contigs with an average N50 of 1,726 bp (Kudapa et al., Citation2014). Details of some key transcriptome assemblies based on NGS technology or combination of NGS technologies with Sanger ESTs in select legume species have been given in . The Legume Information System (LIS, http://www.comparative-legumes.org/) hosts transcriptome assemblies for several legume species.
TABLE 2 NGS-based transcriptome assemblies and genomic resources
Mining of transcriptome assemblies with SSR search tools has provided large numbers of SSR markers and these may be validated as genetic markers in wet lab experiments. For example, 250,393 SSRs were identified from 247 faba bean accessions (Yang et al., Citation2012) using 454/FLX sequencing technology. Beyond providing SSR markers, these transcriptome assemblies have been used for identification of SNPs from NGS datasets generated from two or more genotypes (). For instance, in the case of soybean, a total of 1,682 SNPs were identified by deep re-sequencing of the reduced representation libraries using Illumina Genome Analyzer (GA) (Deschamps and Campbell, Citation2010). Similarly, 26,082 SNPs were discovered from two contrasting drought responsive genotypes in chickpea (Hiremath et al., Citation2011), 36,188 SNPs for two contrasting salinity genotypes in pea (Leonforte et al., Citation2013) and 12,141 SNPs from 10 pigeonpea genotypes representing five mapping populations segregating for Fusarium wilt and sterility mosaic disease (Dubey et al., Citation2011) were identified. In addition, COS markers have found wide application in cross-genome comparative studies in legume species. For example, 387 COS markers were developed in chickpea from the transcriptome dataset (Hiremath et al., Citation2011). In some cases, transcriptome assemblies were analyzed for the identification of flanking intron junctions based on alignment to cognate or related genome sequences. As a result many ISR markers have been identified in legumes; for example, 2,088 and 10,009 ISRs were identified in chickpea (Hiremath et al., Citation2011) and pigeonpea (Kudapa et al., Citation2012), respectively and approximately 1,000 have been identified for pea using a comparative genomics approach (http://bioweb.abc.hu/mt/pisprim) and about 5,500 unigenes have been used to generate a consencus genetic map for pea (Bordat et al., Citation2011).
Although vast genomic resources have been developed rapidly in recent years for legumes, gene expression profiling data is insufficient to correlate the developmental processes to transcriptomes, leaving a large gap to associate the phenotype and genome/gene sequence. However, systematic mutant populations have been developed in several species in order to address this issue. TILLING populations are available for L. japonicus, pea, M. truncatula and chickpea (Dalmais et al., Citation2008; Le-Signor et al., Citation2009; Perry et al., Citation2003; Varshney et al., unpublished), Fast Neutron generated deletion mutant populations for M. truncatula, pea and soybean (Bolon et al., Citation2011; Hofer et al., Citation2009; Rogers et al., Citation2009; Smykal et al., Citation2012) and insertion mutant populations generated using exogenous (Medicago, d’Erfurth et al., 2003) and endogeneous retrotransposons (Lotus, Urbański et al., 2012) have been generated and have contributed to gene discovery in these species (see Domoney et al., Citation2013; Domonkos et al., Citation2013; Urbański et al., 2013).
Plant growth and development are controlled by the programmed (by time, tissue and abundance) expression of suites of genes in response to exogeneous or endogeneous queues. Hence, there is a need to generate genome-wide expression data from a range of tissues/ developmental stages in order to understand gene expression profiles in order to relate phenotypes and genotypes in legumes. A gene expression atlas was developed from a comprehensive set of developmental stages and tissues for some important crops such as rice (Wang et al., Citation2010) and maize (Sekhon et al., Citation2011).
In the case of legumes, the M. truncatula Gene Expression Atlas (MtGEA) was developed covering a range of tissues such as roots, nodules, stems, petioles, leaves, vegetative buds, flowers, seeds and seed pods with detailed developmental time-series for nodules and seeds, using the Affymetrix GeneChip Medicago Genome Arrays (Benedito et al., Citation2008). It has also incorporated the transcriptome data from plants subjected to various kinds of abiotic and biotic stresses and data from specific cell and tissue types. These data are useful for gene function determination, biological discovery, and molecular breeding efforts (http://mtgea.noble.org/v3/). Similarly, gene expression atlases have been developed in Lotus (http://ljgea.noble.org/) and soybean (Libault et al., Citation2010; Severin et al., Citation2010) from diverse tissues and developmental time series. Similar kinds of efforts have been initiated in chickpea, pigeonpea and groundnut at ICRISAT. These gene expression atlases are a powerful resource for legume genomics research, which can be used for understanding the regulatory network of developmental processes, tracing the expression profiles of stress responsive candidate genes etc.
In addition to gene expression atlases, efforts have been started to compile protein sequence information for different plant species. For example, all proteins identified for legumes (Medicago, Lotus and soybean) are available at http://bioinfoserver.rsbs.anu.edu.au/utils/PathExpress4legumes/. This database is a valuable resource for comparative seed proteomics and pathway analysis within and beyond the legume family, and protein sequences are exceptionally useful for genome annotation and determining the nature of genes for which only distant relatives are known. Similarly, another legume protein database (LegProt) has been created containing sequences from seven legume species, i.e., M. sativa, M. truncatula, L. japonicas, G. max, P. vulgaris, P. sativum and L. albus (Lei et al., Citation2011; http://bioinfo.noble.org/manuscript-support/legumedb). In addition the Soybean Proteome Database has been generated exclusively for soybean (http://proteome.dc.affrc.go.jp/Soybean/).
IV. COMPREHENSIVE GENETIC MAPS AND PREDICTIVE MARKERS
As previously mentioned, the availability of large-scale genomic resources has led to development of large numbers of molecular markers, particularly SNPs that can be assayed on a range of genotyping platforms. For instance, a variety of SNP genotyping platforms including GoldenGate (Kassa et al., Citation2012), VeraCode (Roorkiwal et al., Citation2013) and Competitive Allele Specific PCR (KASPar) assays (Saxena et al., Citation2012) have been developed for pigeonpea. Such genotyping platforms in combination with classical SSR genotyping platforms have been used to generate large-scale marker segregation data on mapping populations and have led to comprehensive genetic maps (Saxena et al., Citation2012). Several legume crop species did not have good genetic maps until recently. For example, the first SSR-based genetic linkage map for cultivated groundnut was recently developed for the mapping population, TAG 24 × ICGV 86031 (Varshney et al., Citation2009b). Subsequently five more genetic maps were developed based on mapping populations segregating for drought and foliar diseases (Gautami et al., Citation2012a; Ravi et al., Citation2011; Sujay et al., Citation2012). A consensus map for drought tolerance related traits with 293 SSR loci (Gautami et al., Citation2012a) and foliar disease resistance with 225 SSR loci (Sujay et al., Citation2012), respectively have been developed. Furthermore, international collaborative efforts have resulted in the construction of consensus map with 897 SSR marker loci using genotyping data from 11 mapping populations. This consensus map was upgraded with maps for two more populations and the updated version map covers 2,651 cM with 3,693 marker loci anchored to 20 consensus linkage groups (LG) corresponding to the A and B genomes (Shirasawa et al., Citation2013). Another example of development of comprehensive genetic maps is in cowpea, where a 1,536-SNP GoldenGate genotyping array was developed and applied to 741 recombinant inbred lines from six mapping populations. 1,375 out of 1,536 SNPs provided dependable markers and a subset of 928 were incorporated to develop a consensus genetic map spanning 680 cM with 11 linkage groups and an average marker distance of 0.73 cM (Muchero et al., Citation2009). This map has been further updated and the consensus map has 1,107 SNP loci (Lucas et al., Citation2011).
Details on genetic maps in selected legume crops are listed in . These dense consensus genetic maps are useful resources for the identification of highly informative and well distributed markers to be used for background selection in breeding schemes, the construction of new genetic maps or analysis of genetic diversity. Analysis of genetic maps, together with trait phenotyping on the respective segregating populations provides predictive markers for traits of interest. Both biotic and abiotic stresses are complex in nature, so trait dissection is important for improving component traits through breeding. Often agronomical traits are composites of many underlying phenothypes, but their dissection into component, genetically determined, contributions represent the underlying biology and can be used for marker-based breeding. Both bulked segregant analysis (BSA) as well as quantitative trait loci (QTL) mapping based marker analysis, have been used to identify markers linked to these genetic determinants of traits. In fact, quantitative traits have been studied in legumes since Mendel. In his 1866 paper he commented on the near continuous variation of flower colour in Phaseolus crosses: “Even these enigmatical results, however, might probably be explained by the law governing Pisum if we might assume that the color of the flowers and seeds of Ph. multiflorus is a combination of two or more entirely independent colors, which individually act like any other constant character in the plant” [http://www.mendelweb.org/]
TABLE 3 List of some comprehensive genetic maps for selected legumes
For simple inherited traits, BSA has been used in several plant species including the legumes discussed in this article. For instance, BSA was used for mapping of Fusarium wilt (FW) resistance with RAPD markers in the case of pigeonpea (Kotresh et al., Citation2006). On the other hand, in order to understand the complex nature of drought tolerance in chickpea, precise phenotypic data for 20 different root traits was analyzed using a QTL mapping approach. As a result, nine QTL clusters containing QTLs for several drought tolerance traits have been identified that are being targeted for chickpea molecular breeding program. Among these QTL clusters, one QTL cluster corresponding to QTLs for 12 traits and explaining about 60% phenotypic variation has been identified on CaLG04, referred to as a “QTL-hotspot” (Varshney et al., Citation2013b). Similar mapping efforts have been undertaken for important the biotic stresses like FW (Benko-Iseppon et al., Citation2003; Cobos et al., Citation2005; Sabbavarapu et al., Citation2013), and Aschochyta blight (Anbessa et al., Citation2009; Aryamanesh et al., Citation2010; Kottapalli et al., Citation2009).
One agronomic trait under complex genetic control (Buckler et al., Citation2009; Mouradoy et al., 2002) that has received much attention is flowering time. This was studied as a quantitative trait in rice and pea at the beginning of the 20th century (Hoshino, Citation1915), and the physiological genetics of flowering time in pea was a model for this that was eclipsed by the rise of Arabidopsis as an experimental system. The various lineages of angiosperm families diverged about 100 to 150 million years ago (Bell et al., Citation2010), so it is not surprising that many structural genes that regulate this pathway are common to different families, but that their regulatory circuitry differs. In the legumes (that diversified 55 – 60 million years ago) the study of the regulation of flowering time is a resurgent and the novelty of the genetic control of flowering time in legumes is being revealed (Hecht et al., Citation2011; Mach, Citation2011; Weller et al., Citation2012) with strong indications that floral induction in pea may involve the action of several members of the FT family with distinct modes of action (Hecht et al., Citation2011). Thus some major determinants of flowering time are known, so the underlying genes can be used (if necessary) to follow this trait. However the Buckler et al. (Citation2009) analysis of the control of flowering time in maize suggests that there may also be many loci of small effect that together contribute greatly to the genetic variation of this trait. Some key studies on identification of QTLs and predictive markers associated with agronomically important traits in addition to tolerance/ resistance to abiotic and biotic stresses in select legumes are summarized in .
TABLE 4 Summary of some trait mapping studies in select legumes
V. SUCCESS STORIES OF TGA IN LEGUMES
Molecular breeding is a direct strategy to translate genome information for developing products i.e. superior lines for traits of interest. In general, in addition to MAS, three main approaches namely marker-assisted backcrossing (MABC), marker-assisted recurrent selection (MARS) and genomic selection (GS) can be used in molecular breeding. Several success stories utilizing molecular breeding have been reported in crop species such as rice, maize, wheat (see Kulwal et al., Citation2011). With the notable exception of soybean in the commercial sector, for legumes, in general, molecular breeding has been delayed due to limited availability of genomic resources. However, recent advances in genomics especially in the legumes discussed here have opened the avenues for accelerating practicing of TGA. An overview of integrative genomics for accelerated crop improvement is illustrated in .
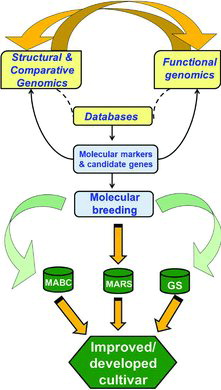
MAS has been extensively deployed for development and release of improved lines/varieties in legumes, some key examples are summarized in . In the case of soybean, in addition to developing improved lines or varieties for resistance to different biotic stresses like soybean cyst nematode (SCN) races (Cahill and Schmidt, Citation2004; Concibido et al., Citation1996), phytophthora root rot and brown stem rot (Cahill and Schmidt, Citation2004), insect resistance (Narvel et al., Citation2001; Walker et al., Citation2002; Warrington et al., Citation2008), mosaic virus (Saghai-Maroof et al., Citation2008; Shi et al., Citation2009), MAS products were also developed for low linolenic acid content (Sauer et al., Citation2008) and yield (Concibido et al., Citation2003). Furthermore, SNP markers tightly linked to soybean root-knot nematode resistance QTL have enhanced the efficiency of MAS (http://www.plantmanagementnetwork.org/pub/php/news/2007/Shortcut/). Similarly, in case of common bean, efforts at CIAT focused mainly in deploying single genes for enhancing resistance to viral diseases. As a result, the cultivar Perola with enhanced resistance bean Anthrocnose in Brazil (Raganin et al., 2003), pinto beans in the United States (Miklas et al., Citation2003) and Andean climbing bean in Mexico / Colombia (Garzon et al., 2008) have been developed. In cowpea, SCAR markers have been used in discriminating Striga resistant and susceptible lines (Omoigui et al., Citation2012).
TABLE 5 Key success stories of TGA in legume crops
MABC is the simplest way to develop superior lines by transfering QTLs with large phenotypic effect using tightly linked molecular markers to a given trait for foreground selection and genome-wide unlinked markers for background selection or recovery of the recurrent parent genome. In particular MABC is useful to capture major QTL(s)/gene(s) with a strong association to the given trait of interest. For example in groundnut, MABC has been used to develop and release an improved variety; markers linked with root-knot nematode (Meloidogyne arenaria) resistance were used for introgression through the amphidiploid pathway into cultivated groundnut (Simpson et al., Citation2001). Identification of linked markers due to sequence divergence between diploid and tetraploid genomes (Chu et al., Citation2007; Nagy et al., Citation2010) was relatively easy in groundnut. The simultaneous introgression of nematode resistance and oil quality was achieved using a recessive AhFAD2B allele (which controls high ratio of oleic: linoleic acid (O/L)) and linked markers for foreground selection. These efforts led to the release of the improved Tiftguard variety “Tifguard High O/L” (Chu et al., Citation2011). The MABC approach has been initiated to introgress a major QTL contributing ca. 80% of the phenotypic variation for leaf rust into the genetic background of three elite cultivars namely ICGV 91114, JL 24 and TAG 24. By using 2–3 rounds of backcrossing and selfing, BC2F3 and BC3F2 homozygous lines have been developed at ICRISAT.
Two major MABC projects are underway in chickpea at ICRISAT and its partner organizations. Under the Tropical Legume-I initiative of CGIAR Generation Challenge Programme in collaboration with Bill & Melinda Gates Foundation, significant efforts have been made to develop drought tolerant progenies (BC3F3:4) in the genetic background of JG11, a leading variety in India, by transferring a genomic region, “QTL-hotspot,” that contains several QTLs for drought tolerance traits (Varshney et al., Citation2013b). Detailed phenotypic evaluation of these lines is underway in India, Kenya and Ethiopia and preliminary results are promising (unpublished). The second initiative is part of the Accelerated Crop Improvement Programme (ACIP) of Department of Biotechnology, Government of India. In this initiative, efforts are being made to introgress resistance to different races independently as well as pyramiding of resistance to two races for FW in some elite varieties in India. ICRISAT (India) is pyramiding resistances for Foc1 and Foc3 from WR 315 and 2 QTLs for Ascochyta blight (AB) resistance from ILC 3279 line into C 214 (Varshney et al., Citation2013c).
However, the majority of traits targeted by breeders e.g. drought tolerance or durable resistance to multiple races of pathogens are controlled by multiple QTLs or genes. In such cases, retaining desirable gene combinations or pyramiding of several QTLs through the MABC approach is a challenging task (Peleman and Voort, Citation2003). Hence, marker assisted recurrent selection (MARS), has been introduced as an alternative approach and demonstrated experimentally to be more effective in accumulating a large number of smaller effect QTLs (Ribaut et al., Citation2010; Ribaut and Ragot, Citation2007). MARS, on the other hand, aims to take advantage of QTL information generated on breeding populations to develop superior lines with an optimum combination of favourable alleles originating from both the parents. QTL alleles are accumulated through successive intercrossing using only genotypic selection. Recombined lines are then subjected to a final phenotypic screening to select the best varieties to release. MARS can be initiated without any prior knowledge of QTLs with the objective of discovering and harnessing the superior QTLs/alleles during the scheme itself, which is not possible in MABC. However, it is observed that existing marker-trait associations enhance the quantum of genetic gains in MARS scheme (Bernardo and Charcosset, Citation2006). The recurrent-selection method is routinely used mainly in cross-pollinated crops like maize and, this process can be improved with the help of molecular markers, which is why the process is called marker-assisted recurrent selection (MARS). While several multinational companies have been using MARS in crops like maize and soybean, only a few public sector institutes have used MARS in crops likes wheat (Charmet et al., Citation2001), sorghum (Abdallah et al., Citation2009) and rice (Grenier et al., Citation2012). MARS based programs have been initiated in cowpea (see Ribaut et al., Citation2012) targeting improvement in drought tolerance. Some efforts have been initiated to use MARS in the case of chickpea, for assembling favorable alleles for drought tolerance using ICCV 04112 × ICCV 93954 and ICCV 05107 × ICCV 94954.
Genomic selection (GS) is a novel approach in which genetic markers covering the whole genome are used so that all (QTL) are in linkage disequilibrium with at least one marker. GS focuses on the selection of desirable genotypes on the basis of an index derived from genome-wide DNA marker information. The index is designated as genomic estimated breeding values (GEBVs) and the phenomenon of selecting individuals on GEBV basis is referred as genomic selection (GS) (Heffner et al., Citation2009). Genotyping and phenotyping of a ‘training population’ is required to train the GS models or extensively calculate GEBV estimates (Nakaya and Isobe, Citation2012). The GEBV estimates thus obtained are subsequently used for selection of genotypes from ‘breeding population.’ In GS, no phenotyping is required for the ‘breeding population’, however all the individuals are genotyped thoroughly. GS efficiently exploits the high density marker data available at reasonable cost and can also reduce the selection cycle length of a breeding program where it could take several seasons to develop reliable phenotypic data. In brief, GS as an approach relies on cycles of prediction and testing by phenotyping the training population as the process proceeds and this constant checking of the selection index vs the training population overcomes type I errors associated with multiple loci contributing small effects to many quantitative traits.
For estimating the GEBVs with high precision, an important component of GS, use of an appropriate statistical model is very critical. Among different models of GS, GEBVs predicted using either best linear unbiased prediction (BLUP) or Bayesian methods are effective according to simulation studies (Bernardo and Yu, Citation2007). In addition to a Bayesian method, Bayes B, another method called wBSR (weighed Bayesian Shrinkage Regression) which reduces the computational burden on MCMC-based Bayesian methods is considered to be a method of choice for genomic selection (Takeshi and Hiroyoshi, Citation2010). Cornell University (Jannink et al., Citation2010), the University of Minnesotta (Bernardo and Yu, Citation2007) and Hohenheim University (Piepho, Citation2009) also developed statistical models and/or pursued applications of GS in breeding of some major crops like maize, wheat. Though GS has not been used in any legume species at present, mostly due to lack of: (i) historical phenotyping data on several breeding lines (that can be used for the training population), (ii) big linkage disequilibrium (LD) blocks in breeding populations, and (iii) genome wide marker genotyping system like DArT or SNP markers, ICRISAT has initiated the efforts to deploy GS in chickpea and groundnut.
VI. CHALLENGES AND OPPORTUNITIES
Whole genome sequences of both model and crop legumes are expected to offer new perspectives for TGA in legume species. From the examples presented above it is evident that ongoing genomics research could accelerate crop improvement programs in legume crops. In the pre-genomics era, comparative genomics approach was successful for identifying homologues/ orthologues or cloning genes in several legumes by using information from model plant systems. For example, conservation of Arabidopsis flowering time genes was studied in legumes and preliminary survey revealed that legume flowering-related genes (LFY and TFL1) identified significant differences in function to Arabidopsis counterparts (Hecht et al., Citation2005). However, this approach was not very successful for developing superior varieties in legume crops. In the post-genomics era, availability of genome sequence information as well as species specific genomic resources offer greater opportunities for TGA even in the crops like lentil, pea and fababean where limited information is available. However, so far, success stories of TGA are limited in the targeted legume crops. In this context, there is a series of challenges that include: (i) availability of limited predictive molecular markers for target traits, (ii) limited access to marker technologies, (iii) limited availability of high-throughput, cost-effective and precise phenotyping platforms, (iv) lack of capacity both human as well as infrastructure and (v) absence of suitable data management systems (Varshney et al., Citation2012b). High-throughput sequencing and genotyping facilities available in different countries could benefit from economies of scale. Although sample shipment across national borders sometimes poses logistic and quarantine challenges, especially in the developing world, access to those centers could facilitate deployment of molecular breeding in the legume crops. For modernization of the breeding programs, it is important to deploy decision support tools (computational tools) in every step of the process of breeding. The Integrated Breeding Platform initiative (www.integratedbreeding.net), houses a range of decision support tools that can be used in a distributed way for example by National Agricultural Research Systems (NARS) in their breeding programs.
Although conventional breeding based exclusively on phenotypic selection remains the mainstay for most breeding programs in these legumes, adoption of TGA is increasing and in some cases is superseding conventional approaches. With advances in genomics, the ultimate aim is to increase the rate of genetic gain across target environments, in less time and at lower cost compared to conventional selection. Nonetheless, it is also important to mention the role of quality phenotypic data. Efforts need to be initiated to establish field based high throughput and cost effective phenotyping platforms. As plants are exposed to range of stresses, breeders in general perform selection based on the plant's overall performance and not just for a single trait. Therefore GS seems to be an ideal approach because it involves selection of the plants based not on just one trait but for overall performance of the plant based on whole genome marker profiling data. We believe that centralized genotyping service facilities, together with access to genomics and breeding analytical tools (decision support tools), should enhance implementation of TGA in these legume crops. Furthermore web accessible mutant populations are available for Medicago, Lotus and soybean. Developing such collections in other crop legumes may fasten the breeding programs. It is also important to continue the training of breeders and geneticists in integrated genomics and molecular breeding. Effective implementation of TGA in the legume crops will lead to crop improvement programs for ensuring food security in developing countries.
Additional information
Funding
REFERENCES
- Abdallah, A.A., Ali, A.M., Geiger, H.H., and Parzies, H.K. 2009. Marker-assisted recurrent selection for increased outcrossing in Caudatum–race Sorghum. In: International Conference on Applied Biotechnology (ICAB), September 28–30, Khartoum, Sudan. [Abstract no. 04].
- Agarwal, G., Jhanwar, S., Priya, P., Singh, V.K., Saxena, M.S., Parida, S.K., Garg, R., Tyagi, A.K., and Jain, M. 2012. Comparative analysis of kabuli chickpea transcriptome with desi and wild chickpea provides a rich resource for development of functional markers. PloS ONE 7: e52443.
- Ahn, S.N. and Tanksley, S.D. 1993. Comparative linkage maps of the rice and maize genomes. Proc. Natl. Acad. Sci. U S A 90: 7980–7984.
- Anbessa, Y., Taran, B., Warkentin, T.D., Tullu, A., and Vandenberg, A. 2009. Genetic analyses and conservation of QTL for Ascochyta blight resistance in chickpea. Theor. Appl. Genet. 119: 757–765.
- Anuradha, C., Gaur, P.M., Pande, S., Gali, K.K., Ganesh, M., Kumar, J., and Varshney, R.K. 2011. Mapping QTL for resistance to botrytis grey mould in chickpea. Euphytica 182: 1–9.
- Arbaoui, M., Balko, C., and Link, W. 2008. Study of faba bean (Vicia faba L.) winter-hardiness and development of screening methods. Field Crop Res. 106: 60–67.
- Arelli, P.R., Pantalone, V.R., Allen, F.L., and Mengrstu, A. 2007. Registration of soybean germplasm JIN-5303. J. Plant Regist. 1: 69–70.
- Arelli, P.R., Young, L.D., and Menglstu, A. 2006. Registration of high yielding and multiple disease resistant soybean germplasm JTN-5503. Crop Sci. 46: 2723–2724.
- Aryamanesh, N., Nelson, M.N., Yan, G., Clarke H.J., and Siddique K.H. M. 2010. Mapping a major gene for growth habit and QTLs for Ascochyta blight resistance and flowering time in a population between chickpea and Cicer reticulatum. Euphytica 173: 307–319.
- Atibalentja, N., Bekal, S., Domier, L.L., Niblack, T.L., Noel, G.R., and Lambert K.N. 2005. A genetic linkage map of the soybean cyst nematode Heterodera glycines. Mol. Gen. Genomics 273: 273–281.
- Avila, C.M., Satovic, Z., Sillero, J.C., Nadal, S., Rubiales, D., Moreno, M.T., and Torres, A.M. 2005. QTL detection for agronomic traits in faba bean (Vicia faba L.). Agric. Conspec. Sci. 3: 65–73.
- Avila, C.M., Satovic, Z., Sillero, J.C., Rubiales, D., Moreno, M.T., and Torres, A.M. 2004. Isolate and organ-specific QTLs for ascochyta blight resistance in faba bean. Theor. Appl. Genet. 108: 1071–1078.
- Avila, C.M., Sillero, J.C., Rubiales, D., Moreno, M.T., and Torres, A.M. 2003. Identification of RAPD markers linked to the Uvf-1 gene conferring hypersensitive resistance against rust (Uromyces viciae-fabae) in Vicia faba L. Theor. Appl. Genet. 107: 353–358.
- Barker, D., Bianchi, S., Blondon, F., Dattee, Y., Duc, G., Essad, S., Flament, T., Gallusci, T., Genier, G., Guy, P., Muel, X., Tourner, J., Denarie, J., and Huguet, T. 1990. Medicago truncatula, a model plant for studying the molecular genetics of the Rhizobium-legume symbiosis. Plant Mol. Biol. Rep. 8: 40–49.
- Barilli, E., Satovic, Z., Rubiales, D., and Torres, A.M. 2010. Mapping of quantitative trait loci controlling partial resistance against rust incited by Uromyces pisi (Pers.) Wint. in a Pisum fulvum L. intraspecific cross. Euphytica 175: 151–159.
- Beebe, S.E., Rojas, M., Yan, X., Blair, M.W., Pedraza, F., Muñoz, F., Tohme, J., and Lynch, J.P. 2006. Quantitative trait loci for root architecture traits correlated with phosphorus acquisition in common bean. Crop Sci. 46: 413–423.
- Bell, C.D., Soltis, E.S., and Soltis, P.S. 2010. The age and diversification of the angiosperms re-revisited. Am. J. Bot. 97: 1296–1303.
- Benedito, V.A., Torres-Jerez, I., Murray, J.D., Andriankaja, A., Allen, S., Kakar, K., Wandrey, M., Verdier, J., Zuber, H., Ott, T., Moreau, S., Niebel, A., Frickey, T., Weiller, G., He, J., Dai, X., Zhao, P.X., Tang, Y., and Udvardi, M.K. 2008. A gene expression atlas of the model legume Medicago truncatula. Plant J. 55: 504–513.
- Benko-Iseppon, A.M., Winter, P., Huettel, B., Staginnus, C., Muehlbauer, F.J., and Kahl, G. 2003. Molecular markers closely linked to fusarium resistance genes in chickpea show significant alignments to pathogenesis-related genes located on Arabidopsis chromosomes 1 and 5. Theor. Appl. Genet. 107: 379–386.
- Bernardo, R. and Charcosset, A. 2006. Usefulness of gene information in marker-assisted recurrent selection: a simulation appraisal. Crop Sci. 46: 614–621.
- Bernardo, R. and Yu, J. 2007. Prospects for genome wide selection for quantitative traits in maize. Crop Sci. 47: 1082–1090.
- Blair, M.W., Muñoz, C., Buendía, H.F., Flower, J., Bueno, J.M., and Cardona, C. 2010. Genetic mapping of microsatellites markers around the arcelinbruchid resistance locus in common bean. Theor. Appl. Genet. 121: 393–402.
- Blair, M.W., Muñoz, C., Garza, R., and Cardona, C. 2006. Molecular mapping of genes for resistance to the bean pod weevil (Apiongodmani Wagner) in common bean. Theor. Appl. Genet. 112: 913–923.
- Blair, M.W., Rodriguez, L.M., Pedraza, F., Morales, F., and Beebe, S. 2007. Genetic mapping of the bean golden yellow mosaic geminivirus resistance gene bgm-1 and linkage with potyvirus resistance in common bean (Phaseolus vulgaris L.). Theor. Appl. Genet. 114: 261–271.
- Bohra, A., Dubey, A., Saxena, R.K., Penmetsa, R.V., Poornima, K.N., Kumar, N., Farmer, A.D., Srivani, G., Upadhyaya, H.D., Gothalwal, R., Ramesh, S., Singh, D., Saxena, K., KaviKishor, P.B., Singh, N.K., Town, C.D., May G.D., Cook, D.R., and Varshney, R.K. 2011. Analysis of BAC-end sequences (BESs) and development of BES-SSR markers for geneticmapping and hybrid purity assessment in pigeonpea. BMC Plant Biol. 11: 56.
- Bohra, A., Saxena, R.K., Gnanesh, B.N., Saxena, K.B., Byregowda, M., Rathore, A., KaviKishor, P.B., Cook, D.R., and Varshney, R.K. 2012. An intra-specific consensus genetic map of pigeonpea [Cajanus cajan (L.) Millspaugh] derived from six mapping populations. Theor. Appl. Genet. 125: 1325–1338.
- Bolon, Y.T., Haun, W.J., Xu, W.W., Grant, D., Stacey, M.G., Nelson, R.T., Gerhardt, D.J., Jeddeloh, J.A., Stacey, G., Muehlbauer, G.J., Orf, J.H., Naeve, S.L., Stupar, R.M., and Vance, C.P. 2011. Phenotypic and genomic analyses of a fast neutron mutant population resource in soybean. Plant Physiol. 156: 240–253.
- Bordat, A., Savois, V., Nicolas, M., Salse, J., Chauveau, A., Bourgeois, M., Potier, J., Houtin, H., Rond, C., Murat, F., Marget, P., Aubert, G., and Burstin, J. 2011. Translational genomics in legumes allowed placing in silico 5460 unigenes on the pea functional map and identified candidate genes in Pisum sativum L. G3. Genes-Genomes-Genetics 2: 93–103.
- Branca, A., Paape, T., Zhou, P., Briskine, R., Farmer, A.D., Mudge, J., Bharti, A.K., Woodward, J.E., May, G.D., Gentzbittel, L., Ben, C., Denny, R., Sadowsky, M.J., Ronfort, J., Bataillon, T., Young, N.D., and Tiffin, P. 2011. Whole-genome nucleotide diversity, recombination, and linkage-disequilibrium in the model legume Medicago truncatula. Proc. Natl. Acad. Sci. U S A. 108: 864–870.
- Buckler, E.S., Holland, J.B., Bradbury, P.J., Acharya, C.B., Brown, P.J., Browne, C., Ersoz, E., Flint-Garcia, S., Garcia, A., Glaubitz, J.C., Goodman, M.M., Harjes, C., Guill, K., Kroon, D.E., Larsson, S., Lepak, N.K., Li, H., Mitchell, S.E., Pressoir, G., Peiffer, J.A., Oropeza Rosas, M., Rocheford, T.R., Romay, M.C., Romero, S., Salvo, S., Sanchez Villeda, H., Sofia da Silva, H., Sun, Q., Tian, F., Upadyayula, N., Ware, D., Yates, H., Yu, J., Zhang, Z., Kresovich, S., and McMullen, M.D. 2009. The genetic architecture of maize flowering time. Science 325: 714–718.
- Burstin, J., Marget, P., Huart, M., Moessner, A., Mangin, B., Duchene, C., Desprez, B., Munier-Jolian, N., and Duc, G. 2007. Developmental genes have pleiotropic effects on plant morphology and source capacity, eventually impacting on seed protein content and productivity in pea. Plant Physiol. 144: 768–781.
- Cahill, D.J. and Schmidt, D.H. 2004. Use of marker assisted selection in a product development breeding program. In: Proceedings of the 4th International Crop Science Congress, 26 September-1 October, Brisbane, Australia.
- Cannon, S.B., May, G.D., and Jackson, S.A. 2009. Three sequenced legume genomes and many crop species: rich opportunities for translational genomics. Plant Physiol. 151: 970–977.
- Carneiro, F.F., dos Santos, J.B., and Leite, M.E. 2010. Marker-assisted backcrossing using microsatellites and validation of SCAR Phs marker for resistance to white mold in common bean. Electron. J. Biotechnol. 13: 9–10.
- Casañas, F., Pérez-Vega, E., Almirall, A., Plans, M., Sabaté, J., and Ferreira, J.J. 2013. Mapping of QTL associated with seed chemical content in a RIL population of common bean (Phaseolus vulgaris L.). Euphytica 192: 279–288.
- Charmet, G., Robert, N., Perretant, M.R., Gay, G., Sourdille, P., Groos, C., Bernard, S., and Bernard, M. 2001. Marker assisted recurrent selection for cumulating QTLs for bread-making related traits. Euphytica 119: 89–93.
- Checa, O.E. and Blair, M.W. 2008. Mapping QTL for climbing ability and component traits in common bean (Phaseolus vulgaris L.). Mol. Breeding 22: 201–215.
- Chen, M., Xu, Z., Xia, Z., Li, L., Cheng, X., Dong, J., Wang, Q., and Ma, Y. 2009. Cold-induced modulation and functional analyses of the DRE-binding transcription factor gene, GmDREB3, in soybean (Glycine max L.). J. Exp. Bot. 60: 121–135.
- Cheung, F., Haas, B.J., Goldberg, S.M. D., May, G.D., Xiao, Y., and Town, C.D. 2006. Sequencing Medicago truncatula expressed sequenced tags using 454 Life Sciences technology. BMC Genomics 7: 272.
- Cho, S.H., Chen, W.D., and Muehlbauer, F.J. 2004. Pathotype-specific genetic factors in chickpea (Cicer arietinum L.) for quantitative resistance to Aschochyta blight. Theor. Appl. Genet. 109: 733–739.
- Cho, S., Kumar, J., Shultz, J.K., Anupama, K., Tefera, F., and Muehlbauer, F.J. 2002. Mapping genes for double podding and other morphological trait in chickpea. Euphytica 125: 285–292.
- Choi, P., Mano, Y., Ishikawa, A., Odashima, M., Umezawa, T., Fujimura, T., Takahata, Y., and Komatsuda, T. 2010. Identification of QTLs controlling somatic embryogenesis using RI population of cultivar × weedy soybean. Plant Biotechnol. Reports 4: 23–27.
- Choudhary, S., Gaur, R., Gupta, S., and Bhatia, S. 2012. EST-derived genic molecular markers: development and utilization for generating an advanced transcript map of chickpea. Theor. Appl. Genet. 124: 1449–1462.
- Chu, Y., Wu, C.L., Holbrook, C.C., Tillman, B.L., Person, G., and Ozias-Akins, P. 2011. Marker-assisted selection to pyramid nematode resistance and the high oleic trait in peanut. Plant Gen. 4: 110–117.
- Chu, Y., Holbrook, C.C., Timper, P., and Ozias-Akins, P. 2007. Development of a PCR-based molecular marker to select for nematode resistance in peanut. Crop Sci. 47: 841–845.
- Cober, E.R., Molnar, S.J., Charette, M., and Voldeng, H.D. 2010. A new locus for early maturity in soybean. Crop Sci. 50: 524–527.
- Cobos, M.J., Fernandez, M., Rubio, J., Kharrat, M., Moreno, M.T., Gil, J., and Millan, T. 2005. A linkage map of chickpea (Cicer arietinum L.) based on populations from Kabuli x Desi crosses: location of genes for resistance to Fusarium wilt race 0. Theor. Appl. Genet. 110: 1347–1353.
- Cobos, M.J., Rubio, J., Fernandez, M., Garza, R., Moreno, M.T., and Millan, T. 2007. Genetic analysis of seed size, yield and days to flowering in a chickpea recombinant inbred line population derived from a Kabuli × Desi cross. Ann. Appl. Biol. 151: 33–42.
- Cobos, M.J., Rubio, J., Strange, R.N., Moreno, M.L., Gil J., and Millan, T. 2006. A new QTL for Ascochyta blight resistance in an RIL population derived from an interspecific cross in chickpea. Euphytica 149: 105–111.
- Cobos, M.J., Winter, P., Kharrat, M., Cubero, J.I., Gil, J., Millan, T., and Rubio, J. 2009. Genetic analysis of agronomic traits in a wide cross of chickpea. Field Crops Res. 111: 130–136.
- Concibido, V.C., Denny, R.L., Lange, D.A., Orf, J.H., and Young, N.D. 1996. RFLP mapping and marker-assisted selection of soybean cyst nematode resistance in PI 209332. Crop Sci. 36: 1643–1650.
- Concibido, V., La Vallee, B., Mclaird, P., Pineda, N., Meyer, J., Hummel, L., Yang, J., Wu, K., and Delannay, X. 2003. Introgression of a quantitative trait locus for yield from Glycine soja into commercial soybean cultivars. Theor. Appl. Genet. 106: 575–582.
- Cook, D.R. 1999. Medicago truncatula—a model in the making! Curr Opin Plant Biol. 2: 301–304.
- Córdoba, J.M., Chavarro, C., Schlueter, J.A., Jackson, S.A., and Blair, M.W. 2010. Integration of physical and genetic maps of common bean through BAC-derived microsatellite markers. BMC Genomics 11: 436.
- Corrêa, R.X., Costa, M.R., Good-God, P.I., Ragagnin, V.A., Faleiro, F.G., Moreira, M.A., and Barros, E.G. 2000. Sequence characterized amplified regions linked to rust resistance genes in the common bean. Crop Sci. 40: 804–807.
- Cruz-Izquierdo, S., Avila, C.M., Satovic, Z., Palomino, C., Gutierrez, N., Ellwood, S.R., Phan, H., Cubero, J., and Torres, A. 2012. Comparative genomics to bridge Vicia faba with model and closely-related legume species: stability of QTLs for flowering and yield-related traits. Theor. Appl. Genet. 125: 1767–1782.
- Dalmais, M., Schmidt, J., Le Signor, C., Moussy, F., Burstin, J., Savois, V., Aubert, G., Brunaud, V., Oliveira, Y., Guichard, C., Thompson, R., and Bendahmane, A. 2008. UTILLdb, a Pisum sativum in silico forward and reverse genetics tool. Genome Biol. 9: R43.
- de Ronde, J.A., Laurie, R.N., Caetano, T., Greyling, M.M., and Kerepesi, I. 2004. Comparative study between transgenic and non-transgenic soybean lines proved transgenic lines to be more drought tolerant. Euphytica 138: 123–132.
- d’Erfurth, I., Cosson, V., Eschstruth, A., Lucas, H., Kondorosi, A., and Ratet, P. 2003. Efficient transposition of the Tnt1 tobacco retrotransposon in the model legume Medicago truncatula. Plant J. 34: 95–106.
- Deschamps, S. and Campbell, M.A. 2010. Utilization of next-generation sequencing platforms in plant genomics and genetic variant discovery. Mol. Breeding 25: 553–570.
- Deschamps, S., Rota, M., Ratashak, J.P., Biddle, P., Thureen, D., Farmer, A., Luck, S., Beatty, M., Nagasawa, N., Michael, L., Llaca, V., Sakai, H., May, G., Lightner, J., and Campbell, M.A. 2010. Rapid genome-wide single nucleotide polymorphism discovery in soybean and rice via deep resequencing of reduced representation libraries with the Illumina genome analyzer. Plant Gen. 3: 53–68.
- Devos, K.M., Beales, J., Nagamura, Y., and Sasaki, T. 1999. Arabidopsis-rice: will colinearity allow gene prediction across the eudicot-monocot divide? Genome Res. 9: 825–829.
- Dhanasekar, P., Dhumal, K.H., and Reddy, K.S. 2010. Identification of RAPD marker linked to plant type gene in pigeonpea. Indian J. Biotechnol. 9: 58–63.
- Díaz-Ruiz, R., Satovic, Z., Ávila, C.M., Alfaro, C.M., Gutierrez, M.V., Torres, A.M., and Román, B. 2009b. Confirmation of QTLs controlling Ascochyta fabae resistance in different generations of faba bean (Vicia faba L.). Crop Pasture Sci. 60: 353–361.
- Díaz-Ruiz, R., Torres, A., Gutierrez, M.V., Rubiales, D., Cubero, J.I., Kharrat, M., Satovic, Z., and Román, B. 2009a. Mapping of quantitative trait loci controlling Orobanche foetida Poir. resistance in faba bean (Vicia faba L.). Afr. J. Biotechnol. 8: 2718–2724.
- Dirlewanger, E., Isaac, P., Ranade, S., Belajouza, M., Cousin, R., and Devienne, D. 1994. Restriction fragment length polymorphism analysis of loci associated with disease resistance genes and developmental traits in Pisum sativum L. Theor. Appl. Genet. 88: 17–27.
- Domoney, C., Knox, M., Moreau, C., Ambrose, M., Palmer, S., Smith, P., Christodoulou, V., Isaac, P.G., Hegarty, M., Blackmore, T., Swain, M., and Ellis, N. 2013. Exploiting a fast neutron mutant genetic resource in Pisum sativum L. (pea) for functional genomics. Funct. Plant Biol. 40: 1261–1270.
- Domonkos, A., Horvath, B., Marsh, J.F., Halasz, G., Ayaydin, F., Oldroyd, G.E. D., and Kalo, P. 2013. The identification of novel loci required for appropriate nodule development in Medicago truncatula. BMC Plant Biol. 13: 157.
- Dubey, A., Farmer, A., Schlueter, J., Cannon, S.B., Abernathy, B., Tuteja, R., Woodward, J., Shah, T., Mulasmanovic, B., Kudapa, H., Raju, N.L., Gothalwal, R., Pande, S., Xiao, Y., Town, C.D., Singh, N.K., May, G.D., Jackson, S., and Varshney, R.K. 2011. Defining the transcriptome assembly and its use for genome dynamics and transcriptome profiling studies in pigeonpea (Cajanus cajan L.). DNA Res. 18: 153–164.
- Duc, G., Trouvelot, A., Gianinazzi-Pearson, V., and Gianinazzi, S. 1989. First report of non-mycorrhizal plant mutants (Myc−) obtained in pea (Pisum sativum L.) and fababean (Vicia faba L.). Plant Sci. 60: 215–222.
- Dumont, E., Fontaine, V., Vuylsteker, C., Sellier, H., Bodèle, S., Voedts, N., Devaux, R., Frise, M., Avia, K., Hilbert, J.L., Bahrman, N., Hanocq, E., Lejeune-Hénaut, I., and Delbreil, B. 2009. Association of sugar content QTL and PQL with physiological traits relevant for frost damage resistance in pea under field and controlled conditions. Theor. Appl. Genet. 118: 1561–1571.
- Dutta, S., Kumawat, G., Singh, B.P., Gupta, D.K., Singh, S., Dogra, V., Gaikwad, K., Sharma, T.R., Raje, R.S., Bandhopadhy, T.K., Datta, S., Singh, M.N., Bashasab, F., Kulwal, P., Wanjari, K.B., Varshney, R.K., Cook, D.R., and Singh, N.K. 2011. Development of genic-SSR markers by deep transcriptome sequencing in pigeonpea [Cajanus cajan (L.) Millspaugh]. BMC Plant Biol. 11: 17.
- Elma, M.J., Salentijn, E.M. J., Pereira, A., Angenent, G.C., van der Linden, G.C., Krens, F., Smulders, M.J. M., and Vosman, B. 2007. Plant translational genomics: from model species to crops. Mol. Breeding 20: 1–13.
- Eskandari, M., Cober, E.R., and Rajcan, I. 2013. Using the candidate gene approach for detecting genes underlying seed oil concentration and yield in soybean. Theor. Appl. Genet. 126: 1839–1850.
- Fall, A.L., Byrne, P.F., Jung, G., Coyne, D.P., Brick, M.A., and Schwartz, H.F. 2001. Detection and mapping of a major locus for fusarium wilt resistance in common bean. Crop Sci. 41: 1494–1498.
- Fedoruk, M.J., Vandenberg, A., and Bett, K.E. 2013. QTL analysis of seed quality characteristics in lentil (Lens culinaris ssp. culinaris Medik.) using SNP markers. Plant Gen. DOI: 10.3835/plantgenome2013.05.0012.
- Foncéka, D., Hodo-Abalo, T., Rivallan, R., Faye, I., Sall, M.N., Ndoye, O., Fávero, A.P., Bertioli, D.J., Glaszmann, J.C., Courtois, B., and Rami, J.F. 2009. Genetic mapping of wild introgressions into cultivated peanut: a way toward enlarging the genetic basis of a recent allotetraploid. BMC Plant Biol. 9: 103.
- Fondevilla, S., Fernández-Aparicio, M., Satovic, Z., Emeran, A.A., Torres, A.M., Moreno, M.T., and Rubiales, D. 2010. Identification of quantitative trait loci for specific mechanisms of resistance to Orobanche crenata Forsk. in pea (Pisum sativum L.). Mol. Breeding 25: 259–272.
- Fondevilla, S., Satovic, Z., Rubiales, D., Moreno, M.T., and Torres, A.M. 2008. Mapping of quantitative trait loci for resistance to Mycosphaerella pinodes in Pisum sativum subsp. syriacum. Mol. Breeding 21: 439–454.
- Fondevilla, S., Martín-Sanz. A., Satovic. Z., Fernández-Romero, M.D., Rubiales, D., and Caminero, C. 2012. Identification of quantitative trait loci involved in resistance to Pseudomonas syringae pv. syringae in pea (Pisum sativum L.). Euphytica 186: 805–812.
- Fondevilla, S., Cubero, J.I., and Rubiales, D. 2011. Confirmation that the Er3 gene, conferring resistance to Erysiphe pisi in pea, is a different gene from er1 and er2 genes. Plant Breeding 130: 281–282.
- Frei, A., Blair, M.W., Cardona, C., Beebe, S.E., Gu, H., and Dorn, S. 2005. QTL mapping of resistance to Thrips palmi karny in common bean. Crop Sci. 45: 379–387.
- Funatstik, H., Kawagucru, K., Matsuba, S., Sato, Y., and Ishimoto, M. 2005. Mapping of QTL associated with chilling tolerance during reproductive growth in soybean. Theor. Appl. Genet. 111: 851–861.
- Gale, M.D. and Devos, K.M. 1998. Comparative genetics in the grasses. Proc. Natl. Acad. Sci. U S A 95: 1971–1974.
- Galeano, C.H., Fernandez, A.C., Franco-Herrera, N., Cichy, K.A., McClean, P.E., Vanderleyden, J., and Blair, M.W. 2011. Saturation of an intra-gene pool linkage map: towards a unified consensus linkage map for fine mapping and synteny analysis in common bean. PLoS ONE 6: e28135.
- Ganapathy, K.N., Byre Gowda, M., Venkatesha, S.C., Ramachandra, R., Gnanesh, B.N., Gomashe, S.S., Babu, P., Girish, G., Prasad, P.S., Veerakumar, G.N., and Patil, J.V. 2009. Identification of AFLP markers linked to sterility mosaic disease in pigeonpea (Cajanus cajan) (L.) Millsp. Int. J. Integr. Biol. 7: 145–149.
- Garg, R., Patel, R.K., Jhanwar, S., Priya, P., Bhattacharjee, A., Yadav, G., Bhatia, S., Chattopadhyay, D., Tyagi, A.K., and Jain, M. 2011b. Gene discovery and tissue-specific transcriptome analysis in chickpea with massively parallel pyrosequencing and web resource development. Plant Physiol. 156: 1661–1678.
- Garg, R., Patel, R.K., Tyagi, A.K., and Jain, M. 2011a. De novo assembly of chickpea transcriptome using short reads for gene discovery and marker identification. DNA Res. 18: 53–63.
- Garzón, L.N., Ligarreto, G.A., and Blair, M.W. 2008. Molecular marker-assisted backcrossing of anthracnose resistance into Andean climbing beans (Phaseolus vulgaris L.). Crop Sci. 48: 562–570.
- Gaur, P.M., Jukanti, A.K., and Varshney, R.K. 2012b. Impact of genomic technologies on chickpea breeding strategies. Agronomy 2: 199–221.
- Gaur, R., Azam, S., Jeena, S., Khan, A.W., Choudhary, S., Jain, M., Yadav, G., Tyagi, A.K., Chattopadhyay, D., and Bhatia, S. 2012a. High-throughput SNP discovery and genotyping for constructing a saturated linkage map of chickpea (Cicer arietinum L.). DNA Res. 19: 357–373.
- Gautami, B., Fonceka, D., Pandey, M.K., Morezsohn, M.C., Sujay, V., Qin, H., Hong, Y., Faye, I., Chen, X., BhanuPrakash, A., Shah, T.M., Gowda, M,V. C., Nigam, S.N., Liang, X., Hoisington, D.A., Guo, B., Bertioli, D.J., Rami, J., and Varshney, R.K. 2012a. An international reference consensus genetic map with 897 marker loci based on 11 mapping populations for tetraploid groundnut (Arachis hypogaea L.). PLoS ONE 7: e41213.
- Gautami, B., Pandey, M.K., Vadez, V., Nigam, S.N., Ratnakumar, P., Krishnamurthy, L., Radhakrishnan, T., Gowda, M.V. C., Narasu, M.L., Hoisington, D.A., Knapp, S.J., and Varshney, R.K. 2012b. QTL analysis and consensus genetic map for drought tolerance traits based on three RIL populations of cultivated groundnut (Arachis hypogaea L.). Mol. Breeding 32: 757–772.
- Githiri, S.M., Watanabe, S., Harada, K., and Takahashi, R. 2006. QTL analysis of flooding tolerance in soybean at an early vegetative growth stage. Plant Breeding 125: 613–618.
- Githiri, S.M., Yang, D., Khan, N.A., Xu, D., Komatsuda, T., and Takahashi, R. 2007. QTL analysis of low temperature induced browning in soybean seed coats. J. Hered. 98: 360–366.
- Gnanesh, B.N., Bohra, A., Sharma, M., Byregowda, M., Pande, S., Wesley, V., Saxena, R.K., Saxena, K.B., KaviKishore P.B., and Varshney, R.K. 2011. Genetic mapping and quantitative trait locus analysis of resistance to sterility mosaic disease in pigeonpea [Cajanus cajan (L.) Millsp.]. Field Crops Res. 123: 53–61.
- Goff, S.A., Ricke, D., Lan, T.H., Presting, G., Wang, R., Dunn, M., Glazebrook, J., Sessions, A., Oeller, P., Varma, H., Hadley, D., Hutchison, D., Martin, C., Katagiri, F., Lange, B.M., Moughamer, T., Xia, Y., Budworth, P., Zhong, J., Miguel, T., Paszkowski, U., Zhang, S., Colbert, M., Sun, W.L., Chen, L., Cooper, B., Park, S., Wood, T.C., Mao, L., Quail, P., Wing, R., Dean, R., Yu, Y., Zharkikh, A., Shen, R., Sahasrabudhe, S., Thomas, A., Cannings, R., Gutin, A., Pruss, D., Reid, J., Tavtigian, S., Mitchell, J., Eldredge, G., Scholl, T., Miller, R.M., Bhatnagar, S., Adey, N., Rubano, T., Tusneem, N., Robinson, R., Feldhaus, J., Macalma, T., Oliphant, A., and Briggs, S. 2002. A draft sequence of the rice genome (Oryza sativa L. ssp. japonica). Science 296: 92–100.
- Gomez-Roldan, V., Fermas, S., Brewer, P.B., Puech-Pagès, V., Dun, E.A., Pillot, J., Letisse, F., Matusova, R., Danoun, S., Portais, J., Bouwmeester, H., Bécard, G., Beveridge, C.A., Rameau, C., and Rochange, S.F. 2008. Strigolactone inhibition of shoot branching. Nature 455: 189–194.
- Govindarajulu, M., Kim, S.Y., Libault, M., Berg, R.H., Tanaka, K., Stacey, G., and Taylor, C.G. 2009. P GS52 ecto-apyrase plays a critical role during soybean nodulation. Plant Physiol. 149: 994–1004.
- Gowda, S.J. M., Radhika, P., Kadoo, N.Y., Mhase, L.B., and Gupta, V. 2009. Molecular mapping of wilt resistance genes in chickpea. Mol. Breeding 24: 177–183.
- Grenier, C., Chatel, M.H., Ospina, Y., Cao, T., Guimaraes, E.P., Martinez, C.P., Tohme, J., Courtois, B., and Ahmadi, N. 2012. Population improvement through recurrent selection in rice. Prospects for maker assisted recurrent selection and genome-wide selection. In: Plant and Animal Genome Conference XX, January 14–18, 2012, San Diego, U S A. Abstract no. W011.
- Guimarães, P., Brasileiro, A., Morgante, C., Martins, A., Pappas, G., Silva, O.B. Jr., Togawa, R., Leal-Bertioli, S.C., Araujo, A.C., Moretzsohn, M.C., and Bertioli, D.J. 2012. Global transcriptome analysis of two wild relatives of peanut under drought and fungi infection. BMC Genomics 13: 387.
- Gujaria, N., Kumar, A., Dauthal, P., Dubey, A., Hiremath, P.J., Bhanu Prakash A., Farmer, A., Bhide, M., Shah, T., Gaur, P.M., Upadhyaya, H.D., Bhatia, S., Cook, D.R., May, G.D., and Varshney, R.K. 2011. Development and use of genic molecular markers (GMMs) for construction of a transcript map of chickpea (Cicer arietinum L.). Theor. Appl. Genet. 122: 1577–1589.
- Guo, B., Sleper, D.A., Lu, P., Shannon, J.G., Nguyen, H.T., and Arelli, P.R. 2006. QTLsassociated with resistance to soybean cyst nematode in soybean: meta-analysis of QTL locations. Crop Sci. 46: 595–602.
- Guo, B., Sleper, D.A., Arelli, P.R., Shannon, J.G., and Nguyen, H.T. 2005. Identification of QTLs associated with resistance to soybean cyst nematode races 2, 3 and 5 in soybean PI 90763. Theor. Appl. Genet. 111: 965–971.
- Guo, X., Wang, D., Gordon, S.G., Helliwell, E., Smith, T., Berry, S.A., Martin, S.K., and Dorrance, A.E. 2008. Genetic Mapping of QTLs Underlying Partial Resistance to Sclerotinia sclerotiorum in Soybean PI 391589A and PI 391589B. Crop Sci. 48: 1129–1139.
- Guo, Y., Khanal, S., Tang, S., Bowers, J.E., Heesacker, A.F., Khalilian, N., Nagy, E.D., Zhang, D., Taylor, C.A., Stalker, H.T., Ozias-Akins, P., and Knapp, S.J. 2012. Comparative mapping in intraspecific populations uncovers a high degree of macrosynteny between A- and B-genome diploid species of peanut. BMC Genomics 13: 608.
- Gupta, D., Taylor, P.W. J., Inder, P., Phan, H.T. T., Ellwood, S.R., Mathur, P.N., Sarker, A., and Ford, R. 2012b. Integration of EST-SSR markers of Medicago truncatula into intraspecific linkage map of lentil and identification of QTL conferring resistance to ascochyta blight at seedling and pod stages. Mol. Breeding 30: 429–439.
- Gupta, H.S., Agrawal, P.K., Mahajan, V., Bisht, G.S., Kumar, A., Verma, P., Srivastava, A., Saha, S., Babu, R., Pant, M.C., and Mani, V.P. 2009. Quality protein maize for nutritional security: rapid development of short duration hybrids through molecular marker assisted breeding. Curr. Sci. 96: 230–237.
- Gupta, H.S., Raman, B., Agrawal, P.K., Mahajan, V., Hossain, F., and Thirunavukkarasu, N. 2013. Accelerated development of quality protein maize hybrid through marker-assisted introgression of opaque-2 allele. Plant Breeding 132: 77–82.
- Gupta, M., Verma, B., Kumar, N., Chahota, R.K., Rathour, R., Sharma, S.K., Bhatia, S., and Sharma, T.R. 2012a. Construction of intersubspecific molecular genetic map of lentil based on ISSR, RAPD and SSR markers. J. Genet. 91: 279–287.
- Gupta, P.K., Kumar, J., Mir, R.R., and Kumar, A. 2009. Marker-assisted selection as a component of conventional plant breeding. Plant Breed. Rev. 33: 145–217.
- Gutiérrez, N., Palomino, C., Satovic, Z., Ruiz-Rodríguez, M.Z., Vitale, S., Gutiérrez, M.V., Rubiales, D., Kharrat, M., Amri, M., Emeran, A.A., Cubero, J.I., Atienza, S.G., Torres, A.M., and Avila, C.M. 2013. QTLs for Orobanche spp. resistance in faba bean: identification and validation across different environments. Mol. Breeding 32: 909–922.
- Guzmán-Maldonado, S.H., Martínez, O., Acosta-Gallegos, J.A., Guevara-Lara, F., and Paredes-López, O. 2003. Putative quantitative trait loci for physical and chemical components of common bean. Crop Sci. 43: 1029–1035.
- Hamon, C., Baranger, A., Coyne, C.J., McGee, R.J., Le Goff, I., L’Anthoene, V., Esnault, R., Rivière, J.P., Klein, A., Mangin, P., McPhee, K.E., Roux-Duparque, M., Porter, L., Miteul, H., Lesné, A., Morin, G., Onfroy, C., Moussart, A., Tivoli, B., Delourme, R., and Pilet-Nayel, M.L. 2011. New consistent QTL in pea associated with partial resistance to Aphanomyces euteiches in multiple French and American environments. Theor. Appl. Genet. 123: 261–281.
- Hamon, C., Coyne, C.J., McGee, R.J., Lesné, A., Esnault, R., Mangin, P., Hervé, M., Goff, I.L., Deniot, G., Roux-Duparque, M., Morin, G., McPhee, K.E., Delourme, R., Baranger, A., and Pilet-Nayel, M.L. 2013. QTL meta-analysis provides a comprehensive view of loci controlling partial resistance to Aphanomyces euteiches in four sources of resistance in pea. BMC Plant Biol. 13: 45.
- Hamwieh, A., Udapa, S.M., Choumane, W., Sarker, A., Dreyer, F., Jung, C., and Baum M. 2005. A genetic linkage map of lentil based on microsatellite and AFLP markers and localization of fusarium vascular wilt resistance. Theor. Appl. Genet. 110: 669–677.
- Han, Y., Teng, W., Yu, K., Poysa, V., Anderson, T., Qiu, L., Lightfoot, D.A., and Li. W. 2008. Mapping QTL tolerance to Phytophthora root rot in soybean using microsatellite and RAPD/SCAR derived markers. Euphytica 162: 231–239.
- Hanai, L.R., Santini, L., Aranha, L.E. C., Pelegrinelli, M.H. F., Gepts, P., Tsai, S.M., and Carneiro, M.L. 2010. Extension of the core map of common bean with EST-SSR, RGA, AFLP, and putative functional markers. Mol. Breeding 25: 25–45.
- Handberg, K. and Stougaard, J. 1992. Lotus japonicus, an autogamous, diploid legume species for classical and molecular genetics. Plant J. 2: 487–496.
- Hecht, V., Foucher, F., Ferrándiz, C., Macknight, R., Navarro, C., Morin, J., Vardy, M.E., Ellis, N., Beltrán, J.P., Rameau, C., and Weller, J.L. 2005. Conservation of Arabidopsis flowering genes in model legumes. Plant Physiol. 137: 1420–1434.
- Hecht, V., Laurie, R.E., Vander Schoor, J.K., Ridge, S., Knowles, C.L., Chee Liew, L., Sussmilch, F.C., Murfet, I.C., Macknight, R.C., and Weller, J.L. 2011. The pea GIGAS gene is a flowering locus T homolog necessary for graft-transmissible specification of flowering but not for responsiveness to photoperiod. Plant Cell 23: 147–161.
- Heffner, E.L., Sorrells, M.E., and Jannink, J.L. 2009. Genomic selection for crop improvement. Crop Sci. 49: 1–12.
- Hernández-Delgado, S., Reyes-Valdés, M.H., Rosales-Serna, R., and Mayek-Pérez, N. 2009. Molecular markers associated with resistance to Macrophominaphaseolina (Tassi) Goid. in common bean. J. Plant Pathol. 91: 163–170.
- Hiremath, P.J., Farmer, A., Cannon, S.B., Woodward, J., Kudapa, H., Tuteja, R., Kumar, A., Bhanuprakash, A., Mulaosmanovic, B., Gujaria, N., Laxmanan, K., Pooran, M.G., Polavarapu, K.K., Shah, T., Srinivasan, R., Lohse, M., Xiao, Y., Christopher, D.T., Cook, D.R., May, G.D., and Varshney, R.K. 2011. Large-scale transcriptome analysis in chickpea (Cicer arietinum L.), an orphan legume crop of the semi-arid tropics of Asia and Africa. Plant Biotechnol. J. 9: 922–931.
- Hiremath, P.J., Kumar, A., Penmetsa, R.V., Farmer, A., Schlueter, J.A., Chamarthi, S.K., Whaley, A.M., Carrasquilla-Garcia, N., Gaur, P.M., Upadhyaya H.D., KaviKishor, P.B., Shah, T.M., Cook, D.R., and Varshney, R.K. 2012. Large-scale development of cost-effective SNP marker assays for diversity assessment and genetic mapping in chickpea and comparative mapping in legumes. Plant Biotechnol. J. 10: 716–732.
- Hoen, P.A. C., Ariyurek, Y., Thygesen, H.H., Rolf, E.V., Vossen, H.A. M., de Menezes, R.X., Boer, J.M., van Ommen, G.B., and den Dunnen, J.T. 2008. Deep sequencing-based expression analysis shows major advances in robustness, resolution and inter-lab portability over five microarray platforms. Nucleic Acids Res. 36: e141.
- Hofer, J., Turner, L., Moreau, C., Ambrose, M., Isaac, P., Butcher, S., Weller, J., Dupin, A., Dalmais, M., Le Signor, C., Bendahmane, A., and Ellis, N. 2009. Tendril-less regulates tendril formation in pea leaves. Plant Cell 21: 420–428.
- Hoshino, Y. 1915. On the inheritance of the flowering time in peas and rice. Jour. Col. Agr. Tohoku Imp. Univ. 6: 229–288.
- Hossain, S., Ford, R., McNeil, D., Pittock, C., and Panozzo, J.F. 2011. Quantitative trait loci analysis of seed coat color components for selective breeding in chickpea (Cicer arietinum L.). Canadian J. Plant Sci. 91: 49–55.
- Huynh, T.T., Bastien, M., Iquira, E., Turcotte, P., and Belzile, F. 2010. Identification of QTLs associated with partial resistance to white mold in soybean using field-based inoculation. Crop Sci. 50: 969–979.
- Hyten, D., Song, Q., Fickus, E., Quigley, C., Lim, J.S., Choi, I.Y., Hwang, E.Y., Pastor-Corrales, M., and Cregan, P. 2010. High-throughput SNP discovery and assay development in common bean. BMC Genomics 11: 475.
- Imelfort, M., Duran, C., Batley, J., and Edwards, D. 2009. Discovering genetic polymorphisms in next generation sequencing data. Plant Biotechnol. 7: 312–317.
- Iruela, M., Castro, P., Rubio, J., Cubero, J.I., Jacinto, C., Millán, T., and Gil, J. 2007. Validation of a QTL for resistance to ascochyta blight linked to resistance to fusarium wilt race 5 in chickpea (Cicer arietinum L.). In: Ascochyta blights of grain legumes. Springer Netherlands. pp. 29–37.
- Iruela, M., Rubio, J., Barro, F., Cubero, J.I., Millan, T., and Gill, J. 2006. Detection of two quantitative trait loci for resistance to ascochyta blight in an intra-specific cross of chickpea (Cicer arietinum L.): development of SCAR markers associated with resistance. Theor. Appl. Genet. 112: 278–287.
- Irzykowska, L. and Wolko, B. 2004. Interval mapping of QTLs controlling yield-related traits and seed protein content in Pisum sativum. J. Appl. Genet. 45: 297–306.
- Jackson, S.A., Iwata, A., Lee, S.H., Schmutz, J., and Shoemaker, R. 2011. Sequencing crop genomes: approaches and applications. New Phytol. 191: 915–925.
- Jaillon, O., Aury, J., Noel, B., Policriti, A., Clepet, C., Casagrande, A., Choisne, N., Aubourg, S., Vitulo, N., Jubin, C., Vezzi, A., Legeai, F., Hugueney, P., Dasilva, C., Horner, D., Mica, E., Jublot, D., Poulain, J., Bruyère, C., Billault, A., Segurens, B., Gouyvenoux, M., Ugarte, E., Cattonaro, F., Anthouard, V., Vico, V., Del Fabbro, C., Alaux, M., Di Gaspero, G., Dumas, V., Felice, N., Paillard, S., Juman, I., Moroldo, M., Scalabrin, S., Canaguier, A., Le Clainche, I., Malacrida, G., Durand, E., Pesole, G., Laucou, V., Chatelet, P., Merdinoglu, D., Delledonne, M., Pezzotti, M., Lecharny, A., Scarpelli, C., Artiguenave, F., Pè, M.E., Valle, G., Morgante, M., Caboche, M., Adam-Blondon, A.F., Weissenbach, J., Quétier, F., and Wincker, P. 2007. The grapevine genome sequence suggests ancestral hexaploidization in major angiosperm phyla. Nature 449: 463–467.
- Jannink, J.L., Lorenz, A.J., and Iwata, H. 2010. Genomic selection in plant breeding: from theory to practice. Brief. Funct. Genomics 9: 166–177.
- Jhanwar, S., Priya, P., Garg, R., Parida, S.K., Tyagi, A.K., and Jain, M. 2012. Transcriptome sequencing of wild chickpea as a rich resource for marker development. Plant Biotechnol. J. 10: 690–702.
- Jia, Y., Gu, H., Wang, X., Chen, Q., Shi, S., Zhang, J., Ma, L., Zhang, H., and Ma, H. 2012. Molecular cloning and characterization of an F-box family gene CarF-box1 from chickpea (Cicer arietinum L.). Mol. Biol. Rep. 39: 2337–2345.
- Johnson, E., Miklas, P.N., Stavely, J.R., and Martinez-Cruzado, J.C. 1995. Coupling- and repulsion-phase RAPDs for marker-assisted selection of PI 181996 rust resistance in common bean. Theor. Appl. Genet. 90: 659–664.
- Johnson, W.C., Guzman, P., Mandala, D., Mkandawire, A.B. C., Temple, S., Gilbertson, R.L., and Gepts, P. 1997. Molecular tagging of the bc-3 gene for introgression into Andean common bean. Crop Sci. 37: 248–254.
- Jung, G., Ariyarathne, H.M., Coyne, D.P., and Nienhuis, J. 2003. Mapping QTL for bacterial brown spot resistance under natural infection in field and seeding stem inoculation in growth chamber in common bean. Crop Sci. 43: 350–357.
- Kahraman, A., Demirel, U., Ozden, M., and Muehlbauer, F.J. 2010. Mapping of QTLs for leaf area and the association with winter hardiness in fall-sown lentil. Afr. J. Biotechnol. 9: 8515–8519.
- Kalavacharla, V., Liu, Z., Meyers, B.C., Thimmapuram, J., and Melmaiee, K. 2011. Identification and analysis of common bean (Phaseolus vulgaris L.) transcriptomes by massively parallel pyrosequencing. BMC Plant Biol. 11: 135.
- Kassa, M.T., Penmetsa, R.V., Carrasquilla-Garcia, N., Sarma, B.K., Datta, S., Upadhyaya, H.D., Varshney, R.K., Wettberg, E.J. B., and Cook, D.R. 2012. Genetic patterns of domestication in pigeonpea (Cajanus cajan (L.) Millsp.) and wild Cajanus relatives. PLoS ONE 7: e39563.
- Kassem, M.A., Shultz, J., Meksem, K., Cho, Y., Wood, A.J., Iqbal, M.J., and Lightfoot, D.A. 2006. An updated EssexForrest linkage map and first composite interval map of QTL underlying six soybean traits. Theor. Appl. Genet. 113: 1115–1126.
- Kazi, S., Shultz, J., Afzal, J., Johnson, J., Njiti, V.N., and Lightfoot, D.A. 2008. Separate loci underlie resistance to root infection and leaf scorch during soybean sudden death syndrome. Theor. Appl. Genet. 116: 967–977.
- Khan, N.A., Githiri, S.M., Benitez, E.R., Abe, J., Kawasaki, S., Hayashi, T., and Takahashi, R. 2008. QTL analysis of cleistogamy in soybean. Theor. Appl. Genet. 117: 479–487.
- Khanh, T.D., Anh, T.Q., Buu, B.C., and Xuan, T.D. 2013. Applying molecular breeding to improve soybean rust resistance in Vietnamese elite soybean. American J. Plant Sci. DOI:10.4236/ajps.2013.41001.
- Khedikar, Y.P., Gowda, M.V. C., Sarvamangala, C., Patgar, K.V., Upadhyaya, H.D., and Varshney, R.K. 2010. A QTL study on late leaf spot and rust revealed one major QTL for molecular breeding for rust resistance in groundnut (Arachis hypogaea L.). Theor. Appl. Genet. 121: 971–984.
- Kim, K.Y., Park, S.W., Chung, Y.S., Chung, C.H., Kim, J.I., and Lee, J.H. 2004. Molecular cloning of low-temperature-inducible ribosomal proteins from soybean. J. Exp. Bot. 55: 1153–1155.
- Kim, M.S., Park, M.J., Jeong W.H., Nam, K.C., and Chung, J.I. 2006. SSR marker tightly linked to the Ti locus in soybean [Glycine max (L.) Merr.]. Euphytica 152: 361–366.
- Kim, M.Y., Shin, J.H., Kang, Y.J., Shim, S.R., and Lee, S.H. 2012. Divergence of flowering genes in soybean. J. Biosci. 37: 857–870.
- Klein, A., Houtin, H., Rond, C., Marget, P., Jacquin, F., Boucherot, K., Huart, M., Riviere, N., Boutet, G., Lejeune-Henaut, I., and Burstin, J. 2014. QTL analysis of frost damage in pea suggests different mechanisms involved in frost tolerance. Theor. Appl. Genet. 127: 1319–1330.
- Koebner, R.M. and Varshney, R.K. 2006. Development and application of genomic models for large-crop plant genomes. In: Model Plants and Crop Improvement. pp. 1–10. Varshney, R.K. and Koebner, R.M., Eds., CRC Press, New York.
- Koilkonda, P., Sato, S., Tabata, S., Shirasawa, K., Hirakawa, H., Sakai, H., Sasamoto, S., Watanabe, A., Wada, T., Kishida, Y., Tsuruoka, H., Fujishiro, T., Yamada, M., Kohara, M., Suzuki, S., Hasegawa, M., Kiyoshima, H., and Isobe, S. 2012. Large-scale development of expressed sequence tag-derived simple sequence repeat markers and diversity analysis in Arachis spp. Mol. Breeding 30: 125–138.
- Kotresh, H., Fakrudin, B., Punnuri, S., Rajkumar, B., Thudi, M., Paramesh, H., Lohithswa H., and Kuruvinashetti, M.S. 2006. Identification of two RAPD markers genetically linked to a recessive allele of a Fusarium wilt resistance gene in pigeonpea (Cajanus cajan (L.) Millsp.). Euphytica 149: 113–120.
- Kottapalli, P., Gaur, P.M., Katiyar, S.K., Crouch, J.H., Buhariwalla, H.K., Pande, S., and Gali, K.K. 2009. Mapping and validation of QTLs for resistance to an Indian isolate of Ascochyta blight pathogen in chickpea. Euphytica 165: 79–88.
- Kudapa, H., Azam, S., Sharpe, A.G., Tar’an, B., Li, R., Deonovic, B., Cameron, C., Farmer, A.D., and Varshney, R.K. 2014. Comprehensive transcriptome assembly of chickpea (Cicer arietinum) using Sanger and next generation sequencing platforms: development and applications. PLoS ONE 9: e86039.
- Kudapa, H., Bharti, A.K., Cannon, S.B., Farmer, A.D., Mulaosmanovic, B., Kramer, R., Bohra, A., Weeks, N.T., Crow, J.A., Tuteja, R., Shah, T., Dutta, S., Gupta, D.K., Singh. A., Gaikwad, K., Sharma, T.R., May, G.D., Singh, N.K., and Varshney, R.K. 2012. A comprehensive transcriptome assembly of pigeonpea (Cajanus cajan L.) using Sanger and second-generation sequencing platforms. Mol. Plant. 5: 1020–1028.
- Kulwal, P.L., Thudi, M., and Varshney, R.K. 2011. Genomics interventions in crop breeding for sustainable agriculture. In: Encyclopedia of sustainability science and technology, Meyers, R.A., Eds., Springer, New York. DOI:10.1007/978-1-4419-0851-3.
- Kumawat, G., Raje, R.S., Bhutani, S., Pal, J.K., Mithra, S.V. C. R., Gaikwad, K., Sharma, T.R., and Singh, N.K. 2012. Molecular mapping of QTLs for plant type and earliness traits in pigeonpea (Cajanus cajan L. Millsp.). BMC Genet. 13: 84.
- Lam, H.M., Xu, X., Lui, X., Chen, W., Yang, G., Wong, F.L., Li, M.W., He, W., Qin, N., Wang, B., Li, J., Jian, M., Wang, J., Shao, G., Wang, J., Sun, S.S., and Zhang, G. 2010. Re-sequencing of 31 wild and cultivated soybean genomes identifies patterns of genetic diversity and selection. Nat Gent. 42: 1053–1059.
- Landau-Ellis, D. and Pantalone, V.R. 2009. Marker-assisted backcrossing to incorporate two low phytate alleles into the Tennessee soybean cultivar 5601T. In: Induced Plant Mutations in the Genomics Era. pp. 316–318. Food and Agriculture Organization of the United Nations, Rome.
- Larsen, R.C. and Miklas, P.N. 2004. Generation and molecular mapping of a SCAR marker linked with the Bct gene for resistance to beet curly top virus in common bean. Phytopathol. 94: 320–325.
- Leal-Bertioli, S.C. M., José, A.C. V. F., Alves-Freitas, D.M. T., Moretzsohn, M.C., Guimarães, P.M., Nielen, S., Vidigal, B.S., Pereira, R.W., Pike, J., Fávero, A.P., Parniske, M., Varshney, R.K., and Bertioli, D.J. 2009. Identification of candidate genome regions controlling disease resistance in Arachis. BMC Plant Biol. 9: 112.
- Lee, J.D., Shannon, J.G., Vuong, T.D., and Nguyen, H.T. 2009. Inheritance of salt tolerance in wild soybean (Glycine sojaSieb. and Zucc.) accession PI 483463. J. Hered. 100: 798–801.
- Lei, Z., Dai, X., Watson, B.S., Zhao, P.X., and Sumner, L.W. 2011. A legume specific protein database (LegProt) improves the number of identified peptides, confidence scores and overall protein identification success rates for legume proteomics. Phytochemistry 72: 1020–1027.
- Leonforte, A., Sudheesh, S., Cogan, N.O., Salisbury, P.A., Nicolas, M.E., Materne, M., Forster, J.W., and Kaur, S. 2013. SNP marker discovery, linkage map construction and identification of QTLs for enhanced salinity tolerance in field pea (Pisum sativum L.). BMC Plant Biol. 13:161.
- Le-Signor, C., Savois, V., Aubert, G., Verdier, J., Nicolas, M., Pagny, G., Moussy, F., Sanchez, M., Baker, D., Clarke, J., and Thompson, R. 2009. Optimizing TILLING populations for reverse genetics in Medicago truncatula. Plant Biotechnol. J. 7: 430–441.
- Li, X.P., Tian, A.G., Luo, G.Z., Gong, Z.Z., Zhang, J.S., and Chen, S.Y. 2005. Soybean DRE-binding transcription factors that are responsive to abiotic stresses. Theor. Appl. Genet. 110: 1355–1362.
- Li, X., Han, Y., Teng, W., Zhang, S., Yu, K., Poysa, V., Anderson, T., Ding, J., and Li, W. 2010. Pyramided QTL underlying tolerance to Phytophthora root rot in mega-environments from soybean cultivars Conrad and Hefeng 25. Theor. Appl. Genet. 121: 651–658.
- Li, L., Wang, W.Q., Wu, C.X., Han, T.F., and Hou, W.S. 2012. Construction of two suppression subtractive hybridization libraries and identification of salt-induced genes in soybean. J. Integr. Agri. 11: 1075–1085.
- Liang, X., Zhou, G., Hong, Y., Chen, X., Liu, H., and Li, S. 2009. Overview of research progress on peanut (Arachis hypogaea L.) host resistance to aflatoxin contamination and genomics at the Guangdong Academy of Agricultural Sciences. Peanut Sci. 36: 29–34.
- Liao, Y., Zou, H.F., Wei, W., Hao, Y.N., Tian, A.G., Huang, J., Liu, Y.F., Zhang, J.S., and Chen, S.Y. 2008. Soybean GmbZIP44, GmbZIP62 and GmbZIP78 genes function as negative regulator of ABA signaling and confer salt and freezing tolerance in transgenic Arabidopsis. Planta 228: 225–240.
- Libault, M., Farmer, A., Joshi, T., Takahashi, K., Langley, R.J., Franklin, L.D., He, J., Xu, D., May, G., and Stacey, G. 2010. An integrated transcriptome atlas of the crop model Glycine max, and its use in comparative analyses in plants. Plant J. 63: 86–99.
- Lichtenzveig, J., Bonfil, D.J., Zhang, H.B., Shtienberg, D., and Abbo, S. 2006. Mapping quantitative trait loci in chickpea associated with time to flowering and resistance to Didymella rabiei the causal agent of Ascochyta blight. Theor. Appl. Genet. 113: 1357–1369.
- Li, H., Liu, H., Han, Y., Wu, X., Teng, W., Liu, G., and Li, W. 2010. Identification of QTL underlying vitamin E contents in soybean seed among multiple environments. Theor. Appl. Genet. 120: 1405–1413.
- Liu, B. and Abe, J. 2010. QTL Mapping for photoperiod insensitivity of a Japanese soybean landrace sakamotowase. J. Hered. 2: 251–256.
- Liu, B., Fujita, T., Yan, Z.H., Sakamoto, S., Xu, D., and Abe, J. 2007. QTL mapping of domestication-related traits in soybean (Glycine max). Ann. Bot-London. 100: 1027–1038.
- Liu, S., Yu, K., and Park, S.J. 2005. AFLP markers are tightly linked to the major QTL for CBB resistance in HR67. Bean Improv. Coop. 48: 102–103.
- López, C.E., Acosta, I.F., Jara, C., Pedraza, F., Gaitán-Solís, E., Gallego, G., Beebe, S., and Tohme, J. 2003. Identifying resistance gene analogs associated with resistances to different pathogens in common bean. Phytopathol. 93: 88–95.
- Lorenc, M.T., Hayashi, S., Stiller, J., Lee, H., Manoli, S., Ruperao, P., Visendi, P., Berkman, P.J., Lai, K., Batley, J., and Edwards. D. 2012. Discovery of single nucleotide polymorphisms in complex genomes using SGSautoSNP. Biology 1: 370–382.
- Loridon, K., McPhee, K., Morin, J., Dubreuil, P., Pilet-Nayel, M.L., Aubert, G., Rameau, C., Baranger, A., Coyne, C., Lejeune-Hènaut, I., and Burstinet, J. 2005. Microsatellite marker polymorphism and mapping in pea (Pisum sativum L.). Theor. Appl. Genet. 111: 1022–1031.
- Lucas, M.R., Diop, N.N., Wanamaker, S., Ehlers, J.D., Roberts, P.A., and Close, T.J. 2011. Cowpea soybean synteny clarified through an improved genetic map. Plant Gen. 4: 218–219.
- Mach, J. 2011. The plot thickens: flowering specification in legumes. Plant Cell 23: 2.
- Madrid, E., Rubiales, D., Moral, A., Moreno, M.T., Millán, T., Gil, J., and Rubio, J. 2008. Mechanism and molecular markers associated with rust resistance in a chickpea interspecific cross (Cicer arietinum× Cicer reticulatum). Eur. J. Plant Pathol. 121: 43–53.
- Mazarei, M., Elling, A.E., Maier, T.R., Puthoff, D.P., and Baum, T.J. 2007. GmEREBP1 is a transcription factor activating defense genes in soybean and Arabidopsis. Mol. Plant-Microbe Interact. 20: 107–119.
- McConnell, M., Mamidi, S., Lee, R., Chikara, S., Rossi, M., Papa, R., and McClean, P. 2010. Syntenic relationships among legumes revealed using a gene-based genetic linkage map of common bean (Phaseolus vulgaris L.). Theor. Appl. Genet. 121: 1103–1116.
- Meng, Q., Zhang, C., Gai, J., and Yu, D. 2007. Molecular cloning, sequence characterization and tissue-specific expression of six NAC-like genes in soybean (Glycine max (L.) Merr.). J. Plant Physiol. 164: 1002–1012.
- Meyer, J.D. F., Silva, D.C. G., Yang, C., Pedley, K.F., Zhang, C., van de Mortel, M., Hill, J.H., Shoemaker, R.C., Abdelnoor, R.V., Whitham, S.A., and Graham, M.A. 2009. Identification and analyses of candidate genes for Rpp4-mediated resistance to Aasian soybean rust in soybean. Plant Physiol. 150: 1295–1307.
- Michael, P.T. and Jackson, S. 2013. The first 50 plant genomes. Plant Gen. DOI: 10.3835/plantgenome2013.03,0001in.
- Mienie, C.M. S., Liebenberg, M.M., Pretorius, Z.A., and Miklas, P.N. 2005. SCAR markers linked to the Phaseolus vulgaris rust resistance gene Ur-13. Theor. Appl. Genet. 111: 972–979.
- Miklas, P.N., Coyne, D.P., Grafton, K.F., Mutlu, N., Reiser, J., Lindgren, D.T., and Singh, S.P. 2003. A major QTL for common bacterial blight resistance derives from the common bean great northern landrace cultivar Montana No. 5. Euphytica 131: 137–146.
- Miklas, P.N., Fourie, D., Trapp, J., Larsen, R.C., Chavarro, C., Blair, M.W., and Gepts, P. 2011. Genetic characterization and molecular mapping Pse-2 gene for resistance to halo blight in common bean. Crop Sci. 51: 2439–2448.
- Miklas, P.N., Fourie, D., Wagner, J., Larsen, R.C., and Mienie, C.M. S. 2009. Tagging and mapping Pse-1 gene for resistance to halo blight in common bean differential cultivar UI-3. Crop Sci. 49: 41–48.
- Miklas, P.N., Hu, J., Grünwald, N.J., and Larsen, K.M. 2006. Potential application of TRAP (Targeted Region Amplified Polymorphism) markers for mapping and tagging disease resistance traits in common bean. Crop Sci. 46: 910–916.
- Miklas, P.N., Stavely, J.R., and Kelly, J.D. 1993. Identification and potential use of a molecular marker for rust resistance in common bean. Theor. Appl. Genet. 85: 745–749.
- Mouradov, A., Cremer, F., and Coupland, G. 2002. Control of flowering time: Interacting pathways as a basis for diversity. Plant Cell 14: S111–S130.
- Muchero, W., Diop, N.N., Bhat, P.R., Fenton, R.D., Wanamaker, S., Pottorff, M., Hearne, H., Cisse, N., Fatokun, C., Ehlers, J.D., Roberts, P.A., and Close, T.J. 2009. A consensus genetic map of cowpea [Vigna unguiculata (L) Walp.] and synteny based on EST-derived SNPs. Proc. Natl. Acad. Sci. U S A 106: 18159–18164.
- Mutlu, N., Miklas, P.N., Steadman, J.R., Vldaver, A.V., Lmdgren, D., ReIser, J., and Pastor-Corrales, M.A. 2005. Registration of pinto bean germplasm line ABCP-8 with resistance to common bacterial blight. Crop Sci. 45: 806.
- Mutlu, N., Urrea, C.A., Mlklas, P.N., Pastor-Corrales, M.A., Steadman, J.R., Lindgren, D.T., Reiser, J., Vidaver, K., and Coyne, D.P. 2008. Registration of common bacterial blight, rust and bean common mosaic resistant great northern common bean germplasm line ABC-Weihing. J. Plant Regist. 2: 120–124.
- Nagy, E., Guo, S., Khanal, S., Taylor, C., Ozias-Akins, P., Stalker, H.T., and Nielsen, N. 2010. Developing a high density molecular map of the A-genome species A. duranensis. In: Proc. American Peanut Research and Education Society (APRES), 12-15 July, Florida, U S A.
- Nakaya, A. and Isobe, S.N. 2012. Will genomic selection be a practical method for plant breeding? Ann. Bot. 110: 1303–1316.
- Narvel, J.M., Walker, D.R., Rector, B.G., All, J.N., Parrott, W.A., and Boerma, H.R. 2001. A retrospective DNA marker assessment of the development of insect resistant soybean. Crop Sci. 41: 1931–1939.
- Nayak, S.N., Zhu, H., Varghese, N., Datta, S., Choi, H.K., Horres, R., Jüngling, R., Singh, J., Kishor, P.B., Sivaramakrishnan, S., Hoisington, D.A., Kahl, G., Winter, P., Cook, D.R., and Varshney, R.K. 2010. Integration of novel SSR and gene-based SNP marker loci in the chickpea genetic map and establishment of new anchor points with Medicago truncatula genome. Theor. Appl. Genet. 120: 1415–1441.
- Neeraja, C.N., Maghirang-Rodriguez, R., Pamplona, A., Heuer, S., Collard, B.C. Y., Septiningsih, E.M., Vergara, G., Sanchez, D., Xu, K., Ismail, A.M., and Mackill, D.J. 2007. A marker-assisted backcross approach for developing submergence-tolerant rice cultivars. Theor. Appl. Genet. 115: 767–776.
- Nichols, D.M., Glover, K.D., Carlson, S.R., Specht, J., and Diers, B.W. 2006. Fine mapping of a seed protein QTL on soybean linkage group I and its correlated effects on agronomic traits. Crop Sci. 46: 834–839.
- Nunes, A.C. S., Vianna, G.R., Cuneo, F., Amaya-Farfán, J., de Capdeville, G., Rech, E.L., and Aragão, F.J. L. 2006. RNAi-mediated silencing of the myo-inositol-1-phosphate synthase gene (GmMIPS1) in transgenic soybean inhibited seed development and reduced phytate content. Planta 224: 125–132.
- Oblessuc, P.R., Baroni, R.M., Gracia, A.A. F., Chioratto, A.F., Carbonell, S.A. M., Camargo, L.E. A., and Benchimol, L.L. 2012. Mapping of angular leaf spot resistance QTL in common bean (Phaseolus vulgaris L.) under different environments. BMC Genet. 13: 50.
- Omoigui, L.O., Kamara, A.Y., Ishiyaku, M.F., and Boukar, O. 2012. Comparative responses of cowpea breeding lines to Striga and Alectra in the dry savannah of northeast Nigeria. Afr. J. Agric. Res. 7: 747–754.
- Oyoo, M.E., Githiri, S.M., Benitez, E.R., and Takahashi, R. 2010. QTL analysis of net-like cracking in soybean seed coats. Breeding Sci. 60: 28–33.
- Pandey, M.K., Feng, S., Culbreath, A., Varshney, R.K., Wang, M.L., Barkley, N.A., Holbrook, C.C., and Guo, B. 2012. Saturation of genetic maps for identification of QTLs controlling biotic resistance, morphological descriptors and oil quality in tetraploid peanut (Arachis hypogaea). In: Proc American Peanut Research and Education Society (APRES), pp. 27, July 9–11, Raleigh, U S A.
- Panthee, D.R., Pantalone, V.R., Sams, C.E., Saxton, A.M., West, D.R., Orf, J.H., and Killam, A.S. 2006. Quantitative trait loci controlling sulfur containing amino acids, methionine and cysteine, in soybean seeds. Theor. Appl. Genet. 112: 546–553.
- Park, S.O., Coyne, D.P., Steadman, J.R., and Skroch, P.W. 2001. Mapping of QTL for resistance to white mold disease in common bean. Crop Sci. 41: 1253–1262.
- Park, S.O., Steadman, J.R., Coyne, D.P., and Crosby, K.M. 2008. Development of a coupling phase SCAR marker linked to the Ur-7 rust resistance gene and its occurrence in diverse common bean lines. Crop Sci. 48: 357–363.
- Peiffer, G.A., King, K.E., Severin, A.J., May, G.D., Cianzio, S.R., Lin, S.F., Lauter, N.C., and Shoemaker, R.C. 2012. Identification of candidate genes underlying an iron efficiency quantitative trait locus in soybean. Plant Physiol. 158: 1745–1754.
- Peleman, J.D. and Voort, J.R. V. D. 2003. Breeding by design. Trends Plant Sci. 8: 330–334.
- Peng, H., Cheng, H.Y., Chen, C., Yu, X.W., Yang, J.N., Gao, W.R., Shi, Q.H., Zhang, H., Li, J.G., and Ma, H. 2009. A NAC transcription factor gene of Chickpea (Cicer arietinum), CarNAC3, is involved in drought stress response and various developmental processes. J. Plant Physiol. 166: 1934–1945.
- Pérez-Vega, E., Pañeda, A., Rodríguez-Suárez, C., Campa, A., Giraldez, R., and Ferreira, J.J. 2010. Mapping of QTLs for morpho-agronomic and seed quality traits in a RIL population of common bean (Phaseolus vulgaris L.). Theor. Appl. Genet. 120: 1367–1380.
- Perry, J.A., Wang, T.L., Welham, T.J., Gardner, S., Pike, J.M., Yoshida, S., and Parniske, M. 2003. A TILLING reverse genetics tool and a web-accessible collection of mutants of the legume Lotus japonicus. Plant Physiol. 131: 866–871.
- Piepho, H.P. 2009. Ridge regression and extensions for genome-wide selection in maize. Crop Sci. 49: 1165–1176.
- Pilet-Nayel, M.L., Muehlbauer, F.J., McGee, R.J., Kraft, J.M., Baranger, A., and Coyne, C.J. 2002. Quantitative trait loci for partial resistance to Aphanomyces root rot in pea. Theor. Appl. Genet. 106: 28–39.
- Prioul, S., Frankewitz, A., Deniot, G., Morin, G., and Baranger, A. 2004. Mapping of quantitative trait loci for partial resistance to Mycosphaerella pinodes in pea (Pisum sativum L.), at the seedling and adult plant stages. Theor. Appl. Genet. 108: 1322–1334.
- Priyanka, B., Sekhar, K., Sunita, T., Reddy, V.D., and Rao, K.V. 2010. Characterization of expressed sequence tags (ESTs) of pigeonpea (Cajanus cajan L.) and functional validation of selected genes for abiotic stress tolerance in Arabidopsis thaliana. Mol. Genet. Genomics 283: 273–287.
- Qi, B., Korir, P., Zhao, T., Yu, D., Chen, S., and Gai, J. 2008. Mapping quantitative trait loci associated with aluminum toxin tolerance in NJRIKY recombinant inbred line population of soybean (Glycine max). J. Integr. Plant Biol. 50: 1089–1095.
- Qin, H., Feng, S., Chen, C., Guo, Y., Knapp, S., Culbreath, A., He, G., Wang, M.L., Zhang, X., Holbrook, C.C., Ozias-Akins, P., and Guo, B. 2012. An integrated genetic linkage map of cultivated peanut (Arachis hypogaea L.) constructed from two RIL populations. Theor. Appl. Genet. 124: 653–664.
- Ragnin, V.A., Sanglard, D.A., de Sousa, T.L. P. O., Moreira, M.A., and de Barros, E.G. 2003. Simultanious transfer of resistance genes for rust, anthracnose, and angular leaf spot to cultivar Perola assisted by molecular markers. Annu. Rev. Bean Improv. Coop. 46: 159–160.
- Rajesh, P.N., Tekeoglu, M., Gupta, V.S., Ranjekar, P.K., and Muehlbauer, F.J. 2002. Molecular mapping and characterization of an RGA locus RGAPtokin1-2171 in chickpea. Euphytica. 128: 427–433.
- Ravi, K., Vadez, V., Isobe, S., Mir, R.R., Guo, Y., Nigam, S.N., Gowda, M.V. C., Radhakrishnan, T., Bertioli, D.J., Knapp, S.J., and Varshney, R.K. 2011. Identification of several small-effect main QTLs and larg number of epistatic QTLs for drought tolerance in groundnut (Arachis hypogaea L.). Theor. Appl. Genet. 122: 1119–1132.
- Reinprecht, Y., Luk‐Labey, S‐Y., Larsen, J., Poysa, V.W., Yu, K., Rajcan, I., Ablett, G.R., and Pauls, K.P. 2009. Molecular basis of the low linolenic acid trait in soybean EMS mutant line RG10. Plant Breeding 128: 253–258.
- Ribaut, J.M. and Ragot, M. 2007. Marker-assisted selection to improve drought adaptation in maize: the backcross approach, perspectives, limitations, and alternatives. J. Exp. Bot. 58: 351–360.
- Ribaut, J.M., Bertioli, D.J., Raatz, B., Roberts, P., Vadez, V., Varshney, R.K., and Diop, N.N. 2012. Innovative approaches to increase tropical legume productivity in Africa and South Asia. In: VI International Conference on Legume Genetics and Genomics (ICLGG), October 2–7, pp.I-GAB01.
- Ribaut, J.M., de Vicente, M.C., and Delannay, X. 2010. Molecular breeding in developing countries: challenges and perspectives. Curr. Opin. Plant Biol. 13: 213–218.
- Rodríguez-Suárez, C., Méndez-Vigo, B., Pañeda, A., Ferreira, J.J., and Giraldez, R. 2007. A genetic linkage map of Phaseolus vulgaris L. and localization of genes for specific resistance to six races of anthracnose (Colletotrichum lindemuthianum). Theor. Appl. Genet. 114: 713–722.
- Rogers, C., Wen, J., Chen, R., and Oldroyd, G. 2009. Deletion-based reverse genetics in Medicago truncatula. Plant Physiol. 151: 1077–1086.
- Roman, B., Torres, A.M., Rubiales, D., Cubero, J.I., and Satovic, Z. 2002. Mapping of quantitative trait loci controlling broomrape (Orobanche crenata Forsk) resistance in faba bean (Vicia faba L.). Genome 45: 1057–1063.
- Roorkiwal, M., Sawargaonkar, S.L., Chitikineni, A., Thudi, M., Saxena, R.K., Upadhyaya, H.D., Vales, M.I., Riera-Lizarazu, O., and Varshney, R.K. 2013. Single nucleotide polymorphism genotyping for breeding and genetics applications in chickpea and pigeonpea using the BeadXpress platform. Plant Gen. DOI: 10.3835/plantgenome2013.05.0017.
- Rubeena, Ford, R., and Taylor, P.W. 2003. Construction of an intraspecific linkage map of lentil (Lens culinaris ssp. culinaris). Theor. Appl. Genet. 107: 910–916.
- Rubeena, Taylor, P.W., Ades, P.K., and Ford, R. 2006. QTL mapping of resistance in lentil (Lens culinaris ssp. culinaris) to Ascochyta blight (Ascochyta lentis). Plant Breeding 125: 506–512.
- Ruben, E., Jamai, A., Afzal, J., Njiti, V.N., Triwitayakorn, K., Iqbal, M.J., Yaegashi, S., Bashir, R., Kazi, S., Arelli, P., Town, C.D., Ishihara, H., Meksem, K., and Lightfoot, D.A. 2006. Genomic analysis of the rhg1 locus: candidate genes that underlie soybean resistance to the cyst nematode. Mol. Gen. Genomics 276: 503–516.
- Sabbavarapu, M.M., Sharma, M., Chamarthi, S.K., Swapna, N., Rathore, A., Thudi, M., Gaur, P.M., Pande, S., Singh, S., Kaur, L., and Varshney, R.K. 2013. Molecular mapping of QTLs for resistance to Fusarium wilt (race 1) and Ascochyta blight in chickpea (Cicer arietinum L.). Euphytica 93: 121–133.
- Saghai-Maroof, M.A., Jeong, S.C., Gunduz, I., Tucker, D.M., Buss, G.R., and Tolm, S.A. 2008. Pyramiding of soybean mosaic virus resistance genes by marker assisted selection. Crop Sci. 48: 517–526.
- Saha, G.C., Sarker, A., Chen, W., Vandemark, G.J., and Muehlbauer, F.J. 2010. Inheritance and linkage map positions of genes conferring resistance to stemphylium blight in lentil. Crop Sci. 50: 1831–1839.
- Salas, P., Oyarzo-Llaipen, J.C., Wang, D., Chase, K., and Mansur, L. 2006. Genetic mapping of seed shape in three populations of recombinant inbred lines of soybean [Glycine max L. Merr.]. Theor. Appl. Genet. 113: 1459–1466.
- Sarvamangala, C., Gowda, M.V. C., and Varshney, R.K. 2011. Identification of quantitative trait loci for protein content, oil content and oil quality for groundnut (Arachis hypogaea L.). Field Crop Res. 122: 49–59.
- Sato, S., Nakamura, Y., Kaneko, T., Asamizu, E., Kato, T., Nakao, M., Sasamoto, S., Watanabe, A., Ono, A., Kawashima, K., Fujishiro, T., Katoh, M., Kohara, M., Kishida, Y., Minami, C., Nakayama, S., Nakazaki, N., Shimizu, Y., Shinpo, S., Takahashi, C., Wada, T., Yamada, M., Ohmido, N., Hayashi, M., Fukui, K., Baba, T., Nakamichi, T., Mori, H., and Tabata, S. 2008. Genome structure of the legume, Lotus japonicus. DNA Res. 15: 227–239.
- Sauer, M.A., Scott, R.A., and Cheesbrough, T.M. 2008. Marker-assisted selection for low linolenic acid content in Soybean. J. Crop Improv. 21: 139–155.
- Saxena, R.K., Penmetsa, R.V., Upadhyaya, H.D., Kumar, A., Carrasquilla-Garcia, N., Schlueter, J.A., Farmer, A., Whaley, A.M., Sarma, B.K., May, G.D., Cook, D.R., and Varshney, R.K. 2012. Large-scale development of cost-effective single-nucleotide polymorphism marker assays for genetic mapping in pigeonpea and comparative mapping in legumes. DNA Res. 19: 449–461.
- Schmutz, J., Cannon, S.B., Schlueter, J., Ma, J., Mitros, T., Nelson, W., Hyten, D.L., Song, Q., Thelen, J.J., Cheng, J., Xu, D., Hellsten, U., May, G.D., Yu, Y., Sakurai, T., Umezawa, T., Bhattacharyya, M.K., Sandhu, D., Valliyodan, B., Lindquist, E., Peto, M., Grant, D., Shu, S., Goodstein, D., Barry, K., Futrell-Griggs, M., Abernathy, B., Du, J., Tian, Z., Zhu, L., Gill, N., Joshi, T., Libault, M., Sethuraman, A., Zhang, X., Shinozaki, K., Nguyen, H.T., Wing, R.A., Cregan, P., Specht, J., Grimwood, J., Rokhsar, D., Stacey, G., Shoemaker, R.C., and Jackson, S.A. 2010. Genome sequence of the palaeopolyploid soybean. Nature 463: 178–183.
- Schnable, P.S., Ware, D., Fulton, R.S., Stein, J.C., Wei, F., Pasternak, S., Liang, C., Zhang, J., Fulton, L., Graves, T.A., Minx, P., Reily, A.D., Courtney, L., Kruchowski, S.S., Tomlinson, C., Strong, C., Delehaunty, K., Fronick, C., Courtney, B., Rock, S.M., Belter, E., Du, F., Kim, K., Abbott, R.M., Cotton, M., Levy, A., Marchetto, P., Ochoa, K., Jackson, S.M., Gillam, B., Chen, W., Yan, L., Higginbotham, J., Cardenas, M., Waligorski, J., Applebaum, E., Phelps, L., Falcone, J., Kanchi, K., Thane, T., Scimone, A., Thane, N., Henke, J., Wang, T., Ruppert, J., Shah, N., Rotter, K., Hodges, J., Ingenthron, E., Cordes, M., Kohlberg, S., Sgro, J., Delgado, B., Mead, K., Chinwalla, A., Leonard, S., Crouse, K., Collura, K., Kudrna, D., Currie, J., He, R., Angelova, A., Rajasekar, S., Mueller, T., Lomeli, R., Scara, G., Ko, A., Delaney, K., Wissotski, M., Lopez, G., Campos, D., Braidotti, M., Ashley, E., Golser, W., Kim, H., Lee, S., Lin, J., Dujmic, Z., Kim, W., Talag, J., Zuccolo, A., Fan, C., Sebastian, A., Kramer, M., Spiegel, L., Nascimento, L., Zutavern, T., Miller, B., Ambroise, C., Muller, S., Spooner, W., Narechania, A., Ren, L., Wei, S., Kumari, S., Faga, B., Levy, M.J., McMahan, L., Van Buren, P., Vaughn, M.W., Ying, K., Yeh, C.T., Emrich, S.J., Jia, Y., Kalyanaraman, A., Hsia, A.P., Barbazuk, W.B., Baucom, R.S., Brutnell, T.P., Carpita, N.C., Chaparro, C., Chia, J.M., Deragon, J.M., Estill, J.C., Fu, Y., Jeddeloh, J.A., Han, Y., Lee, H., Li, P., Lisch, D.R., Liu, S., Liu, Z., Nagel, D.H., McCann, M.C., SanMiguel, P., Myers, A.M., Nettleton, D., Nguyen, J., Penning, B.W., Ponnala, L., Schneider, K.L., Schwartz, D.C., Sharma, A., Soderlund, C., Springer, N.M., Sun, Q., Wang, H., Waterman, M., Westerman, R., Wolfgruber, T.K., Yang, L., Yu, Y., Zhang, L., Zhou, S., Zhu, Q., Bennetzen, J.L., Dawe, R.K., Jiang, J., Jiang, N., Presting, G.G., Wessler, S.R., Aluru, S., Martienssen, R.A., Clifton, S.W., McCombie, W.R., Wing, R.A., and Wilson, R.K. 2009. The B73 maize genome: complexity, diversity, and dynamics. Science 326: 1112–1115.
- Schneider, K.A., Grafton, K.F., and Kelly, J.D. 2001. QTL analysis of resistance to Fusarium root rot in bean. Crop Sci. 41: 535–442.
- Sekhar, K., Priyanka, B., Reddy, V.D., and Rao, K.V. 2010. Isolation and characterization of a pigeonpea cyclophilin (CcCYP) gene, and its over-expression in Arabidopsis confers multiple abiotic stress tolerance. Plant Cell Environ. 33: 1324–1338.
- Sekhon, R.S., Lin, H., Childs, K.L., Hansey, C.N., Buell, C.R., de Leon, N., and Kaeppler, S.M. 2011. Genome-wide atlas of transcription during maize development. Plant J. 66: 553–563.
- Selvaraj, M.G., Narayana, M., Schubert, A.M., Ayers, J.L., Baring, M.R., and Burow, M.D. 2009. Identification of QTLs for pod and kernel traits in cultivated peanut by bulked segregant analysis. Elec. J. Biotechnol. 12: DOI:10.2225/vol12.
- Severin, A.J., Woody, J.L., Bolon, Y.T., Joseph, B., Diers, B.W., Farmer, A.D., Muehlbauer, G.J., Nelson, R.T., Grant, D., Specht, J.E., Graham, M.A., Cannon, S.B., May, G.D., Vance, C.P., and Shoemaker, R.C. 2010. RNA-Seq Atlas of Glycine max: a guide to the soybean transcriptome. BMC Plant Biol. 10: 160.
- Shade, R.E., Schroeder, H.E., Pueyo, J.J., Tabe, L.M., Murdock, L.L., Higgins, T.J. V., and Chrispeels, M.J. 1994. Transgenic pea seeds expressing the α-amylase inhibitor of the common bean are resistant to bruchid beetles. Nat. Biotechnol. 12: 793–796.
- Sharpe, A.G., Ramsay, L., Sanderson, L.A., Fedoruk, M.J., Clarke, W.E., Li, R., Kagale, S., Vijayan, P., Vandenberg, A., and Bett, K.E. 2013. Ancient orphan crop joins modern era: gene-based SNP discovery and mapping in lentil. BMC Genomics 14: 192.
- Shi, A., Chen, P., Li, D.X., Zheng, C., Hou, A., and Zhang, B. 2008. Genetic confirmation of 2 independent genes for resistance to soybean mosaic virus in J05 soybean using SSR markers. J. Hered. 99: 598–603.
- Shi, A., Chen, P., Li, D., Zheng, C., Zhang, B., and Hou, A. 2009. Pyramiding multiple genes for resistance to soybean mosaic virus in soybean using molecular markers. Mol. Breeding 23: 113–124.
- Shirasawa, K., Bertioli, D.J., Varshney, R.K., Moretzsohn, M.C., Leal-Bertioli, S.C., Thudi, M., Pandey, M.K., Rami, J.F., Foncéka, D., Gowda, M.V., Qin, H., Guo, B., Hong, Y., Liang, X., Hirakawa, H., Tabata, S., and Isobe, S. 2013. Integrated consensus map of cultivated peanut and wild relatives reveals structures of the A and B genomes of Arachis and divergence of the legume genomes. DNA Res. 20: 173–184.
- Shirasawa, K., Koilkonda, P., Aoki, K., Hirakawa, H., Tabata, S., Watanabe, M., Hasegawa, M., Kiyoshima, H., Suzuki, S., Kuwata, C., Naito, Y., Kuboyama, T., Nakaya, A., Sasamoto, S., Watanabe, A., Kato, M., Kawashima, K., Kishida, Y., Kohara, M., Kurabayashi, A., Takahashi, C., Tsuruoka, H., Wada, T., and Isobe, S. 2012. In silico polymorphism analysis for the development of simple sequence repeat and transposon markers and construction of linkage map in cultivated peanut. BMC Plant Biol. 12: 80.
- Silva, C. and Danielle, G. 2008. Molecular mapping of two loci that confer resistance to Asian rust in soybean. Theor. Appl. Genet. 117: 57–63.
- Simpson, C.E., Krapovickas, A., and Valls, J.F. M. 2001. History of Arachis including evidence of A. hypogaea L. progenitors. Peanut Sci. 28:78–79.
- Simpson, C.E., Starr, J.L., Church, G.T., Burow, M.D., and Paterson, A.H. 2003. Registration of ‘NemaTAM’ peanut. Crop Sci. 43:1561.
- Sindhu, A., Marwan, M., Bett, K., Taran, B., Sharpe, A.G., Ramsay, L.D., and Warkentin, T. 2013. Development of a functional SNP map for pea and identification of markers linked to agronomic important traits. In: Plant and Animal Genome Conference XXI 2013, P0355.
- Smith, J. 2010. USDA, ARS, National Genetic Resources Program. Germplasm Resources Information Network - (GRIN). National Germplasm Resources Laboratory, Beltsville, Maryland.
- Smykal, P., Aubert, G., Burstin, J., Coyne, C.J., Ellis, T.H. N., Flavell, A.J., Ford, R., Hybl, M., Macas, J., Neumann, P., Kevin, E.E. McPhee, K.E., Redden, R.J., Rubiales, D., Weller, J.L., and Warkentin, T.D. 2012. Pea (Pisum sativum L.) in the Genomic Era. Agronomy. 2: 74–115.
- Song, X., Han, Y., Teng, W., Sun, G., and Li, W. 2010. Identification of QTL underlying somatic embryogenesis capacity of immature embryos in soybean (Glycine max (L.) Merr.). Plant Cell Rep. 29: 125–131.
- Soule, M., Porter, L., Medina, J., Santana, G.P., Blair, M.W., and Miklas, P.N. 2011. Comparative QTL map for white mold resistance in common bean, and characterization of partial resistance in dry bean lines VA19 and I9365-31. Crop Sci. 51: 123–139.
- Strausbaugh, C.A., Myers, J.R., Forster, R.L., and McClean, P.E. 1999. bc-1 and bc-u-two loci controlling bean common mosaic virus resistance in common bean are linked. J. Am. Soc. Hort. Sci. 124: 644–648.
- Sujay, V., Gowda, M.V. C., Pandey, M.K., Bhat, R.S., Khedikar, Y.P, Nadaf, H.L., Gautami, B., Sarvamangala, C., Lingaraju, S., Radhakrishan, T., Knapp, S.J., and Varshney, R.K. 2012. Quantitative trait locus analysis and construction of consensus genetic map for foliar disease resistance based on two recombinant inbred line populations in cultivated groundnut (Arachis hypogaea L.). Mol. Breeding 30: 773–788.
- Sun, D., Li, W., Zhang, Z., Chen, Q., Ning, H., Qiu, L., and Sun, G. 2006. Quantitative trait loci analysis for the developmental behavior of Soybean [Glycine max (L.) Merr.]. Theor. Appl. Genet. 112: 665–673.
- Sundaram, R.M., Vishnupriya, M.R., Laha, G.S., Rani, N.S., Rao, P.S., Balachandran, S.M., Reddy, G.A., Sarma, N.P., and Sonti R.V. 2009. Introduction of bacterial blight resistance into Triguna, a high yielding, mid-early duration rice variety. Biotechnology J. 4: 400–407.
- Tachi, H., Fukuda-Yamada, K., Kojima, T., Shiraiwa, M., and Takahara, H. 2009. Molecular characterization of a novel soybean gene encoding a neutral PR-5 protein induced by high-salt stress. Plant Physiol. Biochem. 47: 73–79.
- Takanashi, K., Takahashi, H., Sakurai, N., Sugiyama, A., Suzuki, H., Shibata, D., Nakazono, M., and Yazaki, K. 2012. Tissue-specific transcriptome analysis in nodules of Lotus japonicus. Mol. Plant-Microbe Interact. 25: 869–876.
- Takeshi, H. and Hiroyoshi, I. 2010. EM algorithm for Bayesian estimation of genomic breeding values. BMC Genet. 11: 3.
- Tar’an, B., Warkentin, T.D., Tullu, A., and Vandenberg, A. 2007. Genetic mapping of ascochyta blight resistance in chickpea (Cicer arietinum L.) using a simple sequence repeat linkage map. Genome 50: 26–34.
- Tar’an, B., Warkentin, T., Somers, D.J., Miranda, D., Vandenberg, A., Blade, S., Woods, S., Bing, D., Xue, A., DeKoeyer, D., and Penner, G. 2003a. Quantitative trait loci for lodging resistance, plant height and partial resistance to mycosphaerella blight in field pea (Pisum sativum L.). Theor. Appl. Genet. 107: 1482–1491.
- Tar’an, B., Warkentin, T., Somers, D.J., Miranda, D., Vandenberg, A., Blade, S., Woods, S., and Bing, D. 2004. Identification of quantitative trait loci for grain yield, seed protein concentration and maturity in field pea (Pisum sativum L.). Euphytica 136: 297–306.
- Tar’an, B., Buchwaldt, L., Tullu, A., Banniza, S., Warkentin, T.D., and Vandenberg, A. 2003b. Using molecular markers to pyramid genes for resistance to ascochyta blight and anthracnose in lentil (Lens culinaris Medik). Euphytica 134: 223–230.
- Teixeira, F.F., Dos Santos, J.B., Ramalho, M.A. P., Abreu, A.F. B., Guimaraes, C.T., and de Oliveira, A.C. 2005. QTL mapping for angular leaf spot in common bean using microsatellite markers. Crop Breed. Appl. Biotechnol. 5: 272–278.
- Tester, M. and Langridge, P. 2010. Breeding technologies to increase crop production in a changing world. Science 327: 818–822.
- Thudi, M., Bohra A., Nayak, S.N., Varghese, N., Shah, T.M., Penmesta, R.V., Thirunavukkarasu, N., Gudipati, S., Gaur, P.M., Kulwal, P.K., Upadhyaya, H.D., KaviKishor P.B., Winter, P., Kahl, G., Town, C.D., Kilian, A., Cook, D.R., and Varshney, R.K. 2011. Novel SSR markers from BAC-end sequences, DArT arrays and a comprehensive genetic map with 1,291 marker loci for chickpea (Cicer arietinum L.). PLoS ONE 6: e27275.
- Thudi, M., Li, Y., Jackson, S.A., May, G.D., and Varshney, R.K. 2012. Current state-of-art of sequencing technologies for plant genomics research. Brief Funct.Genomics 11: 3–11.
- Timmerman-Vaughan, G.M., Frew, T.J., Russell, A.C., Khan, T., Butler, R., Gilpin, M., Murray, S., and Falloon, K. 2002. QTL mapping of partial resistance to field epidemics of ascochyta blight of pea. Crop Sci. 42: 2100–2111.
- Timmerman-Vaughan, G.M., Mills, A., Whitfield, C., Frew, T., Butler, R., Murray, S., Lakeman, M., McCallum, J., Russell, A., and Wilson, D. 2005. Linkage mapping of QTL for seed yield, yield components, and development traits in pea. Crop Sci. 45: 1336–1344.
- Timmerman-Vaughan, G.M., Frew, T.J., Butler, R., Murray, S., Gilpin, M., Falloon, K., Johnston, P., Lakeman, M.B., Russell, A., and Khan, T. 2004. Validation of quantitative trait loci for Ascochyta blight resistance in pea (Pisum sativum L.), using populations from two crosses. Theor. Appl. Genet. 109: 1620–1631.
- Tullu, A., Tar’an, B., Breitkreutz, C., Buchwaldt, L., Banniza, S., Warkentin, T.D., and Vandenberg, A. 2006. A quantitative trait locus for resistance to ascochyta blight [Ascochyta lentis] maps close to a gene for resistance to anthracnose [Colletotrichum truncatum]. Can. J. Plant Pathol. 28: 588–595.
- Tullu, A., Tar’an, B., Warkentin, T., and Vandenberg, A. 2008. Construction of an intraspecific linkage map and QTL analysis for earliness and plant height in lentil. Crop Sci. 48: 2254–2264.
- Tuskan, G., DiFazio, S., Jansson, S., Bohlmann, J., Grigoriev, I., Hellsten, U., Putnam, N., Ralph, S., Rombauts, S., Salamov, A., Schein, J., Sterck, L., Aerts, A., Bhalerao, R.R., Bhalerao, R.P., Blaudez, D., Boerjan, W., Brun, A., Brunner, A., Busov, V., Campbell M., Carlson, J., Chalot, M., Chapman, J., Chen, G.-L., Cooper, D., Coutinho, P.M., Couturier, J., Covert, S., Cronk, Q., Cunningham, R., Davis, J., Degroeve, S., Déjardin, A., dePamphilis, C., Detter, J., Dirks, B., Dubchak, I., Duplessis, S., Ehlting, J., Ellis, B., Gendler, K., Goodstein, D., Gribskov, M., Grimwood, J., Groover, A., Gunter, L., Hamberger, B., Heinze, B., Helariutta, Y., Henrissat, B., Holligan, D., Holt, R., Huang, W., Islam-Faridi, N., Jones, S., Jones-Rhoades, M., Jorgensen, R., Joshi, C., Kangasjärvi, J., Karlsson, J., Kelleher, C., Kirkpatrick, R., Kirst, M., Kohler, A., Kalluri, U., Larimer, F., Leebens-Mack, J., Leplé, J.-C., Locascio, P., Lou, Y., Lucas, S., Martin, F., Montanini, B., Napoli, C., Nelson, D.R., Nelson, C., Nieminen, K., Nilsson, O., Pereda, V., Peter, G., Philippe, R., Pilate, G., Poliakov, A., Razumovskaya, J., Richardson, P., Rinaldi, C., Ritland, K., Rouzé, P., Ryaboy, D., Schmutz, J., Schrader, J., Segerman, B., Shin, H., Siddiqui, A., Sterky, F., Terry, A., Tsai, C.-J., Uberbacher, E., Unneberg, P., Vahala, J., Wall, K., Wessler, S., Yang, G., Yin, T., Douglas, C., Marra, M., Sandberg, G., Van de Peer, Y., and Rokhsar, D. 2006. The genome of black cottonwood, Populus trichocarpa (Torr. & Gray). Science 313: 1596–1604.
- Tuyen, D.D., Lal, S.K., and Xu, D.H. 2010. Identification of a major QTL allele from wild soybean (Glycine soja) for increasing alkaline salt tolerance in soybean. Theor. Appl. Genet. 121: 229–236.
- Ubayasena, L., Bett, K., Tar’an, B., Vijayan, P., and Warkentin, T.D. 2010. Genetic control and QTL mapping of cotyledon bleaching resistance in green field pea (Pisum sativum L.). Genome 53: 346–359.
- Urbański, D.F., Małolepszy, A., Stougaard, J., and Andersen, S.U. 2012. Genome‐wide LORE1 retrotransposon mutagenesis and high‐throughput insertion detection in Lotus japonicus. Plant J. 69: 731–741.
- Urbański, D.F., Małolepszy, A., Stougaard, J., and Andersen, S.U. 2013. High-throughput and targeted genotyping of Lotus japonicus LORE1 insertion mutants. Methods Mol. Biol. 1069: 119–146.
- Vandemark, G.J., Foune, D., and Mlidas, P.N. 2008. Genotyping with real-time PCR reveals recessive epistasis between independent QTL conferring resistance to common bacterial blight in dry bean. Theor. Appl. Genet. 117: 513–522.
- Varshney, R.K., Bertioli, D.J., Moretzsohn, M.C., Vadez, V., Krishnamurthy, L., Aruna, R., Nigam, S.N., Moss, B.J., Seetha, K., Ravi, K., He, G., Knapp, S.J., and Hoisington, D.A. 2009b. The first SSR-based genetic linkage map for cultivated groundnut (Arachis hypogaea L.). Theor. Appl. Genet. 118: 729–739.
- Varshney, R.K., Chen, W., Li, Y., Bharthi A.K., Saxena, R.K., Schlueter, J.A., Donoghue, M.A., Azam, S., Fan, G., Whaley, A.M., Farmer, A.D., Sheridan, J., Iwata, A., Tuteja, R., Penmetsa, R.V., Wu, W., Upadhyaya, H.D., Yang, S., Shah, T., Saxena, K.B., Michael, T., McCombie, W.R., Yang, B., Zhang, G., Yang, H., Wang, J., Spillane, C., Cook, D.R., May, G.D., Xu, X., and Jackson, S.A. 2012a. Draft genome sequence of pigeonpea (Cajanus cajan), an orphan legume crop of resource-poor farmers. Nat. Biotechnol. 30: 83–89.
- Varshney, R.K., Gaur, P.M., Chamarthi, S.K., Krishnamurthy, L., Tripathi, S., Kashiwagi, J., Samineni, S., Singh, V.K., Thudi, M., and Jaganathan, D. 2013b. Fast-track introgression of “QTL-hotspot” for root traits and other drought tolerance traits in JG 11, an elite and leading variety of chickpea. Plant Gen. DOI: 10.3835/plantgenome2013.07.0022.
- Varshney, R.K., Glaszmann, J.C., Leung, H., and Ribaut, J.M. 2010b. More genomic resources for less-studied crops. Trends Biotechnol. 28: 452–460.
- Varshney, R.K., Graner, A., and Sorrells, M.E. 2005. Genomics-assisted breeding for crop improvement. Trends Plant Sci. 10: 621–630.
- Varshney, R.K., Kudapa, H., Roorkiwal, M., Thudi, M., Pandey, M., Saxena, R., Chamarthi, S.K.S. Mohan, M.S., Upadhyaya, H.D., Gaur, P.M., Krishnamurthy, L., Saxena, K.B., Nigam S., and Pande, S. 2012b. Advances in genetics and molecular breeding of three legume crops of semi-arid tropics using next-generation sequencing and high-throughput genotyping technologies. J. Bioscience. 37: 811–820.
- Varshney, R.K., Mohan, S.M., Gaur, P.M., Chamarthi, S.K., Singh, V.K., Srinivasan, S., Swapna, N., Sharma, M., Singh, S., Kaur, L., and Pande S. 2013c. Marker-assisted backcrossing to introgress resistance to Fusarium wilt (FW) race 1 and Ascochyta blight (AB) in C 214, an elite cultivar of chickpea. Plant Gen. DOI: 10.3835/plantgenome2013.10.0035.
- Varshney, R.K., Nayak, S.N., May, G.D., and Jackson, S.A. 2009a. Next generation sequencing technologies and their application for crop genetics and breeding. Trends Biotechnol. 27: 522–530.
- Varshney, R.K., Pandey, M.K., Janila, P., Nigam, S.N., Sudini, H., Gowda, M.V. C., Sriswathi, M., Radhakrishnan, T., Manohar, S.S., and Nagesh, P. 2014. Marker-assisted introgression of a QTL region to improve rust resistance in three elite and popular varieties of peanut (Arachis hypogaea L.). Theor. Appl. Genet. DOI 10.1007/s00122-014-2338-3
- Varshney, R.K., Song, C., Saxena, R.K., Azam, S., Yu, S., Sharpe, A.G., Cannon, S. Baek, J., Rosen, B.D., Tar’an, B., Millan, T., Zhang, X., Ramsay, L.D., Iwata, A., Wang, Y., Nelson, W., Farmer, A.D., Gaur, P.M., Soderlund, C., Penmetsa, R.V., Xu, C., Bharti, A.K., He, W., Winter, P., Zhao, S., Hane, J.K., Carrasquilla-Garcia, N., Condie, J.A., Upadhyaya, H.D., Luo, M., Thudi, M., Gowda, C.L. L., Singh, N.P., Lichtenzveig, J., Gali, K.K., Rubio, J., Nadarajan, N., Dolezel, J., Bansal, K.C., Xu, X., Edwards, D., Zhang, G., Kahl, G., Gil, G., Singh, K.B., Datta, S.K., Jackson, S.A., Wang, J., and Cook, D.R. 2013a. Draft genome sequence of chickpea (Cicer arietinum) provides a resource for trait improvement. Nat. Biotechnol. 31: 240–246.
- Varshney, R.K., Thudi, M., May, G.D., and Jackson, S.A. 2010a. Legume genomics and breeding. Plant Breed. Rev. 33: 257–304.
- Vuong, T.D., Diers, B.W., and Hartman, G.L. 2008. Identification of QTL for resistance to Sclerotinia stem rot (Sclerotinia sclerotiorum) in soybean plant introduction 194639. Crop Sci. 48: 2209–2214.
- Vuong, T.D., Sleper, D.A., Shannon, J.G., and Nguyen, H.T. 2010. Novel quantitative trait loci for broad-based resistance to soybean cyst nematode (Heteroderaglycines Ichinohe) in soybean PI 567516C. Theor. Appl. Genet. 121: 1253–1266.
- Walker, D.R., Boenna, H.R, All, J.N., and Parrott, W.A. 2002. Combining crylAc with QTL alleles from PI229358 to improve soybean resistance to lepidopteran pests. Mol. Breeding 9: 43–51.
- Wang, D., Ma, Y., Liu, N., Yang, Z., Zheng, G., and Zhi, H. 2011. Fine mapping and identification of the soybean RSC4 resistance candidate gene to soybean mosaic virus. Plant Breeding 130: 653–659.
- Wang, H., Pandey, M.K., Qiao, L., Qin, H., Culbreath, A.K., He, G., Varshney, R.K., and Guo, B. 2013. Genetic mapping and QTL analysis for disease resistance using F2 and F5generation-based genetic maps derived from Tifrunner × GT-C20 in peanut (Arachis hypogaea L.). Plant Gen. DOI: 10.3835/plantgenome2013.05.0018.
- Wang, H., Penmetsa, R.V., Yuan, M., Gong, L., Zhao, Y., Guo, B., Farmer, A.D., Rosen, B.D., Gao, J., Isobe, S., Bertioli, D.J., Varshney, R.K., Cook, D.R., and He, G. 2012. Development and characterization of BAC-end sequence derived SSRs and their incorporation into a new higher density genetic map for cultivated peanut (Arachis hypogaeaL.). BMC Plant Biol. 12: 10.
- Wang, H., Waller, L., Tripathy, S., St. Martin, S.K., Zhou, L., Krampis, K., Tucker, D.M., Mao, Y., Hoeschele, I., SaghaiMaroof, M.A., Tyler, B.M., and Dorrance, A.E. 2010. Analysis of genes underlying soybean quantitative trait loci conferring partial resistance to Phytophthora sojae. Plant Gen. 3: 23–40.
- Wang, N., Khan, W., and Smith, D.L. 2012. Changes in soybean global gene expression after application of lipo-chitooligosaccharide from Bradyrhizobium japonicum under sub-optimal temperature. PLoS ONE 7: e31571.
- Warrington, C.V., Zhu, S., Parrot, W.A., All, J.N., and Boerma, H.R. 2008. Seed yield of near-isogenic soybean lines with introgressed quantitative trait loci conditioning resistance to corn earworm (Lepidoptera: Noctuidae) and soybean looper (Lepidoptera: Noctuidae) from PI 229358. J. Econ. Entomol. 101: 1471–1477.
- Weller, J.L., Liew, L.C., Hecht, V.F. G., Rajandran, V., Laurie, R.E., Ridge, S., Wenden, B., Vander Schoor, J.K., Jaminon, O., Blassiau, C., Dalmais, M., Rameau, C., Bendahmane, A., Macknight, R.C., and Lejeune-Hénaut I. 2012. A conserved molecular basis for photoperiod adaptation in two temperate legumes. Proc. Natl. Acad. Sci. U S A 51: 21158–21163.
- Winter, S.M. J., Shelp, B.J., Anderson, T.R., Welacky, T.W., and Rajcan, I. 2007. QTL associated with horizontal resistance to soybean cyst nematode in Glycine soja PI464925B. Theor. Appl. Genet. 114: 461–472.
- Wu, X., Blake, S., Sleper, D.A., Shannon, J.G., Cregan, P.B., and Nguyen, H.T. 2009. QTL, additive, and epistatic effects for SCN resistance in PI 437654. Theor. Appl. Genet. 118: 1093–1105.
- Xia, Z., Tsubokura, Y., Hoshi, M., Hanawa, M., Yano, C., Okamura, K., Ahmed, T.A., Anai, T., Watanabe, S., Hayashi, M., Kawai, T., Hossain, K.G., Masaki, H., Asai, K., Yamanaka, N., Kubo, N., Kadowaki, K., Nagamura, Y., Yano, M., Sasaki, T., and Harada, K. 2007. An integrated high-density linkage map of soybean with RFLP, SSR, STS, and AFLP markers using a single F2 population. DNA Res. 14: 257–269.
- Xu, X., Liu, X., Ge, S., Jensen, J.D., Hu, F., Li, X., Dong, Y., Gutenkunst, R.N., Fang, L., Huang, L., Li, J., He, W., Zhang, G., Zheng, X., Zhang, F., Li, Y., Yu, C., Kristiansen, K., Zhang, X. ,Wang, J., Wright, M., McCouch, S., Nielsen, R., Wang, J., and Wang, W. 2011. Resequencing 50 accessions of cultivated and wild rice yields markers for identifying agronomically important genes. Nature Biotechnol. 30: 105–111.
- Xu, Y., Lu, Y., Xie, C., Gao, S., Wan, J., and Prasanna, B.M. 2012. Whole-genome strategies for marker-assisted plant breeding. Mol. Breeding 29: 833–854.
- Yang, T., Bao, S., Ford, R., Jia, T., Guan, J., He, Y., Sun, X., Jiang, J., Hao, J., Zhang, X., and Zong, X. 2012. High-throughput novel microsatellite marker of faba bean via next generation sequencing. BMC Genomics 13: 602.
- Yin, Z., Meng, F., Song, H., He, X., Xu, X., and Yu, D. 2010. Mapping quantitative trait loci associated with chlorophyll a fluorescence parameters in soybean (Glycine max (L.) Merr.). Planta 231: 875–885.
- Young, N.D., Debellé, F., Oldroyd, G.E., Geurts, R., Cannon, S.B., Udvardi, M.K., Benedito, V.A., Mayer, K.F. X., Gouzy, J., Schoof, H., Van de Peer, Y., Proost, S., Cook, D.R., Meyers, B.C., Spannagl, M., Cheung, F., De Mita, S., Krishnakumar, V., Gundlach, H., Zhou, S., Mudge, J., Bharti, A.K., Murray, J.D., Naoumkina, M.A., Rosen, B., Silverstein, K.A., Tang, H., Rombauts, S., Zhao, P.X., Zhou, P., Barbe, V., Bardou, P., Bechner, M., Bellec, A., Berger, A., Bergès, H., Bidwell, S., Bisseling, T., Choisne, N., Couloux, A., Denny, R., Deshpande, S., Dai, X., Doyle, J.J., Dudez, A.M., Farmer, A.D., Fouteau, S., Franken, C., Gibelin, C., Gish, J., Goldstein, S., González, A.J., Green, P.J., Hallab, A., Hartog, M., Hua, A., Humphray, S.J., Jeong, D.H., Jing, Y., Jöcker, A., Kenton, S.M., Kim, D.J., Klee, K., Lai, H., Lang, C., Lin, S., Macmil, S.L., Magdelenat, G., Matthews, L., McCorrison, J., Monaghan, E.L., Mun, J.H., Najar, F.Z., Nicholson, C., Noirot, C., O’Bleness, M., Paule, C.R., Poulain, J., Prion, F., Qin, B., Qu, C., Retzel, E.F., Riddle, C., Sallet, E., Samain, S., Samson, N., Sanders, I., Saurat, O., Scarpelli, C., Schiex, T., Segurens, B., Severin, A.J., Sherrier, D.J., Shi, R., Sims, S., Singer, S.R., Sinharoy, S., Sterck, L., Viollet, A., Wang, B.B., Wang, K., Wang, M., Wang, X., Warfsmann, J., Weissenbach, J., White, D.D., White, J.D., Wiley, G.B., Wincker, P., Xing, Y., Yang, L., Yao, Z., Ying, F., Zhai, J., Zhou, L., Zuber. A., Dénarié. J., Dixon, R.A., May, G.D., Schwartz, D.C., Rogers, J., Quétier, F., Town, C.D., and Roe, B.A. 2011. The Medicago genome provides insight into the evolution of rhizobial symbioses. Nature 480: 520–524.
- Yu, J., Hu, S., Wang, J., Wong, G.K. S., Li, S., Liu, B., Deng, Y., Dai, L., Zhou, Y., Zhang, X., Cao, M., Liu, J., Sun, J., Tang, J.B., Chen, Y.J., Huang, X.B., Lin, W., Ye, C., Tong, W., Cong, L.J., Geng, J.N., Han, Y.J., Li, L., Li, W., Hu, G.Q., Yuan, L.P., Huang, X., Li, W., Li, J., Liu, Z., Li, L., Liu, J., Qi, Q., Liu, J., Li, L., Li, T., Wang, X., Lu, H., Wu, T., Zhu, M., Ni, P., Han, H., Dong, W., Ren, X., Feng, X., Cui, P., Li, X., Wang, H., Xu, X., Zhai, W., Xu, Z., Zhang, J., He, S., Zhang, J., Xu, J., Zhang, K., Zheng, X., Dong, J., Zeng, W., Tao, L., Ye, J., Tan, J., Ren, X., Chen, X., He, J., Liu, D., Tian, W., Tian, C., Xia, H., Bao, Q., Li, G., Gao, H., Cao, T., Wang, J., Zhao, W., Li, P., Chen, W., Wang, X., Zhang, Y., Hu, J., Wang, J., Liu, S., Yang, J., Zhang, G., Xiong, Y., Li, Z., Mao, L., Zhou, C., Zhu, Z., Chen, R., Hao, B., Zheng, W., Chen, S., Guo, W., Li, G., Liu, S., Tao, M., Wang, J., Zhu, L., Yuan, L., and Yang, H. 2002. A draft sequence of the rice genome (Oryza sativa L. ssp. indica). Science 296: 79–92.
- Zhang, J., Liang, S., Duan, J., Wang, J., Chen, S., Cheng, Z., Zhang, Q., Liang, X., and Li, Y. 2012. De novo assembly and characterisation of the transcriptome during seed development, and generation of genic-SSR markers in Peanut (Arachis hypogaea L.). BMC Genomics 13: 90.