Abstract
The rapid development of microbial resistance to conventional antibiotics has accelerated efforts to find anti-infectives with a novel mode-of-action, which are less prone to bacterial resistance. Intense nonclinical and clinical research is today ongoing to evaluate antimicrobial peptides (AMPs) as potential next-generation antibiotics. Currently, multiple AMPs are assessed in late-stage clinical trials, not only as novel anti-infective drugs, but also as innovative product candidates for immunomodulation, promotion of wound healing, and prevention of post-operative scars. The efforts to translate AMP-based research findings into pharmaceutical product candidates are expected to accelerate in coming years due to technological advancements in multiple areas, including an improved understanding of the mechanism-of-action of AMPs, smart formulation strategies, and advanced chemical synthesis protocols. At the same time, it is recognized that cytotoxicity, low metabolic stability due to sensitivity to proteolytic degradation, and limited oral bioavailability are some of the key weaknesses of AMPs. Furthermore, the pricing and reimbursement environment for new antimicrobial products remains as a major barrier to the commercialization of AMPs.
Introduction
The rapidly increasing resistance to conventional antibiotics all over the world has resulted in intensive research now being invested into bringing new and non-conventional anti-infective drugs to the market. The antimicrobial peptides (AMPs), also known as host defense peptides, have recently captured attention as innovative drug candidates for the treatment of infectious disease and as novel immunomodulatory therapies (). Although AMPs represent considerable diversity in their sources (natural/synthetic), mechanisms-of-action, structural and physicochemical characteristics, and therapeutic focus areas, many development programs involving AMPs for the treatment of human disease share commonalities in terms of strengths and opportunities but also technical barriers as well as regulatory and commercial risks as discussed in this review.
Figure 1. Published research on AMPs in PubMed (https://www.ncbi.nlm.nih.gov/pubmed) between years 2000 and 2018. The database was searched using the terms AMPs, antimicrobial peptides, host defense peptides, or host defence peptides.
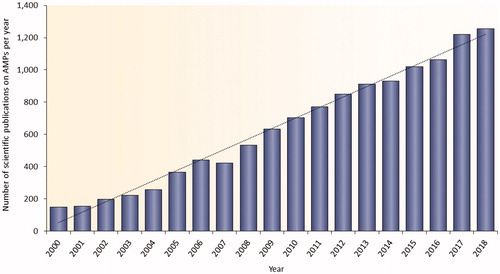
Natural AMPs are evolutionary conserved molecules that display structural and functional diversity and are found in virtually all organisms (). Most AMPs directly kill microbial pathogens and many of them possess profoundly wide range of antimicrobial action that includes activities against Gram-positive and Gram-negative microbes, fungi, unicellular protozoa, and viruses [Citation1–4]. In addition, several AMPs exhibit immunomodulatory activity thereby indirectly promoting pathogen clearance of the host [Citation5,Citation6]. Most AMPs are short (typically, 10–50 amino acids), possess a positive net charge (commonly ranging from +2 to +11), and contain a significant proportion of hydrophobic residues (typically 50%) [Citation7–9]. AMPs are classified based on their secondary structure into three categories characterized by a α-helical, β-sheet, or extended/random-coil structure. Notably, the majority of AMPs belong to the first two classes [Citation9–12].
Figure 2. Sources of AMPs registered in the Antimicrobial Peptide Database (APD; http://aps.unmc.edu/AP, accessed on 17 September 2019). The APD contains 3128 AMPs from six kingdoms of life (bacteria, archaea, protists, fungi, plants, and animals).
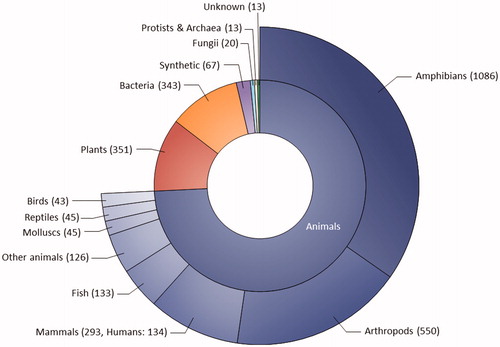
There are encouraging examples of several AMPs already introduced into medical practice, such as the well-characterized cyclic anti-infective peptides gramicidins and polymyxins. Furthermore, a wide range of additional AMPs is currently being evaluated in nonclinical settings and in clinical trials for several indication areas. Examples of AMPs that have progressed into late-stage clinical studies include omiganan, pexiganan, and DPK-060 developed for the treatment against infections, LL-37 developed for improved healing of venous leg ulcers, and PXL01 evaluated for preventing post-surgical adhesions; all these AMPs are linear cationic peptides with the molecular length varying from 12 (omiganan) to 37 (LL-37) amino acids.
This review focuses on AMPs as potential therapeutic agents with an emphasis on the opportunities and vulnerabilities of this drug category from technical, regulatory, and commercial perspectives.
Mode-of-action (MOA) of AMPs
An understanding of their MOA is a prerequisite to develop efficient and safe AMPs as novel candidate drugs. Interestingly, the pharmacodynamics of AMPs is complex and several AMPs are known to display multiple and complementary effects in the body, which vary depending on parameters, such as peptide concentration, tissue localization, target pathogen, growth phase, and local environment [Citation10,Citation13–16].
Most AMPs exert a direct microbicidal effect by disrupting the membrane integrity of the target organism and/or by translocating across the microbial membrane to reach the intracellular targets [Citation7]. Notably, the antibacterial effect of AMPs is often very rapid, for example chicken cathelicidin-2 (CATH-2), the tick defensin-derived Os(3–12)NH2, and the computer-designed peptide PaDBS1R1 all killed microbes within 5–10 min of exposure [Citation17–19]. Membrane interactions, mediated by the electrostatic forces between the positively charged AMPs and bacterial surface that is negatively charged, are critical for the direct microbicidal action of AMPs [Citation5,Citation20]. Both Gram-positive and Gram-negative microbes possess cytoplasmic membranes that are rich in phospholipids, which carry negatively charged head groups, strongly promoting interactions with cationic AMPs. Furthermore, teichoic acids that are present in the cell wall of Gram-positive microbes, and lipopolysaccharides (LPS) that are constituents of the outer membrane of Gram-negative microbes, supply further electronegative charge to the surface of bacteria, strengthening the interaction with AMPs [Citation20,Citation21]. Once in contact with the bacterial surface, the AMP forms an amphipathic structure [Citation7,Citation10,Citation22], where the hydrophilic segments of the peptide interact with the charged head groups of phospholipids, and the hydrophobic peptide domains interact with the lipid bilayer [Citation20].
The three classical models of bacterial membrane perturbation by AMPs, i.e. barrel-stave model, toroidal-pore model, and carpet model [Citation23,Citation24], are described in . As examples, the fungal peptide alamethicin is described to use the barrel-stave model [Citation25], the amphibian magainin 2-analog Mag H2 forms toroidal pores [Citation26], and the amphibian AMP aurein 1.2 disrupts membranes via the carpet mechanism [Citation27].
Figure 3. Schematic presentation of the MOA of AMPs illustrating the broad range of immunomodulatory functions. The three classical models proposed to explain the permeabilization of bacterial cytoplasmic membrane by AMPs are shown. In the barrel-stave model, the peptides insert into the membrane resulting in the formation of a pore lined with AMPs oriented with their hydrophilic faces toward the interior of the pore and their hydrophobic faces toward the lipid core of the bilayer. A transmembrane pore is also formed in the toroidal-pore model; however, in this model the pore is lined with both the hydrophilic faces of the AMPs and the head groups of membrane phospholipids. In the carpet model, the AMPs accumulate on the surface of the membrane causing tension and eventually disruption of the membrane integrity and formation of micelles. The structure of the AMP in the figure is adapted from RCSB Protein Data Bank (ID 2K6O). PRR, pathogen recognition receptors
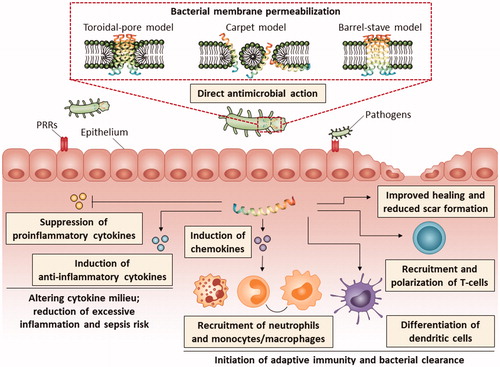
AMPs can also destabilize the bacterial membrane by decreasing or increasing its thickness (membrane thinning/thickening model) [Citation28] or by causing phospholipid head groups in the membrane to cluster (charged lipid clustering model) [Citation29,Citation30]. Furthermore, AMPs can cause membrane permeabilization by forming a complex with small organic anions carrying them across the membrane [Citation10,Citation31] or by inducing molecular electroporation, where the charged AMPs generate a transmembrane potential resulting in pore formation and molecule translocation [Citation32,Citation33]. Additionally, AMPs can induce the formation of non-bilayer intermediates internalizing the peptide within the membrane, followed by collapse of the intermediate and peptide release [Citation30,Citation34], whereas according to the non-lytic membrane depolarization model, the AMPs primarily cause dissipation of the membrane potential without significant structural damage of the membrane [Citation10,Citation35]. Interestingly, several AMPs have been described to alter the lipid phase behavior in the bacterial membrane through direct interactions with lipids that compose the membranes including inducing positive membrane curvature, negative membrane curvature, and cubic lipid phases [Citation30,Citation34]. A peptide-induced positive curvature strain facilitates the formation of toroidal pores and micelles, a negative curvature stress can lead to formation of non-bilayer intermediates, and the induction of cubic lipid phases by AMPs may result in porous structures in the membrane [Citation30,Citation34,Citation36].
It is suggested that membrane permeabilization by AMPs initially results in leakage of metabolites, membrane dysfunction, and ultimately, membrane rupture and the lysis of bacteria [Citation37–39]. Membrane permeabilization is also a key step allowing certain AMPs to translocate into the bacterial cytoplasm to target intracellular processes such as the synthesis of DNA, RNA, and protein, enzymatic activity, cell wall synthesis, and protein folding [Citation10,Citation37,Citation38,Citation40]. Examples of AMPs with intracellular targets include teixobactin that prevents cell wall synthesis by binding the peptidoglycan precursor lipid II [Citation41], indolicidin that binds to DNA thereby inhibiting DNA replication [Citation35], the larvae peptide Lser-PRP2 that is suggested to inhibit protein folding by binding to the bacterial chaperone DnaK [Citation42], and the proline-rich peptide Bac5 that blocks translation by binding to ribosomes [Citation43].
In addition to direct bactericidal action, many AMPs exert complex immunomodulatory activities. The wide array of immunomodulatory functions displayed by AMPs include enhanced chemotaxis of immune cells, activation of immune cell differentiation including dendritic cell maturation and initiation of adaptive immunity, repression of cytokine-mediated and Toll-like receptor (TLR)-mediated release of proinflammatory cytokines and reactive oxygen species, induction of anti-inflammatory cytokines, scavenging of bacterial endotoxins, stimulating angiogenesis, enhancing wound healing, and reducing scar formation () [Citation44,Citation45]. As an example, the bovine bactenecin-derived 12-meric host defense peptide IDR-1002 has recently been described to dampen the TNF-α, IL-6, IL-8, and nitric oxide production triggered by TLR ligands [Citation46–48] and, in addition to suppressing the release of proinflammatory cytokines, peptides such as bactenecin-derived IDR-1018 and tunicate clavanin A also increase the expression of the anti-inflammatory cytokine IL-10 [Citation49,Citation50]. Examples of AMPs with LPS-scavenging properties include frog dermaseptin-derived peptides [Citation51] and thrombin-derived C-terminal peptides (TCPs) [Citation52,Citation53], whereas the centipede peptide scolopendrasin IX and ToAP2 identified from the venom of the scorpion have been described as chemotactic for immune cells [Citation54,Citation55]. The human endogenous peptide beta-defensin 2 (hBD-2) and its derivative A-hBD-2 are examples of AMPs which have recently been shown to increase the proliferation and migration of keratinocytes in vitro and improve wound healing in vivo [Citation56].
It has generally been considered that the development of widespread microbial resistance against AMPs is unlikely since AMPs, in contrast to conventional antibiotics, attack multiple low-affinity targets (such as bacterial membranes) rather than a single high-affinity target, making it more difficult for target pathogens to defend themselves by developing resistance [Citation57,Citation58]. Moreover, it has been postulated that the rapid microbicidal action of AMPs precludes strong resistance evolution [Citation59]. There are numerous examples of AMPs that maintain their activity profile even after long exposure to the bacterial strains [Citation60–62]; however, experimental data has recently been presented describing mechanisms by which microorganisms may develop resistance toward AMPs under continuous selection pressure [Citation63–65], emphasizing the need of additional studies to describe the potential risks of microbial AMP resistance. Importantly, further systematic investigations to elucidate the molecular mechanism-of-action of different AMPs in a range of bacterial species are essential to understand what dictates the bacterial propensity to evolve resistance against AMPs, in order to guide the design and optimization of more efficient AMP-based drug candidates.
Therapeutic applications of AMPs
The broad-spectrum and rapid bactericidal action of AMPs, combined with a postulated low risk for resistance development, makes AMPs promising candidates for antimicrobial drugs [Citation66]. A handful of AMPs are approved today for clinical use as anti-infectives, with cyclic peptides, such as gramicidins and polymyxins being the most well characterized. Gramicidins are not used systemically due to hemolytic effects; instead, gramicidins are mainly administrated locally to treat infections in surface wounds as well as nasal, ocular, and throat infections [Citation67]. Polymyxins are mostly used for local applications to treat eye infections and for selective decontamination of the digestive tract but also for systemic treatment of infections caused by drug-resistant Gram-negative pathogens [Citation68]. Daptomycin is an example of a cyclic AMP introduced more recently into clinical practice (approved by the FDA in 2003) to treat complicated skin and skin-structure infections (cSSSI) by Gram-positive bacteria, in particular Staphylococcus aureus [Citation69].
A large number of additional AMPs are currently being evaluated in clinical trials for treatment against various bacterial and fungal infections, with linear AMPs omiganan, pexiganan, and DPK-060 being some of the most well studied (). Omiganan (CLS001 or MBI-226), a 12-amino-acid analog of the indolicidin (isolated from bovine neutrophils), displays efficacy against Gram-positive and Gram-negative bacteria as well as fungi. Omiganan has been assessed in clinical trials as a local treatment for catheter infections (Clinical trial identifier: NCT00231153), atopic dermatitis (NCT03091426), genital warts (NCT02849262), acne vulgaris (NCT02571998), and rosacea (NCT02576847). Pexiganan, a 22-amino-acid analog of the Xenopus peptide magainin, is active against both Gram-positive and Gram-negative bacteria as well as fungi, including several multidrug-resistant microbes. Pexiganan has been evaluated as a topical cream in two phase III clinical trials for the treatment of mild infections in diabetic foot ulcers (NCT01590758, NCT01594762) and additional clinical studies are reportedly planned in cSSSI including surgical wounds, burns, and decubitus ulcers [Citation69]. Similarly to omiganan and pexiganan, DPK-060 (structurally derived from human protein kininogen) also displays a broad spectrum of antimicrobial actions [Citation70–72]. The efficacy of DPK-060 ointment has been studied in clinical phase II trials in the treatment of infections in atopic dermatitis (NCT01522391) [Citation72] and acute external otitis (NCT01447017).
Table 1. List of selected AMPs in clinical phase of development.
In addition to AMPs developed as anti-infectives, a few AMPs are today under late-stage clinical development based on their mechanism-of-action not related to antimicrobial properties. Two of the best characterized examples in this regard are LL-37 and PXL01 – described below.
LL-37 is a 37-meric peptide derived by proteolytic cleavage from the 18-kDa human cathelicidin antimicrobial protein (hCAP18). The initial observation that LL-37 was abundantly present at the wound margin in acute wounds, but was totally lacking in chronic wounds, provided the first evidence that this peptide may be involved in natural wound healing [Citation73,Citation74]. Later studies with experimental animals and in an ex vivo model of human acute wounds have supported a role for LL-37 as a potent enhancer of wound healing [Citation74–79], although the results are not entirely consistent. Increasing the local abundance of LL-37 stimulated wound repair in diabetic and non-diabetic mice [Citation75,Citation78,Citation79] and in mice treated with a corticosteroid to impair normal healing [Citation76] as well as in infected wounds in pigs [Citation77]. However, additional studies performed in diabetic murine wounds failed to show any significant benefit with LL-37 treatment [Citation77]. Reciprocally, antibodies targeting LL-37 inhibited healing in an ex vivo wound healing model composed of organ-cultured human skin [Citation74]. Consistent with nonclinical evidence, a phase I/II clinical trial in patients with chronic leg ulcers revealed that supplementation of LL-37 to nonhealing wounds markedly and significantly increased the healing rate [Citation80]. A phase IIb clinical trial addressing the dose-response efficacy of LL-37 in patients with hard-to-heal venous leg ulcers has recently been initiated (EudraCT: 2018-000536-10). Notably, the MOA of LL-37 to improve the wound repair is not fully elucidated, although this has been suggested to relate to the ability of LL-37 to stimulate re-epithelialization and angiogenesis, and to regulate the inflammatory response [Citation45].
PXL01, derived from the iron-binding glycoprotein lactoferrin present in milk and mucosal secretions, has shown efficacy in a phase II clinical study for prevention of post-operative adhesions in patients going through flexor tendon repair surgery of the hand (NCT01022242) [Citation81]. In vitro, PXL01 was found to suppress the most important hallmarks of adhesion formation by reducing proinflammatory cytokine secretion while promoting fibrinolysis [Citation82]. In addition, PXL01 was shown to regulate the production of the mucinous glycoprotein lubricin, which likely contributes to the adhesion-preventive properties of this peptide [Citation83]. Consistently, in nonclinical proof-of-principle studies, PXL01 efficiently reduced post-surgical adhesion formation in experimental models of abdominal surgery in rats [Citation82] and flexor tendon repair surgery in rabbits [Citation84,Citation85]. Notably, no cytotoxicity of PXL01 was detected in vitro and no negative effects of PXL01 treatment were observed on the healing of bowel anastomosis or the repaired tendons of rats or rabbits, respectively [Citation82,Citation85]. PXL01 was also demonstrated to display microbicidal activity against both Gram-positive and Gram-negative bacteria with a markedly improved potency compared to the parent molecule lactoferrin [Citation82]. A phase III clinical trial addressing the dose-response efficacy of PXL01 in reducing adhesion formation, and correspondingly improving the post-surgical recovery, is now being initiated in patients of hand surgery (EudraCT: 2017-002688-16).
Aside from the direct administration of AMPs as described above, there are also several attempts ongoing to enhance the endogenous production of AMPs. For example, vitamin D3 has been implicated to directly augment the expression of several AMPs, such as hCAP18 (a precursor of LL-37), defensin β2, and neutrophil gelatinase-associated lipocalin (NGAL) [Citation86]. The best studied target for vitamin D3 is the hCAP18 gene, which was demonstrated to be markedly induced by 1,25-dihydroxyvitamin D3 [1,25(OH)2D3, the biologically active form of vitamin D3] in different human cell types in vitro [Citation86]. Topical treatment with 1,25(OH)2D3 also upregulated hCAP18 abundance in human skin in vivo [Citation87]. Furthermore, oral vitamin D supplementation has been shown to increase lesional as well as nonlesional hCAP18/LL-37 levels in skin biopsies from patients with atopic dermatitis [Citation88,Citation89]. In silico analysis has revealed that the induction occurs via a consensus vitamin D response element (VDRE) in the hCAP18 promoter that is activated by vitamin D binding to the vitamin D receptor (VDR) [Citation90]. Notably, microorganisms such as Mycobacterium tuberculosis induce the expression of VDR and vitamin D-1-hydroxylase genes, leading to the production of more 1,25(OH)2D3, which in turn upregulates hCAP18 expression [Citation91]. Importantly, the 1,25(OH)2D3-induced stimulation of antimicrobial activity against M. tuberculosis is critically dependent on the upregulation of hCAP18 gene expression since 1,25(OH)2D3-triggered antimicrobial activity is completely inhibited in the presence of hCAP18-targeting small interfering RNA (siRNA), which instead leads to enhanced intracellular growth of mycobacteria [Citation92]. Given the high prevalence of vitamin D insufficiency, further clinical investigations are warranted to understand whether vitamin D supplementation provides a therapeutic strategy to increase the ability of the host to combat infections by stimulating the production of AMPs.
Strengths and vulnerabilities of AMPs as therapeutic agents
Although a limited number of AMPs are used today in medical practice as anti-infectives, and numerous additional AMPs are currently being evaluated in clinical trials over a broad range of therapeutic areas, there is still considerable discrepancy when comparing several thousands of AMPs claimed to be potential candidate drugs in patent applications or scientific publications with relatively few reported outcomes of the clinical trials. Some liabilities and advantages to bring AMP-based drug candidates into clinical development and market approval are summarized in and commented below [the information extracted from published research on AMPs in PubMed (https://www.ncbi.nlm.nih.gov/pubmed) and the ClinicalTrials.gov registry].
Table 2. Strength-weakness-opportunity-threat (SWOT) analysis of AMPs.
A recent analysis performed on a comprehensive set of data including all peptides that have entered human clinical studies (in total 484 therapeutic peptides) revealed that peptides display higher success rates (defined as the likelihood that a drug candidate will eventually receive regulatory approval at a given phase of development) at all stages of clinical trials compared with small-molecule drugs [Citation93]. This likely reflects the lower safety concerns of peptides [Citation93]. However, it has not been assessed whether different structural or functional classes of peptides have significantly different success rates or which factors may have substantial impact on the probability of success. Identical or similar with endogenous signaling molecules, AMPs provide an opportunity for therapeutic interventions which closely mimics natural pathways. In fact, several AMP-based drugs are principally “replacement therapies” that supplement the peptide at the body locations where the endogenous levels are inadequate/absent (for example supplementation of LL-37 in chronic wounds), and thus, are very unlikely to result in any adverse reaction. The superior safety profiles of AMPs can also be explained by the degradation products of peptides being natural amino acids; moreover, peptides very seldom accumulate in tissues due to their short half-life [Citation94]. Local treatment, which is the most common route of administration for AMPs, further reduces the risk for systemic adverse events since only a minor fraction of the peptide reaches the bloodstream [Citation95]. Notably, therapeutic peptides are considered to be less immunogenic than recombinant proteins and antibodies [Citation96].
In spite of their generally favorable safety profile, AMPs can also, in parallel with microbial membrane affinity, interact and disassemble the membrane of eukaryotic cells, particularly erythrocytes, leading to cytotoxicity (especially hemolysis). This represents a challenge when designing new AMPs and many attempts have been made to decipher the structural features and physicochemical properties that maximize the antimicrobial activity and minimize the cytotoxicity of AMPs. It is known that increased hydrophobicity and/or amphipathicity of AMPs may lead to aggravated hemolysis; however, the relationship between hydrophobicity/amphipathicity and the risk of cytotoxicity appears to be complex and the outcome is not dependent on only one factor but rather requires a balance between charge, hydrophobicity, and amphipathicity, making the theoretical prediction very difficult [Citation97,Citation98]. To experimentally address the risk of cytotoxicity, the selectivity of AMPs is frequently measured by the so-called therapeutic index, defined as the ratio between the minimum hemolytic concentrations (MHC) and minimum inhibitory concentrations (MIC) [Citation98].
One obstacle limiting the clinical application of AMPs (which also applies to peptide therapeutics in general) is the low oral bioavailability. Digestive enzymes which break the amide bonds of ingested proteins are also effective at cleaving these bonds in AMPs. Furthermore, the intestinal permeability of AMPs is limited by their high polarity and molecular weight. Systemic administration of AMPs is also restricted due to the rapid proteolysis in the bloodstream, combined with quick withdrawal from circulation by the kidneys and liver (renal and hepatic clearance, respectively), resulting in a short half-life of the peptide [Citation94,Citation99]. This lability allows the body to rapidly modulate peptide hormone levels to maintain homeostasis but is presenting challenges for therapeutic development. Consequently, although there are examples of AMPs which are aimed for oral or intravenous administration, it is recognized that local application remains the most common delivery route of AMPs (). Notably, even upon local treatment, AMPs are prone to degradation by tissue proteolytic enzymes.
Different strategies to modify the peptide backbone have been described to circumvent the low metabolic stability of AMPs and reduce their susceptibility to proteolytic degradation, such as incorporation of D-amino acids, end-tagging by hydrophobic amino acid stretches, intramolecular cyclization, and blocking N- and/or C-terminal ends of the peptide by N-acetylation, C-amidation, or N-pyroglutamate [Citation72,Citation100–103]. In addition, PEGylation, a process by which a polyethylene glycol chain is added to a biomolecule, is one of the first and most extensively studied approaches to enhance the performance of peptides and many AMPs including magainin and the food preservative nisin have been PEGylated, leading to improved properties [Citation97]. Peptidomimetics, referring to compounds that are designed to mimic a peptide structure, but in which the backbone is not based on α-amino acid residues alone, has also emerged as a promising approach to improve the stability of AMPs in biological matrices [Citation97,Citation104]. Examples of antimicrobial peptidomimetics include peptoids, oligoacyllysines, and β-peptides [Citation97,Citation104] and several peptidomimetic AMPs including LTX-109 (NCT01223222, NCT01803035, and NCT01158235) and PMX30063 (NCT02324335, NCT02052388, and NCT01211470) have to date progressed into clinical development. Importantly, several computational tools have recently been developed to assist in designing and optimizing AMP variants with improved efficacy. Among them, machine-learning methods are frequently used, with the focus on a quantitative structure-activity relationship (QSAR) model, which applies physicochemical descriptors to predict the biological efficacy of peptides from their amino acid sequences [Citation105]. In addition, the de novo computational methods, generating AMP sequences without a seed sequence but using amino acid frequency/position preferences to guarantee certain characteristics, such as load, amphipathicity, and structure, have allowed the production of a large number of peptide variants with a great diversity in amino acid composition as well as 3D structure [Citation105]. Evolutionary algorithms, particularly genetic algorithms, in which the process of evolution is performed in silico through successive generations of mutations and deletions, are also broadly applied to search for AMPs with improved activity [Citation105]. Another main strategy to improve the half-life and performance of AMPs is to use advanced delivery vehicles. Interestingly, the use of nanoparticles for the delivery for AMPs has recently been intensely explored, since nanocarriers provide a large surface area for adsorption/encapsulation of AMPs and also enable to prevent peptide self-aggregation [Citation106–113]. In the opinion of the authors, these efforts are most promising with regard to the local delivery of AMPs, whereas meaningful improvements allowing peroral and systemic delivery still remain challenging.
Regulatory hurdles may considerably delay the development of AMPs, as is the case for any category of pharmaceutical agents, especially in therapeutic indications for which regulatory precedent and well-defined clinical trial endpoints do not exist. Notably, a decline in approvals of new anti-infectives over the recent years, and a rapid rise in resistance toward conventional antibiotics, have led to implementation of several measures by regulatory authorities to accelerate the development of new antimicrobial drugs including an extended period of market exclusivity as well as increased flexibility in the design of clinical trials [Citation114]. Since many AMPs today in clinical pipeline are developed to treat different types of infections, it is the authors’ view, that such initiatives are likely to have a significant impact on facilitating AMPs reaching the market.
Furthermore, due to alarming rise in resistance toward conventional antibiotics, increased research funding has been made available to organizations conducting early-stage research in the anti-infection area both in the United States and the European Union. The largest provider of such incentives is CARB-X, an internationally funded public-private partnership based in Boston that has given more than $100 million to small pharmaceutical companies since it was launched in 2016. However, very few incentives are available for the late-stage clinical development and market access, which represent the costliest part of the development chain for drug developers.
Historically, the relatively high cost of goods sold (COGS) of peptides compared with small molecules has limited the competitiveness of AMPs. Until the late 1990s, most peptide drugs were manufactured recombinantly. Recombinant production can certainly allow for low COGS in the large scale. However, the validation of a GMP-qualified biopharmaceutical production method is associated with steep initial costs, that generally are unattractive to absorb during the early stages of a clinical development program before evidence of clinical effectiveness and safety has been unambiguously demonstrated, applying particularly to small and mid-sized companies. Furthermore, recombinant manufacturing does not allow the use of non-natural amino acid building blocks. Therefore, today, chemical production by Fmoc solid-phase peptide synthesis (SPPS) is the preferred method for peptide manufacturing. Importantly, over the last two decades the cost of amino acid building blocks and solvents has markedly decreased, advanced protection and coupling chemistries have emerged, and production sites have been re-located to low-cost countries [Citation115,Citation116]. There has also been a considerable increase in the length of peptides which can efficiently be chemically synthesized and some service providers (e.g. Genscript) today offer the manufacturing of peptides up to 200 amino acids in length. Mostly, this has been achieved due to the success of applying pseudoproline [Citation117] and backbone protection [Citation118] for the synthesis of long peptides.
It is generally considered that, since SPPS is a stepwise process, errors may become compounded throughout the synthesis. Although this presents an initial challenge in process optimization, it can also provide competitive advantage for innovator companies. Along the path of synthesis route optimization, and the development and validation of analytical methods to assess the peptide purity and the identity/amount of impurities, the manufacturing protocol and analytical process for a specific peptide become highly individualized. This in turn, means that the chemistry, manufacturing, and controls (CMC) dossier of peptide drugs becomes challenging to replicate, which is reflected by a reduced likelihood for generic replacement after patent expiration. According to a strategic report by Giraud [Citation119], the loss of sales due to generic competition only amounted to 15% in the 24 months after the patent expiry of therapeutic peptides as compared to >90% for conventional small-molecule drugs.
Novel approaches for peptide synthesis based on innovations in synthetic biology (e.g. cell-free peptide synthesis using biological extracts and synthetic enzymes) may open the door for more facile and cost-effective production. However, in the author’s view, this field requires further research and commercial maturation before it can be deployed in the setting of AMP production for clinical trials and commercial supply. In summary, the authors believe that SPPS will likely remain as a predominant production method for the manufacturing of AMPs of limited length (<50 amino acids) even in the foreseeable future.
Pricing and reimbursement of antibacterial drugs
The antibacterial drug category has not attracted large interest from conventional pharmaceutical companies in recent decades in spite of an increasing total antibiotic consumption rate (). In fact, it has been pointed out in several analyses that the return-on-investment potential is lower in the anti-infective field than in other therapeutic areas [Citation120]. Among the reasons, high R&D investment needed, short duration of treatment (often 3–10 d), a limited unmet medical need, and significant pricing pressures are frequently cited. Aside from Prevnar (which is a pneumococcal vaccine), no antibacterial drug reached the top-50 list of pharmaceutical products with the highest world-wide sales in 2017 [Citation121].
Box 1. Growth of the anti-bacterial business world-wide. With use of sales data from the IMS Health MIDAS database, Klein et al. [Citation127] estimated that between 2000 and 2015, antibiotic consumption, expressed in defined daily doses (DDD), increased 65% (21–34 billion DDDs), and the antibiotic consumption rate increased 39% (11–16 DDDs per 1000 inhabitants per day). The increase was driven by low- and middle-income countries (mainly China and India). In contrast, in high-income countries, the DDDs per 1000 inhabitants per day decreased by 4% in the same time interval. From a market perspective, this means that the global growth rate is largely driven by increased sales volumes of generic products in low- and middle-income countries. In contrast, the revenue growth rate of the antibacterial products segment on the traditional pharmaceutical market regions (France, Germany, Italy, Japan, Spain, United states, and United Kingdom) is low or negative [Citation128]. The chart illustrates the examples of annual costs for treatment with various common patent-protected prescription medications in the United Kingdom year 2019 showing the cost of 1-year supply of treatment at a standard daily dose. For sofosbuvir, lymecycline, and retapamulin, the annual cost is based on the cost for one completed treatment regimen [Citation129,Citation130].
![Box 1. Growth of the anti-bacterial business world-wide. With use of sales data from the IMS Health MIDAS database, Klein et al. [Citation127] estimated that between 2000 and 2015, antibiotic consumption, expressed in defined daily doses (DDD), increased 65% (21–34 billion DDDs), and the antibiotic consumption rate increased 39% (11–16 DDDs per 1000 inhabitants per day). The increase was driven by low- and middle-income countries (mainly China and India). In contrast, in high-income countries, the DDDs per 1000 inhabitants per day decreased by 4% in the same time interval. From a market perspective, this means that the global growth rate is largely driven by increased sales volumes of generic products in low- and middle-income countries. In contrast, the revenue growth rate of the antibacterial products segment on the traditional pharmaceutical market regions (France, Germany, Italy, Japan, Spain, United states, and United Kingdom) is low or negative [Citation128]. The chart illustrates the examples of annual costs for treatment with various common patent-protected prescription medications in the United Kingdom year 2019 showing the cost of 1-year supply of treatment at a standard daily dose. For sofosbuvir, lymecycline, and retapamulin, the annual cost is based on the cost for one completed treatment regimen [Citation129,Citation130].](/cms/asset/e8640bbc-d08f-44e0-8e29-4be26a5dab4e/ibty_a_1796576_f0004_c.jpg)
The majority of blockbuster products (commonly defined as products with an annual peak-sale in excess of $1 billion) fall into therapeutic areas involving chronic conditions. It is notable, that the sales revenues for all topical antibiotic products aggregated in 2012 were below $1 billion [Citation122]. Thus, many of the novel AMPs developed as topical anti-infectives would be entering this relatively marginal market segment, which largely represents a set of subcritical infections where there is an abundance of commercially available treatments, including prescription and non-prescription antibiotics as well as antiseptic products, such as chlorhexidine, silver nitrate, hydrogen peroxide, and polyhexanide that are sold over-the-counter (OTC).
On most of the traditional pharmaceutical markets, novel antibacterial drugs (including novel AMP-based products) are reimbursed based on each episode of their use and price-referenced against inexpensive generics within hospital directives or national pricing standards. Moreover, due to the threat of escalating antibiotics resistance, regulators have demanded that several new product initiatives are utilized as “spare agents,” i.e. only to be prescribed in rare cases of infection as a last-line resort [Citation123]. Examples of novel products that are restricted to use in life-threatening conditions are tigecycline (Tygacil), levonadifloxacin (Emrok), and cefiderocol (Fetroja) [Citation124]. The indicated uses for these new antibiotics involve complicated intra-abdominal infections, acute bacterial skin and skin structure infections caused by MRSA, and complicated urinary tract infections after treatment failures with other antibiotics, respectively. This current reimbursement model presents a significant economic constraint on value capture for the developer of novel anti-infective AMPs requiring extensive R&D investments.
One may speculate that until these reimbursement dynamics are meaningfully improved and moved toward more value-based pricing, sales revenues will remain insufficient to justify meaningful industry investments. Alternative payment schemes have occasionally been suggested for anti-infectives. For example, the US FDA published a statement proposing a new reimbursement model for antibiotics and antimicrobials to help overcome investment challenges in 2018 [Citation125]. The FDA’s suggestion is that instead of hospitals being reimbursed for antimicrobials on a per-use basis, drug makers would be reimbursed for licenses of certain antimicrobial products aimed for the treatment of multi-drug-resistant infections. Additional initiatives to address these barriers and to ensure a robust antibiotic pipeline have been introduced by nonprofit institutions, national agencies, and WHO (for details, see [Citation126]); however, their appropriateness and value are still largely inconclusive.
Interestingly, based on the inherent pluripotency of AMPs, there are several development initiatives ongoing, where the properties of AMPs not related to their antimicrobial action are explored. Examples that have reached the stage of clinical testing are the use of the lactoferricin derivative PXL01 for the prevention of post-surgical adhesions and the application of LL-37 for stimulation of healing in chronic leg ulcers (). The use of AMPs for noninfectious applications presents an opportunity to bypass the constraints of the traditional antibacterial market segment.
Conclusions
AMPs are unique molecules that possess huge natural diversity and a novel mechanism-of-action, offering alternatives to current anti-infective and immunomodulatory therapies. Although challenges in bringing the nonclinical candidate AMPs into clinical use are recognized, the development of AMPs as therapeutic agents is expected to accelerate in coming years by recent improvements in characterizing their mechanism-of-action, smart formulation strategies enhancing the chemical and metabolic stability, and advanced chemical synthesis protocols lowering manufacturing costs. With a number of AMPs currently going through late-stage clinical development, the authors envision that several new AMP-based drugs will be brought into medical practice in the next decade. As with many other therapeutic areas, new AMP-based investigational drugs reaching the market and demonstrating significant and differentiated medical value could then be transformative on the willingness of corporations and granting agencies to make further investments in the AMP arena.
Disclosure statement
MM is consultant for Promore Pharma AB and JE is employed at Promore Pharma AB, a biopharmaceutical company that develops peptide-based product candidates aimed for the bioactive wound care market.
References
- Di Somma A, Moretta A, Canè C, et al. Antimicrobial and antibiofilm peptides. Biomolecules. 2020;10(4):652.
- Giovati L, Ciociola T, Magliani W, et al. Antimicrobial peptides with antiprotozoal activity: current state and future perspectives. Future Med Chem. 2018;10(22):2569–2572.
- Brice DC, Diamond G. Antiviral activities of human host defense peptides. Curr Med Chem. 2020;27(9):1420–1443.
- Fernández de Ullivarri M, Arbulu S, Garcia-Gutierrez E, et al. Antifungal peptides as therapeutic agents. Front Cell Infect Microbiol. 2020;10:105.
- Yeung AT, Gellatly SL, Hancock RE. Multifunctional cationic host defence peptides and their clinical applications. Cell Mol Life Sci. 2011;68(13):2161–2176.
- Hancock RE, Nijnik A, Philpott DJ. Modulating immunity as a therapy for bacterial infections. Nat Rev Microbiol. 2012;10(4):243–254.
- Hancock RE, Sahl HG. Antimicrobial and host-defense peptides as new anti-infective therapeutic strategies. Nat Biotechnol. 2006;24(12):1551–1557.
- Chen CH, Lu TK. Development and challenges of antimicrobial peptides for therapeutic applications. Antibiotics (Basel). 2020;9(1):24.
- Pasupuleti M, Schmidtchen A, Malmsten M. Antimicrobial peptides: key components of the innate immune system. Crit Rev Biotechnol. 2012;32(2):143–171.
- Nguyen LT, Haney EF, Vogel HJ. The expanding scope of antimicrobial peptide structures and their modes of action. Trends Biotechnol. 2011;29(9):464–472.
- Mwangi J, Hao X, Lai R, et al. Antimicrobial peptides: new hope in the war against multidrug resistance. Zool Res. 2019;40(6):488–505.
- Takahashi D, Shukla SK, Prakash O, et al. Structural determinants of host defense peptides for antimicrobial activity and target cell selectivity. Biochimie. 2010;92(9):1236–1241.
- Jenssen H, Hamill P, Hancock RE. Peptide antimicrobial agents. Clin Microbiol Rev. 2006;19(3):491–511.
- Yeaman MR, Yount NY. Mechanisms of antimicrobial peptide action and resistance. Pharmacol Rev. 2003;55(1):27–55.
- Yan J, Wang K, Dang W, et al. Two hits are better than one: membrane-active and DNA binding-related double-action mechanism of NK-18, a novel antimicrobial peptide derived from mammalian NK-lysin. Antimicrob Agents Chemother. 2013;57(1):220–228.
- Cotter PD, Hill C, Ross RP. Bacteriocins: developing innate immunity for food. Nat Rev Microbiol. 2005;3(10):777–788.
- Schneider VA, Coorens M, Ordonez SR, et al. Imaging the antimicrobial mechanism(s) of cathelicidin-2. Sci Rep. 2016;6:32948.
- Ismail NO, Odendaal C, Serem JC, et al. Antimicrobial function of short amidated peptide fragments from the tick-derived OsDef2 defensin. J Pept Sci. 2019;25(12):e3223.
- Irazazabal LN, Porto WF, Fensterseifer ICM, et al. Fast and potent bactericidal membrane lytic activity of PaDBS1R1, a novel cationic antimicrobial peptide. Biochim Biophys Acta Biomembr. 2019;1861(1):178–190.
- Ebenhan T, Gheysens O, Kruger HG, et al. Antimicrobial peptides: their role as infection-selective tracers for molecular imaging. Biomed Res Int. 2014;2014:867381.
- Malmsten M. Interactions of antimicrobial peptides with bacterial membranes and membrane components. Curr Top Med Chem. 2016;16(1):16–24.
- Teixeira V, Feio MJ, Bastos M. Role of lipids in the interaction of antimicrobial peptides with membranes. Prog Lipid Res. 2012;51(2):149–177.
- Matsuzaki K. Membrane permeabilization mechanisms. Adv Exp Med Biol. 2019;1117:9–16.
- Rončević T, Puizina J, Tossi A. Antimicrobial peptides as anti-infective agents in pre-post-antibiotic era? Int J Mol Sci. 2019;20(22):5713.
- Su Z, Shodiev M, Leitch JJ, et al. Role of transmembrane potential and defects on the permeabilization of lipid bilayers by alamethicin, an ion-channel-forming peptide. Langmuir. 2018;34(21):6249–6260.
- Mescola A, Marín-Medina N, Ragazzini G, et al. Magainin-H2 effects on the permeabilization and mechanical properties of giant unilamellar vesicles. J Colloid Interface Sci. 2019;553:247–258.
- Shahmiri M, Cornell B, Mechler A. Phenylalanine residues act as membrane anchors in the antimicrobial action of Aurein 1.2. Biointerphases. 2017;12(5):05G605.
- Grage SL, Afonin S, Kara S, et al. Membrane thinning and thickening induced by membrane-active amphipathic peptides. Front Cell Dev Biol. 2016;4:65.
- Epand RM. Anionic lipid clustering model. Adv Exp Med Biol. 2019;1117:65–71.
- Lohner K. Membrane-active antimicrobial peptides as template structures for novel antibiotic agents. Curr Top Med Chem. 2017;17(5):508–519.
- Rokitskaya TI, Kolodkin NI, Kotova EA, et al. Indolicidin action on membrane permeability: carrier mechanism versus pore formation. Biochim Biophys Acta. 2011;1808(1):91–97.
- Lai PK, Kaznessis YN. Insights into membrane translocation of protegrin antimicrobial peptides by multistep molecular dynamics simulations. ACS Omega. 2018;3(6):6056–6065.
- Shagaghi N, Palombo EA, Clayton AHA, et al. Antimicrobial peptides: biochemical determinants of activity and biophysical techniques of elucidating their functionality. World J Microbiol Biotechnol. 2018;34(4):62.
- Haney EF, Nathoo S, Vogel HJ, et al. Induction of non-lamellar lipid phases by antimicrobial peptides: a potential link to mode of action. Chem Phys Lipids. 2010;163(1):82–93.
- Cardoso MH, Meneguetti BT, Costa BO, et al. Non-lytic antibacterial peptides that translocate through bacterial membranes to act on intracellular targets. Int J Mol Sci. 2019;20(19):4877.
- Schmidt NW, Wong GC. Antimicrobial peptides and induced membrane curvature: geometry, coordination chemistry, and molecular engineering. Curr Opin Solid State Mater Sci. 2013;17(4):151–163.
- Pfalzgraff A, Brandenburg K, Weindl G. Antimicrobial peptides and their therapeutic potential for bacterial skin infections and wounds. Front Pharmacol. 2018;9:281.
- Brogden KA. Antimicrobial peptides: pore formers or metabolic inhibitors in bacteria? Nat Rev Microbiol. 2005;3(3):238–250.
- Lei J, Sun L, Huang S, et al. The antimicrobial peptides and their potential clinical applications. Am J Transl Res. 2019;11(7):3919–3931.
- Bahar AA, Ren D. Antimicrobial peptides. Pharmaceuticals (Basel). 2013;6(12):1543–1575.
- Chiorean S, Antwi I, Carney DW, et al. Dissecting the binding interactions of teixobactin with the bacterial cell-wall precursor lipid II. Chembiochem. 2020;21(6):789–792.
- Cytryńska M, Rahnamaeian M, Zdybicka-Barabas A, et al. Proline-rich antimicrobial peptides in medicinal maggots of Lucilia sericata interact with bacterial DnaK but do not inhibit protein synthesis. Front Pharmacol. 2020;11:532.
- Mardirossian M, Barrière Q, Timchenko T, et al. Fragments of the nonlytic proline-rich antimicrobial peptide Bac5 kill Escherichia coli cells by inhibiting protein synthesis. Antimicrob Agents Chemother. 2018;62(8):e00534–18.
- van der Does AM, Hiemstra PS, Mookherjee N. Antimicrobial host defence peptides: immunomodulatory functions and translational prospects. Adv Exp Med Biol. 2019;1117:149–171.
- Hancock RE, Haney EF, Gill EE. The immunology of host defence peptides: beyond antimicrobial activity. Nat Rev Immunol. 2016;16(5):321–334.
- Wuerth KC, Falsafi R, Hancock R. Synthetic host defense peptide IDR-1002 reduces inflammation in Pseudomonas aeruginosa lung infection. PLoS One. 2017;12(11):e0187565.
- Wu BC, Lee AH, Hancock R. Mechanisms of the innate defense regulator peptide-1002 anti-inflammatory activity in a sterile inflammation mouse model. J Immunol. 2017;199(10):3592–3603.
- Levast B, Hogan D, van Kessel J, et al. Synthetic cationic peptide IDR-1002 and human cathelicidin LL37 modulate the cell innate response but differentially impact PRRSV replication in vitro. Front Vet Sci. 2019;6:233.
- Freitas CG, Lima SMF, Freire MS, et al. An immunomodulatory peptide confers protection in an experimental candidemia murine model. Antimicrob Agents Chemother. 2017;61(8):e02518–16.
- Silva ON, de la Fuente-Núñez C, Haney EF, et al. An anti-infective synthetic peptide with dual antimicrobial and immunomodulatory activities. Sci Rep. 2016;6:35465.
- Shao C, Li W, Tan P, et al. Symmetrical modification of minimized dermaseptins to extend the spectrum of antimicrobials with endotoxin neutralization potency. Int J Mol Sci. 2019;20(6):1417.
- Petrlova J, Hansen FC, van der Plas MJA, et al. Aggregation of thrombin-derived C-terminal fragments as a previously undisclosed host defense mechanism. Proc Natl Acad Sci USA. 2017;114(21):E4213–E4222.
- Saravanan R, Holdbrook DA, Petrlova J, et al. Structural basis for endotoxin neutralisation and anti-inflammatory activity of thrombin-derived C-terminal peptides. Nat Commun. 2018;9(1):2762.
- Park YJ, Park B, Lee M, et al. A novel antimicrobial peptide acting via formyl peptide receptor 2 shows therapeutic effects against rheumatoid arthritis. Sci Rep. 2018;8(1):14664.
- Marques-Neto LM, Trentini MM, das Neves RC, et al. Antimicrobial and chemotactic activity of scorpion-derived peptide, ToAP2, against Mycobacterium massiliensis. Toxins (Basel). 2018;10(6):219.
- Mi B, Liu J, Liu Y, et al. The designer antimicrobial peptide A-hBD-2 facilitates skin wound healing by stimulating keratinocyte migration and proliferation. Cell Physiol Biochem. 2018;51(2):647–663.
- Peschel A, Sahl HG. The co-evolution of host cationic antimicrobial peptides and microbial resistance. Nat Rev Microbiol. 2006;4(7):529–536.
- Fjell CD, Hiss JA, Hancock RE, et al. Designing antimicrobial peptides: form follows function. Nat Rev Drug Discov. 2011;11(1):37–51.
- Koo HB, Seo J. Antimicrobial peptides under clinical investigation. Pept Sci. 2019;111(5):e24122.
- Spohn R, Daruka L, Lázár V, et al. Integrated evolutionary analysis reveals antimicrobial peptides with limited resistance. Nat Commun. 2019;10(1):4538.
- Tucker AT, Leonard SP, DuBois CD, et al. Discovery of next-generation antimicrobials through bacterial self-screening of surface-displayed peptide libraries. Cell. 2018;172(3):618–628. e613.
- Ling LL, Schneider T, Peoples AJ, et al. A new antibiotic kills pathogens without detectable resistance. Nature. 2015;517(7535):455–459.
- Lofton H, Pränting M, Thulin E, et al. Mechanisms and fitness costs of resistance to antimicrobial peptides LL-37, CNY100HL and wheat germ histones. PLoS One. 2013;8(7):e68875.
- Kubicek-Sutherland JZ, Lofton H, Vestergaard M, et al. Antimicrobial peptide exposure selects for Staphylococcus aureus resistance to human defence peptides. J Antimicrob Chemother. 2017;72(1):115–127.
- Andersson DI, Hughes D, Kubicek-Sutherland JZ. Mechanisms and consequences of bacterial resistance to antimicrobial peptides. Drug Resist Updat. 2016;26:43–57.
- Mahlapuu M, Håkansson J, Ringstad L, et al. Antimicrobial peptides: an emerging category of therapeutic agents. Front Cell Infect Microbiol. 2016;6:194.
- Stevenson CL. Advances in peptide pharmaceuticals. Curr Pharm Biotechnol. 2009;10(1):122–137.
- Zavascki AP, Goldani LZ, Li J, et al. Polymyxin B for the treatment of multidrug-resistant pathogens: a critical review. J Antimicrob Chemother. 2007;60(6):1206–1215.
- Sierra JM, Fuste E, Rabanal F, et al. An overview of antimicrobial peptides and the latest advances in their development. Expert Opin Biol Ther. 2017;17(6):663–676.
- Boge L, Umerska A, Matougui N, et al. Cubosomes post-loaded with antimicrobial peptides: characterization, bactericidal effect and proteolytic stability. Int J Pharm. 2017;526(1–2):400–412.
- Nordström R, Nyström L, Andren OCJ, et al. Membrane interactions of microgels as carriers of antimicrobial peptides. J Colloid Interface Sci. 2018;513:141–150.
- Håkansson J, Ringstad L, Umerska A, et al. Characterization of the in vitro, ex vivo, and in vivo efficacy of the antimicrobial peptide DPK-060 used for topical treatment. Front Cell Infect Microbiol. 2019;9:174.
- Rivas-Santiago B, Rivas Santiago CE, Castaneda-Delgado JE, et al. Activity of LL-37, CRAMP and antimicrobial peptide-derived compounds E2, E6 and CP26 against Mycobacterium tuberculosis. Int J Antimicrob Agents. 2013;41(2):143–148.
- Heilborn JD, Nilsson MF, Kratz G, et al. The cathelicidin anti-microbial peptide LL-37 is involved in re-epithelialization of human skin wounds and is lacking in chronic ulcer epithelium. J Invest Dermatol. 2003;120(3):379–389.
- Carretero M, Escamez MJ, Garcia M, et al. In vitro and in vivo wound healing-promoting activities of human cathelicidin LL-37. J Invest Dermatol. 2008;128(1):223–236.
- Ramos R, Silva JP, Rodrigues AC, et al. Wound healing activity of the human antimicrobial peptide LL37. Peptides. 2011;32(7):1469–1476.
- Steinstraesser L, Hirsch T, Schulte M, et al. Innate defense regulator peptide 1018 in wound healing and wound infection. PLoS One. 2012;7(8):e39373.
- Kos S, Vanvarenberg K, Dolinsek T, et al. Gene electrotransfer into skin using noninvasive multi-electrode array for vaccination and wound healing. Bioelectrochemistry. 2017;114:33–41.
- Steinstraesser L, Lam MC, Jacobsen F, et al. Skin electroporation of a plasmid encoding hCAP-18/LL-37 host defense peptide promotes wound healing. Mol Ther. 2014;22(4):734–742.
- Grönberg A, Mahlapuu M, Ståhle M, et al. Treatment with LL-37 is safe and effective in enhancing healing of hard-to-heal venous leg ulcers: a randomized, placebo-controlled clinical trial. Wound Repair Regen. 2014;22(5):613–621.
- Wiig ME, Dahlin LB, Friden J, et al. PXL01 in sodium hyaluronate for improvement of hand recovery after flexor tendon repair surgery: randomized controlled trial. PLoS One. 2014;9(10):e110735.
- Nilsson E, Björn C, Sjöstrand V, et al. A novel polypeptide derived from human lactoferrin in sodium hyaluronate prevents postsurgical adhesion formation in the rat. Ann Surg. 2009;250(6):1021–1028.
- Edsfeldt S, Holm B, Mahlapuu M, et al. PXL01 in sodium hyaluronate results in increased PRG4 expression: a potential mechanism for anti-adhesion. Ups J Med Sci. 2017;122(1):28–34.
- Håkansson J, Mahlapuu M, Ekström L, et al. Effect of lactoferrin peptide (PXL01) on rabbit digit mobility after flexor tendon repair. J Hand Surg Am. 2012;37(12):2519–2525.
- Wiig M, Olmarker K, Håkansson J, et al. A lactoferrin-derived peptide (PXL01) for the reduction of adhesion formation in flexor tendon surgery: an experimental study in rabbits. J Hand Surg Eur Vol. 2011;36(8):656–662.
- Wang TT, Nestel FP, Bourdeau V, et al. Cutting edge: 1,25-dihydroxyvitamin D3 is a direct inducer of antimicrobial peptide gene expression. J Immunol. 2004;173(5):2909–2912.
- Weber G, Heilborn JD, Chamorro Jimenez CI, et al. Vitamin D induces the antimicrobial protein hCAP18 in human skin. J Invest Dermatol. 2005;124(5):1080–1082.
- Albenali LH, Danby S, Moustafa M, et al. Vitamin D and antimicrobial peptide levels in patients with atopic dermatitis and atopic dermatitis complicated by eczema herpeticum: a pilot study. J Allergy Clin Immunol. 2016;138(6):1715–1719. e1714.
- Hata TR, Kotol P, Jackson M, et al. Administration of oral vitamin D induces cathelicidin production in atopic individuals. J Allergy Clin Immunol. 2008;122(4):829–831.
- Chen X, Zou X, Qi G, et al. Roles and mechanisms of human cathelicidin LL-37 in cancer. Cell Physiol Biochem. 2018;47(3):1060–1073.
- Liu PT, Stenger S, Li H, et al. Toll-like receptor triggering of a vitamin D-mediated human antimicrobial response. Science. 2006;311(5768):1770–1773.
- Liu PT, Stenger S, Tang DH, et al. Cutting edge: vitamin D-mediated human antimicrobial activity against Mycobacterium tuberculosis is dependent on the induction of cathelicidin. J Immunol. 2007;179(4):2060–2063.
- Lau JL, Dunn MK. Therapeutic peptides: historical perspectives, current development trends, and future directions. Bioorg Med Chem. 2018;26(10):2700–2707.
- Vlieghe P, Lisowski V, Martinez J, et al. Synthetic therapeutic peptides: science and market. Drug Discov Today. 2010;15(1–2):40–56.
- U.S. Department of Health and Human Services Food and Drug Administration. Guidance for industry chronic cutaneous ulcer and burn wounds – developing products for treatment. Silver Spring (MD): U.S. Department of Health and Human Services Food and Drug Administration; 2006.
- McGregor DP. Discovering and improving novel peptide therapeutics. Curr Opin Pharmacol. 2008;8(5):616–619.
- Kumar P, Kizhakkedathu JN, Straus SK. Antimicrobial peptides: diversity, mechanism of action and strategies to improve the activity and biocompatibility in vivo. Biomolecules. 2018;8(1):4.
- Maturana P, Martinez M, Noguera ME, et al. Lipid selectivity in novel antimicrobial peptides: implication on antimicrobial and hemolytic activity. Colloids Surf B Biointerfaces. 2017;153:152–159.
- Di L. Strategic approaches to optimizing peptide ADME properties. AAPS J. 2015;17(1):134–143.
- Björn C, Mahlapuu M, Mattsby-Baltzer I, et al. Anti-infective efficacy of the lactoferrin-derived antimicrobial peptide HLR1r. Peptides. 2016;81:21–28.
- Zhao Y, Zhang M, Qiu S, et al. Antimicrobial activity and stability of the D-amino acid substituted derivatives of antimicrobial peptide polybia-MPI. AMB Express. 2016;6(1):122.
- Andreev K, Martynowycz MW, Ivankin A, et al. Cyclization improves membrane permeation by antimicrobial peptoids. Langmuir. 2016;32(48):12905–12913.
- Liu B, Zhang W, Gou S, et al. Intramolecular cyclization of the antimicrobial peptide Polybia-MPI with triazole stapling: influence on stability and bioactivity. J Pept Sci. 2017;23(11):824–832.
- Molchanova N, Hansen PR, Franzyk H. Advances in development of antimicrobial peptidomimetics as potential drugs. Molecules. 2017;22(9):1430.
- Cardoso MH, Orozco RQ, Rezende SB, et al. Computer-aided design of antimicrobial peptides: are we generating effective drug candidates? Front Microbiol. 2019;10:3097.
- Nordström R, Malmsten M. Delivery systems for antimicrobial peptides. Adv Colloid Interface Sci. 2017;242:17–34.
- Braun K, Pochert A, Linden M, et al. Membrane interactions of mesoporous silica nanoparticles as carriers of antimicrobial peptides. J Colloid Interface Sci. 2016;475:161–170.
- Groo AC, Matougui N, Umerska A, et al. Reverse micelle-lipid nanocapsules: a novel strategy for drug delivery of the plectasin derivate AP138 antimicrobial peptide. Int J Nanomedicine. 2018;13:7565–7574.
- Matougui N, Boge L, Groo AC, et al. Lipid-based nanoformulations for peptide delivery. Int J Pharm. 2016;502(1–2):80–97.
- Matougui N, Groo AC, Umerska A, et al. A comparison of different strategies for antimicrobial peptides incorporation onto/into lipid nanocapsules. Nanomedicine (Lond). 2019;14(13):1647–1662.
- Nordström R, Andren OCJ, Singh S, et al. Degradable dendritic nanogels as carriers for antimicrobial peptides. J Colloid Interface Sci. 2019;554:592–602.
- Umerska A, Cassisa V, Bastiat G, et al. Synergistic interactions between antimicrobial peptides derived from plectasin and lipid nanocapsules containing monolaurin as a cosurfactant against Staphylococcus aureus. Int J Nanomedicine. 2017;12:5687–5699.
- Umerska A, Matougui N, Groo AC, et al. Understanding the adsorption of salmon calcitonin, antimicrobial peptide AP114 and polymyxin B onto lipid nanocapsules. Int J Pharm. 2016;506(1–2):191–200.
- Fox JL. Antimicrobial peptides stage a comeback. Nat Biotechnol. 2013;31(5):379–382.
- Behrendt R, White P, Offer J. Advances in Fmoc solid-phase peptide synthesis. J Pept Sci. 2016;22(1):4–27.
- DePalma A. Peptides: new processes, lower costs. Genet Eng Biotechnol News. 2015;35(13):7–8.
- White P, Keyte JW, Bailey K, et al. Expediting the Fmoc solid phase synthesis of long peptides through the application of dimethyloxazolidine dipeptides. J Pept Sci. 2004;10(1):18–26.
- Cardona V, Eberle I, Barthelemy S, et al. Application of DMB‐dipeptides in the Fmoc SPPS of difficult and aspartimide‐prone sequences. Int J Pept Res Ther. 2008;14(4):285–292.
- Giraud M. Peptide market overview. Chemical process development conference. 2012. Lonza, Basel.
- Payne DJ, Miller LF, Findlay D, et al. Time for a change: addressing R&D and commercialization challenges for antibacterials. Philos Trans R Soc Lond B Biol Sci. 2015;370(1670):20140086.
- Iervolino A, Urqhart L. World preview 2017, outlook to 2022. 10 ed. Evaluate pharma. 2017. Available from: http://info.evaluategroup.com/rs/607-YGS-364/images/WP17.pdf
- LytixBiopharma. LTX-109. 2013. Available from: http://www.lytixbiopharma.com/uploads/LTX-109%20slides_Aug%202013.pdf
- World Health Organization (WHO). In the face of slow progress, WHO offers a new tool and sets a target to accelerate action against antimicrobial resistance. 2019. Available from: https://www.who.int/news-room/detail/18-06-2019-in-the-face-of-slow-progress-who-offers-a-new-tool-and-sets-a-target-to-accelerate-action-against-antimicrobial-resistance
- Fischer K. FDA approves new drug for UTI: what to know. Healthline. 2019. Available from: https://www.healthline.com/health-news/fda-approves-new-drug-for-uti
- Gottlieb S. Statement from FDA commissioner Scott Gottlieb, M.D., on FDA’s efforts to foster discovery and development of new tools to fight antimicrobial-resistant infections. Silver Spring (MD): US Food and Drug Administration; 2018.
- O’Neill J. Tackling drug-resistant infections globally: final report and recommendations. The review on antimicrobial resistance. 2016. Available from: https://amr-review.org/sites/default/files/160525_Final%20paper_with%20cover.pdf
- Klein EY, Van Boeckel TP, Martinez EM, et al. Global increase and geographic convergence in antibiotic consumption between 2000 and 2015. Proc Natl Acad Sci USA. 2018;115(15):E3463–E3470.
- Visiongain. Global antibacterial drugs market 2017–2027: cephalosporins, penicillins, fluoroquinolones, macrolides, carbapenems and others. London: Visiongain; 2017.
- Regional Drug and Therapeutics Centre (RDTC). Cost comparison charts. 16/17. Framlington place, Newcastle upon Tyne, NE2 4AB. 2019. Available from: http://gmmmg.nhs.uk/docs/cost_comparison_charts.pdf
- National Institute for Health and Care Excellence (NICE). 2019. BMJ Group, London, UK. Available from: https://www.nice.org.uk/bnf-uk-only