Abstract
Streptomyces is the largest and most significant genus of Actinobacteria, comprising 961 species. These Gram-positive bacteria produce many versatile and important bioactive compounds; of these, antibiotics, specifically the enhancement or activation of their production, have received extensive research attention. Recently, various biotic and abiotic elicitors have been reported to modify the antibiotic metabolism of Streptomyces, which promotes the production of new antibiotics and bioactive metabolites for improvement in the yields of endogenous products. However, some elicitors that obviously contribute to secondary metabolite production have not yet received sufficient attention. In this study, we have reviewed the functions and mechanisms of chemicals, novel microbial metabolic elicitors, microbial interactions, enzymes, enzyme inhibitors, environmental factors, and novel combination methods regarding antibiotic production in Streptomyces. This review has aimed to identify potentially valuable elicitors for stimulating the production of latent antibiotics or enhancing the synthesis of subsistent antibiotics in Streptomyces. Future applications and challenges in the discovery of new antibiotics and enhancement of existing antibiotic production using elicitors are discussed.
Introduction
Although microbial secondary metabolites are not essential for cell growth, they are important for human health, nutrition, and various economic activities, and they are usually produced during the late growth phase [Citation1]. To date, tens of thousands of natural products, representing only a small proportion of all bioactive metabolites, have been discovered, among which the most important secondary metabolites have proven to be antibiotics. Nearly two-thirds of antibiotics are produced by actinomycetes, mainly by Streptomyces species, and antibiotic enhancement or activation in Streptomyces has been a research hotspot [Citation2,Citation3]. Genome sequencing of S. coelicolor A3(2) [Citation4], S. avermitilis [Citation5], S. gilvosporeus [Citation6], and S. griseus [Citation7] indicated that each species contains gene clusters responsible for the synthesis of ≥20 potential secondary metabolites. However, the biosynthesis of only a few of these metabolites is activated. Antibiotic resistance and its wider implications represents a growing healthcare crisis, and new antibacterial compounds, particularly antibiotics, are urgently needed [Citation8,Citation9].
Generally, the biosynthesis of microbial antibiotics is charged by gene clusters, numerous of which comprise several transcription units [Citation10]. Various types of regulatory genes, including pathway-specific regulatory genes, work in different ways to affect the gene cluster expressions and the cognate antibiotic productions [Citation11]. Approaches to improve the productivity of industrial microorganisms range from classical random mutagenesis, genetic engineering [Citation12,Citation13], and optimization of large-scale fermentation to more rational protein design and heterologous host expression [Citation11,Citation14–16]. As typical secondary metabolites, antibiotics are usually synthesized at the late growth phase of the producing microorganisms. Gene expression exhibits temporal changes, and it can also be influenced greatly by environmental manipulation. In addition, the coordination of primary and secondary metabolism occurs through multiple interactions between pleiotropic and cluster-situated transcriptional factors [Citation1,Citation17].
Natural product discovery has been divided into three generations: the first 30 years (1940s–1970s) of phenotypic screening, the second 30 years (1970s–2000s) of knowledge-based approaches, and genomics-based approaches (2000s and beyond) [Citation18]. Recently, research on antibiotic enhancement or activation in Streptomyces has focused on elicitors that activate gene cluster expression [Citation2,Citation16], which was also divided into “unselective” and “selective” strategies by Nodwell [Citation19]. Elicitation has been widely applied to enhance the production of bioactive molecules in plants [Citation20,Citation21]. Elicitors, such as nutrient sources including: carbon, nitrogen, and phosphorous (summarized by Romero-Rodríguez et al. [Citation22] and van Wezel [Citation10,Citation23]), microbial metabolic elicitors (γ-butyrolactones, γ-butenolides, PI factor, diketopiperazine, and furans; summarized by Daniel-Ivad et al. [Citation24]), exogenous small molecules (summarized by Okada and Seyedsayamdost [Citation25]) and signaling molecules (summarized by Niu and Li [Citation26,Citation27]) have been reported to activate cryptic pathways and enhance antibiotic production in Streptomyces. As new concepts for discovery of natural products, elicitors combined with proteomics, ecology, and genomics have raised concerns (summarized by van Wezel [Citation10,Citation28,Citation29]).
However, many other potential elicitors, such as chemicals, novel microbial metabolic elicitors, microbial interactions, enzymes, enzyme inhibitors, environmental factors, and combination modalities based on elicitors, can also exert regulatory effects in Streptomyces. In this review, we summarized the neglected elicitors that stimulate the production of latent antibiotics or enhance the synthesis of subsistent antibiotics, and we also discussed the mechanisms by which antibiotic production is activated in Streptomyces.
Chemical elicitors activate or enhance antibiotic production in Streptomyces
Chemical elicitors are considered widely applicable for enhancing the production of secondary metabolites and potentiating the yields of cryptic secondary metabolites in Actinobacteria [Citation30]. Chemical molecules have also been reported to be valid for altering the production of antibiotics in Streptomyces [Citation31–33]. In this section, we will summarize the chemical elicitors that activate or enhance antibiotic production in Streptomyces on the basis of recent research findings.
Rare earth elements (REEs) activate the expression of gene clusters involved in antibiotic biosynthesis
Metals such as Fe, Co, Cu, Mn, Ni, Zn, Mg, and Vanadium (V) are essential micronutrients for microbial metabolism, and they can act as effectors of secondary metabolite production, as summarized by Locatelli [Citation34]. In addition, Ni2+ and Fe2+ were also demonstrated to drive antimicrobial melanin production by Streptomyces sp. ZL-24 [Citation35]. In this study, we focused on the effect of REEs, a general term for 17 elements including scandium (Sc), yttrium (Y), and the lanthanides (La) that have been widely used in high-technology products [Citation36] ().
REEs appear to represent a forceful stress stimulus that activates antibiotic synthesis. Kawai and Ochi [Citation37] reported that Sc increases antibiotic production by 2–25-fold when added at 10–100 μM to cultures of S. coelicolor A3(2) (actinorhodin [ACT] production increased by 25-fold), S. antibioticus (ACT production enhanced by 2-fold), and S. griseus (streptomycin production enhanced by nearly 4-fold). More interestingly, although the ACT synthesis gene is silent in S. lividans, the addition of Sc at a sublethal concentration (one-third of the minimal inhibitory concentration) to the culture medium activates ACT synthesis. Subsequently, the effects of REEs on ACT production were tested, and all examined REEs effectively enhanced ACT synthesis in S. coelicolor A3(2) [Citation36]. Then, Shentu et al. [Citation38] confirmed that the addition of a small amount (5–20 μM) of Sc also enhances toyocamycin production in S. diastatochromogenes SD3145, and La supplementation increased tacrolimus production by 1.3-fold in S. tsukubaensis [Citation39].
Several studies have attempted to clarify the mechanism, by which REEs enhance antibiotic synthesis. The first classified factor is guanosine 5′-diphosphate 3′-diphosphate (ppGpp), which is synthesized in the ribosome and which is essential for ACT overproduction through its function as a bacterial alarmone [Citation40]. Kawai et al. found that Sc provoked basal-level ppGpp to guarantee ACT overproduction in S. coelicolor [Citation37]. Moreover, macromolecules such as RNA polymerase and ribosomes are proposed to be the targets through which REEs activate cryptic genes [Citation41]. According to transcriptional analysis, the expression of nine genes belonging to nine secondary metabolite biosynthesis gene clusters was activated by 2.5–12-folds using optimal concentrations of Sc and La (200 and 1900 μM, respectively) in S. coelicolor A3(2). In particular, expression of the ACT cluster activator actII-ORF4 and prodiginines transcriptional regulator redD were increased to 12- and 4-folds, respectively [Citation36]. Interestingly, Streptomyces species, such as S. levoris and S. flavoviridis, were found to have the capacity to accumulate europium and samarium [Citation42]. Streptomyces albus can accumulate lutetium in greater quantities than other REEs [Citation43]. The mechanism of this accumulation is indistinct, but binding of metal and the strong negative charge (conferred by teichoic acid polymers) on the surface of cell walls may change the microenvironment around cells, after which the signal of pH alteration offers the possibility of modifying synthesis of antibiotic. Moreover, because REEs are distributed ubiquitously in soil, acquisition of the ability to respond to low levels of REEs and adapt their physiology to prevailing conditions around microorganisms is another possible mechanism of antibiotic synthesis activation [Citation41].
Global control is confirmed to be an effective strategy for activating specific gene clusters, as summarized by van Wezel [Citation44]. Although the mechanism of action remains to be clarified, the aforementioned works revealed the global regulatory effects of REEs on antibiotic production and highlighted their enormous potential for antibiotic gene activation and enhancement. Based on the connection between REEs and microbiology, “rare earth microbiology,” as defined by Kawai and Ochi [Citation41], may thus offer a new approach to induce or stimulate antibiotic production in Streptomyces. Moreover, this method displays advantages across a wide range of effective concentrations without the use of gene engineering technology. Together with other methods, such as drug resistance mutations [Citation45], pathway-specific regulatory modification [Citation46], and genomic sequencing [Citation47], rare earth microbiology may represent a powerful tool for activating silent or poorly expressed genes to facilitate the discovery of novel biologically active compounds or increase the production of existing antibiotics. Moreover, although metals such as Fe, Zn, Cu, Mg, and Ni are essential for enzymatic activity [Citation48], high concentrations of these metals are highly toxic. Thus, the concentrations of most metals (both essential and non-essential) including REEs must be precisely controlled during the biosynthesis of antibiotics in Streptomyces.
Dimethyl sulfoxide (DMSO) and ethanol induce the deviation of metabolic flux
In the presence of different concentrations of DMSO, the production of tylosin in S. fradiae [Citation49], chloramphenicol in S. venezuelae, tetracenomycin C in S. glaucescens, prodigiosin in S. lividans, and ascomycin in S. hygroscopicus was increased, and this phenomenon was termed the “DMSO effect” [Citation50,Citation51]. Further research indicated that the DMSO concentration is vital for determining the secondary metabolite profile. For example, thiostrepton production is increased by approximately 2-fold in S. azureus ATCC14921 in the presence of 3–5% DMSO, whereas the chloramphenicol yield is reduced and the tetracenomycin C level is unchanged in the presence of up to 5% DMSO [Citation50]. Wang et al. found that DMSO alone or combined with LaCl3 can increase tacrolimus (FK506) accumulation by more than 30% in S. tsukubaensis [Citation39]. Furthermore, the addition of DMSO and furfural resulted in a 33% increase in biomass and a 27% increase in undecylprodigiosin (RED) production [Citation52].
Metabolomic analysis in S. tsukubaensis revealed the enhancement of precursors such as methoxymalonyl-CoA, (4R,5R)-4,5-dihydroxycyclohex-1-enecarboxylic acid, methylmalonyl-CoA, malonyl-CoA, allylmalonyl-CoA, CoA-ester, and pipecolic acid. The enhancement of precursor synthesis via the pentose phosphate and glycolysis and through amino acid metabolism was revealed to be the main contributor to FK506 overproduction [Citation39]. In S. hygroscopicus, the pathways responding to DMSO, such as aromatic amino acid biosynthesis and the pentose phosphate pathway, similarly affected the synthesis of ascomycin (FK520), a 23-membered polyketide macrolide and analog of FK506 that also exhibits antifungal and immunosuppressive activity [Citation51]. Meanwhile, DMSO redirects carbon flux from the tricarboxylic acid (TCA) cycle and pyruvate metabolism toward pyruvate [Citation39]. Notably, during FK506 and FK520 synthesis [Citation53], DMSO demonstrates a more potent effect than other chemicals, including γ-butyrolactone, which is a powerful elicitor of various secondary metabolites produced by Streptomyces [Citation24].
Ethanol can also stimulate secondary metabolism or antibiotic synthesis. Tetracenomycin C production in S. glaucescens is stimulated by 1–3% ethanol, but the same concentration of ethanol inhibits chloramphenicol production in S. venezuelae. Sekurova et al. [Citation54] found that 6% ethanol activates chloramphenicol and jadomycin biosynthesis, consistent with a report by Doull et al. [Citation55] on S. venezuelae ATCC 10712 (ISP5230). Nevertheless, low ethanol concentrations also affect the synthesis of antibiotics by Streptomyces. For example, validamycin A (Val-A) production in S. hygroscopicus is greatly enhanced by <10 mM ethanol, which increases the transcription of eight Val-A structural genes [Citation56].
The effects of ethanol on overall metabolic flow or specific targets in antibiotic synthesis differs among various Streptomyces species and even appears to occur via different mechanisms. Notably, in S. venezuelae ATCC 10712 (ISP5230), ethanol can enhance chloramphenicol biosynthesis via the positive regulation of JadR1 [54]; contrarily, JadR1 is postulated to be a repressor of the chloramphenicol biosynthetic pathway [Citation57]. The promoter cmlRp is silent in maltose- and yeast extract-rich medium, and it does not respond to ethanol. This suggests that the activity of ethanol as a positive or negative regulator depends on the bacterial growth conditions [Citation57]. Nevertheless, the effect of ethanol on the signal transduction cascade in Streptomyces may also be influenced by intracellular reactive oxygen species (ROS) signaling, global regulators such as AfsR and GlnR that participate in the regulation of Val-A biosynthesis, enhancement of the activity of glucose-6-phosphate dehydrogenase and the inhibition of glyceraldehyde 3-phosphate dehydrogenase [Citation56]. Furthermore, the synthesis of the autoregulator γ-butyrolactone, was suppressed by (R)-paraconyl alcohol, implying a new mechanism by which ethanol regulates antibiotic production [Citation58].
To summarize, DMSO and ethanol can significantly affect the types and levels of antibiotics produced by Streptomyces. Pleiotropic or global transcriptional regulation appears to be the pathway induced by both DMSO and ethanol. In addition, DMSO mainly alters the metabolic flux toward primary metabolism (pentose phosphate pathway, glycolysis, and TCA cycle) to offer more precursors to “push” the synthesis of antibiotics. Conversely, ethanol appears to preferentially stimulate pathway-specific transcriptional regulation and oxidative stress reactions to “pull” the production of antibiotics (). Although this regulation is indirect controlled and the regulatory mechanisms require further research for elucidation, DMSO and ethanol offer novel but easily controllable and economical approaches to optimizing antibiotic production by Streptomyces.
Figure 1. The elicitors DMSO and ethanol and their regulation of antibiotic production in Streptomyces. DMSO and ethanol are effective elicitors of secondary metabolism in Streptomyces and exert their effects by changing the membrane structure, affecting steady-state growth, and regulating associated genes and carbon metabolism. A-CoA: acetyl-CoA; Am-CoA: allylmalonyl-CoA; CIT: citrate; FK506: tacrolimus; DMSO: dimethyl sulfoxide; FK520: ascomycin; Glu-6-P: glucose 6-phosphate; HK-RR: histidine kinase–response regulator; Ile: isoleucine; M-CoA: malonyl-CoA; MET: methionine; MM-CoA: methylmalonyl-CoA; MOM-CoA: methoxymalonyl-CoA; OAA: oxaloacetate; P-CoA: propionyl-CoA; PEP: phosphoenolpyruvic acid; SP: sedoheptulose 7-phosphate; SUCC: succinic acid; TCA: tricarboxylic acid; Val-A: validamycin A; 1,3-DPG: 1,3-bisphosphoglyceric acid.
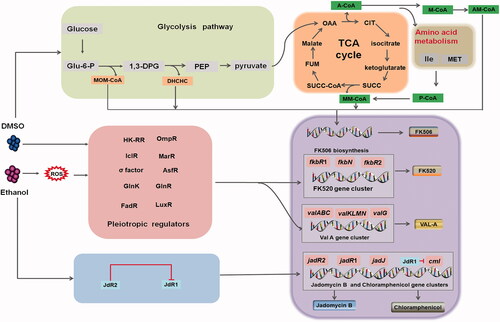
Nanoparticles regulate gene expression and primary metabolic pathways
Generally, nanoparticles have toxic effects on microorganisms [Citation59–62]. However, the effects of nanoparticles on antibiotic metabolite synthesis in Streptomyces has recently attracted increasing attention ().
Liu et al. demonstrated a higher yield of antibiotics in S. coelicolor M145 after treating cultures with smaller CuO nanoparticles [Citation62,Citation63], Al2O3 nanoparticles [Citation64], and carbon nanomaterials [Citation65]. When S. coelicolor M145 was exposed to 10 mg/L 40-nm CuO nanoparticles, ACT synthesis was improved 2-fold. However, ACT synthesis was inhibited when the concentration of CuO nanoparticles exceeded 20 mg/L. Following treatment with Al2O3 nanoparticles, the yield of RED and ACT increased by as much as 3.7- and 4.6-folds, respectively, in the presence of 1000 mg/L 80-nm CuO nanoparticles, exceeding the effects of 100 mg/L CuO nanoparticles [Citation64]. When the particle size of biochar was reduced to 133–170 nm within the nanometer range via ball milling, ball-milled biochar was formed. After biochar was added to the fermentation broth at 10 mg/L, the yields of RED and ACT were increased by 3.2- and 2.9-folds, respectively, accompanied by massive cell disruption, but the production of RED and ACT started 4 and 24 h earlier, respectively, than observed in the control [Citation65]. In addition to the concentration of nanoparticles, the particle size of Al2O3 also affects antibiotic production by S. coelicolor M145. In the presence of 1000 mg/L 80-nm Al2O3 nanoparticles, the maximum RED concentration of 3.7 mg/L was observed after 72 h, and the concentration exceeded that in the presence of 30-nm Al2O3 nanoparticles [Citation64].
The regulatory mechanisms underlying the effects of different nanoparticles on secondary metabolism in S. coelicolor M145 appears to substantially vary [Citation62]. Cu2+ released from CuO nanoparticles promotes the production of acetyl-CoA via primary metabolic pathways that are also mediated by enhanced ROS levels. This metal alters the expression of several genes in the ACT cluster, which may in turn affect the expression of antibiotic synthesis genes. CuO and Al2O3 nanoparticles have no significant effect on actII-ORF4 gene expression [Citation63]; however, Al2O3 nanoparticles can increase the expression of antibiotic biosynthetic genes and two-component systems (such as SCO4229/SCO4230) inhibit primary metabolic pathways, thereby affecting antibiotic synthesis.
Thus, the scant available research suggests a phenomenon in which the addition of nanoparticles, enhancement of antibiotics synthesis, and increased accumulation of ROS occurs simultaneously in Streptomyces. This indicated that the regulatory effects of nanoparticles may depend on the oxidative stress regulatory pathway, which is a global regulatory system that controls the primary and the secondary metabolism (additional details are presented in ROS section). Although few reports have described the effects of nanoparticles on antibiotic synthesis in Streptomyces and the associated mechanisms require further investigation, nanoparticles have potential utility as effective additives for increasing antibiotic yields [Citation64].
Enzymes and enzyme inhibitors activate or enhance the antibiotic synthesis in Streptomyces
Metabolite biosynthesis depends on the orchestrated regulation of the biomachineries involved in the translation of DNA into working proteins [Citation66]. In Streptomyces, several cross-talking regulatory networks, constituted by pathway-specific regulators and pleiotropic shared regulators, have been uncovered.
Histone deacetylase (HDAC) inhibitors upregulate certain biosynthetic pathways
HDAC antagonizes the acetylation of histones and controls gene expression by leading to alterations in chromosome structure and gene expression in eukaryotes [Citation67,Citation68]. Conversely, HDAC inhibitors selectively target HDAC to alter this regulatory effect [Citation67]. More interestingly, HDAC inhibitors have been described to affect the metabolism of S. coelicolor.
The HDAC inhibitors sodium butyrate at a concentration of 25 mM and valproic acid at a concentration 0.5 mM inhibited ACT production and reduced RED production on R5 medium, which is a rich medium for the S. coelicolor A3 (2) strain, M145 [Citation69,Citation70]. However, on a minimal medium plate, 25 mM sodium butyrate strongly induces ACT production and slightly induces RED production, whereas 1 mM valproic acid strongly induces the production of ACT. Moreover, HDAC inhibitors such as suberoylanilide hydroxamic acid, trichostatin A, apicidin, phenyl butyrate, splitomicin, resveratrol, and quercetin inhibit the production of ACT and/or RED in S. coelicolor [Citation71]. Notably, the variation induced by sodium butyrate is similar to that caused by N-acetylglucosamine induction, which was operated by DasR transcription factor [Citation72]. Meanwhile, HDACs inhibitors suppressed ACT production on rich medium and promoted ACT overproduction on minimal plates, revealing the cross-talk with nutritional regulation. This suggests the existence of a synergistic effect and provides a novel point for investigations into the molecular mechanism(s) by which this HDAC inhibitor alters the regulation of antibiotic synthesis.
HDAC and its associated proteins are known as DNA-binding repressors or co-repressors [Citation73]. Overexpression of the sirtuin-like molecule SCO6464 inhibited ACT and RED production by S. coelicolor on R5 agar, and this effect was reversed by the addition of splitomicin [Citation71]. HDAC inhibitors released this repression by adjusting the dynamic chromatin-like structure of the nucleoid in S. coelicolor (). Up-regulation of certain biosynthetic pathways (including sugar, sesquiterpene, hopanoids, germicidin, and coelibactin) following treatment with HDAC inhibitors in S. coelicolor may occur through modification of the nucleoid structure with concomitant alteration of the expression of secondary metabolic gene clusters, among others [Citation71]. Predictably, this method can be used to screen for potential new antibiotics, enhance the activity of existing antibiotics, or overcome the inhibitory effects on antibiotic production.
Cholesterol oxidase enhances antibiotic production by acting as fungal sensors
Cholesterol oxidases are flavoproteins that catalyze the degradation of cholesterol or other sterols with a 3-β-hydroxyl group [Citation74]. To date, numerous Streptomyces gene clusters for the synthesis of small-sized polyenes have been revealed to contain a cholesterol oxidase-encoding gene, such as the pimaricin gene clusters (sgnE in S. gilvosporeus [Citation6], pimE in S. natalensis [Citation75], scnE in S. chattanoogensis [Citation76], and sclE in S. lydicus [Citation47]), tetramycin gene clusters (tetrO in S. hygrospinosus var. beijingensis [Citation77]), filipin gene clusters (pteG in S. avermitilis [Citation5] and filG in S. filipinensis [Citation78]), and rimocidin/CE-108 gene clusters (rimD in S. diastaticus [Citation79]).
In S. natalensis, pimE appears to have an obvious role in antifungal production, namely the effects on the synthesis and condensation of carbon skeleton and glycosyl [Citation80]. However, functional analyses confirmed that pimE is essential for the biosynthesis of pimaricin. This extracellular cholesterol oxidase was revealed to restore pimaricin production when added to cultures of knockout mutants or “resting cells” [Citation81]. Wang et al. also confirmed that an extra copy of sgnE, which confers higher cholesterol oxidase activity, enhances the production of pimaricin in S. gilvosporeus [Citation82].
Aparicio et al. [Citation80] proposed that cholesterol oxidase can act as a fungal sensor. Once ergosterol, a representative component of fungi, was detected, a response triggering the biosynthesis of antifungal antibiotics is activated, and natamycin production begins [Citation83]. Our recent research on S. gilvosporeus indicated that the regulatory mechanism of cholesterol oxidase may be directly related to ROS homeostasis in S. gilvosporeus. Hydrogen peroxide (H2O2) is a final enzymatic reaction product [Citation74]. Our research demonstrated that it can be produced in an enzymatic reaction using ergosterol and stigmasterol as substrates. The production and accumulation of H2O2 and the subsequent alteration of ROS homeostasis are proposed to be the signals that trigger the biosynthesis of natamycin, which has reducibility and antifungal activity, to prevent ROS imbalance and respond to invading fungi. Studies of the downstream regulatory proteins and targets of the natamycin synthesis gene cluster in this pathway are currently ongoing (unpublished data).
Phosphopantetheinyl transferase (PPTase) and its inhibitor 7ae activate new antibiotic production
PPTase is involved in the biosynthesis and post-translational modification of polyketide and non-ribosomal peptides [Citation84]. PPTase genes in the gene cluster for pactamycin [Citation85], A-74528 [Citation86], tirandamycin [Citation87], oviedomycin [Citation88], griseorhodin [Citation89], and fredericamycin production [Citation90] in different Streptomyces species have been identified. PPTase appears to be the most likely enzyme to add a 4′-phosphopantetheine group onto the apo form of the acyl carrier protein (ACP) to yield mature proteins. Recently, a highly efficient approach for activating cryptic biosynthetic pathways through the augmentation of PPTase was developed.
Upon PPTase overexpression, S. alboniger NRRL B-1832 produces the same three products as the wild-type strain and it further yields one known product (puromycin A), and two new derivatives (puromycins B and C) [Citation91]. Through PPTase overexpression, the production of halichomycin in S. ghanaensis, oviedomycin A (angucycline family), and oviedomycins B and C (moderate anti-cancer activity) in S. antibioticus NRRL 3238 is significantly activated [Citation92]. Similar activation of antibiotic production by PPTases has been illustrated in numerous Streptomyces species, such as natamycin in S. chattanoogensis L10 (increased by 40%) [Citation93]. Cultures of S. venezuelae ISP5230, which carry a disrupted PPTase family gene (jadM), produce only 2–5% of the wild-type titer of jadomycin B [Citation94].
Inhibitors of PPTase influence antibiotic synthesis through another mechanism. 7ae is synthesized through the effects of the anthranilate 4-H-oxazol-5-one pharmacophore, which can attenuate PPTase activity. Intriguingly, 7ae enhances ACT production when added to cultures of S. coelicolor. Foley et al. proposed a working hypothesis [Citation95] (). Two classes of PPTase, specifically AcpS-type and Sfp-type PPTases, are responsible for modifying carrier protein domains in all secondary metabolic pathways. Culturing microorganisms with 7ae chemically inactivates constitutive ACPs, which only act on the dissociated fatty acid synthase (FAS)-ACP, leaving FAS–ACP in the apo form. Chemical inactivation of AcpS transduces a nutrient deficiency signal, thereby triggering the up-regulation of secondary metabolic gene clusters that contain a Sfp-type PPTase. This process is resistant to the inhibitory effects of 7ae. This increases the flux of acetate units through the ACT biosynthetic pathway by decreasing the demands on a shared substrate pool and concomitant metabolite production [Citation95,Citation96]. Thus, unlike the opposing effects of HDAC and HDAC inhibitors on antibiotic synthesis, PPTase overexpression and the PPTase inhibitor 7ae both positively influence antibiotic production, providing better practicality.
Fatty acid synthesis enzyme inhibitors affect primary metabolic flux
Because of the shared precursors, fatty acids and secondary metabolism is linked. Some fatty acid synthesis components, such as acetyl-CoA and malonyl-CoA, are the main substrates of polyketide for antibiotic synthesis in Streptomyces. Thus, the metabolic flux of those precursors is a key point for both fatty acids and antibiotics [Citation97]. To increase antibiotic production, inhibition of fatty acid synthesis is a feasible method.
Figure 3. pH shock regulation in Streptomyces. Temperature shifts cause changes in antibiotic metabolism by influencing the TCA cycle, energy metabolism, and TCS. ACT: actinorhodin; CoQ: coenzyme Q; Cyt: cytochrome; Fe-S: iron–sulfur protein; FP: flavin proteases; P: phosphate group; TCA: tricarboxylic acid cycle; ε-PL: ε-poly-l-lysine; TCS: two-component regulatory system.
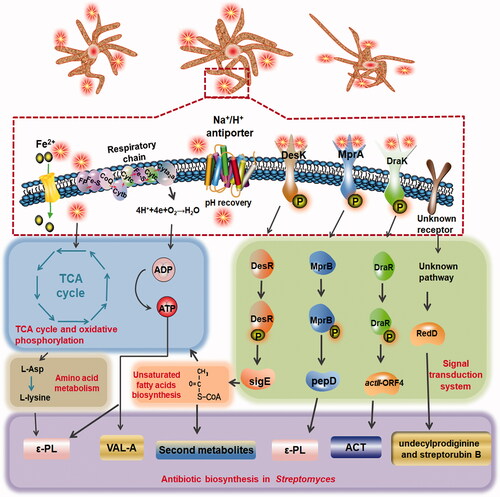
The antibiotic triclosan, an antibiotic remodeling compound (ARC), shares a similar furan structure with ACT. Craney et al. found that triclosan can enhance the ACT synthesis in S. coelicolor [Citation98]. Among all of the remodeling compounds, ARC2 is the most active. The ARC2 series of molecules both enhanced the yields of ACT in S. coelicolor and induced the production of as-yet unidentified compounds in S. peuceticus [Citation98,Citation99]. A derivative of ARC2, called C1-ARC, was also used to increase the oxohygrolidin yield in S. ghanaensis cultures [Citation100].
Craney et al. [Citation98] proposed that the mechanism of ARC2 involves its activity as an inhibitor of fatty acid synthesis and that this function is conserved across various actinomycetes. ARC2 molecules perform their functions via inhibition of the enoyl-ACP reductase FabI, which catalyzes the final rate-limiting step in fatty acid biosynthesis. Partial inhibition of fatty acid synthesis can allow the preferential flow of acetyl-CoA and malonyl-CoA toward antibiotic production [Citation101] ().
For most antibiotics, short chains of carbons and their derivatives, such as acetyl-CoA and malonyl-CoA, are the shared precursors between polyketide and fatty acid synthesis. The production of antibiotics in Streptomyces requires precursors from fatty acid synthesis, and this process is also affected by the primary metabolic flux of fatty acids.
Environmental stress elicitors trigger antibiotic synthetic gene expression
Environmental stress, such as nutrient and oxygen deprivation, is a crucial approach for promoting secondary metabolite production in Streptomyces [Citation102]. In addition to those conventional approaches, environmental factors such as heat shock and pH shock can be used to effectively trigger the expression of secondary metabolic genes, especially the antibiotic synthesis gene clusters [Citation31].
Temperature shifts cause changes in antibiotic metabolism
Fermentation at optimum temperature is an important factor during antibiotic metabolism. Streptomyces are mesophiles that grow optimally at 25–35 °C, but heat shock can also cause changes in their antibiotic metabolism.
A temperature increase from 35 to 37 °C enhances Val-A production by S. hygroscopicus by 5-fold. Particularly, high fermentation temperatures (e.g. 42 °C) further enhance Val-A production [Citation103,Citation104], similar to that observed for jadomycin B production in S. venezuelae and methylenomycin production in S. coelicolor A3(2) [Citation105,Citation106]. Saito et al. revealed that almost half of 57 thermotolerant actinomycetes can produce “heat shock metabolites (HSMs)” (e.g. murecholamide, angucycline-related compounds) [Citation107]. Similarly, temperature decreases also have a dramatic effect on product yield. For example, recombinant human interleukin-3 production by an S. lividans strain is increased at least 2-fold when the culture temperature is reduced to 19 °C [Citation108] and ).
Table 1. Influence of rare earth elements (REEs) on antibiotic production.
Table 2. Influence of nanoparticles on antibiotic production.
All organisms respond to a sudden increase in temperature by inducing the synthesis of heat shock proteins (HSPs), which alter the regulatory effects of HrcA, RheA, and HspR on metabolism [Citation109]. Meanwhile, Bucca et al. [Citation110] monitored transcriptional and translational changes in S. coelicolor following heat shock, and 74 genes were more efficiently translated at 42 °C, including the OsdR/OsdS two-component system, which has roles in morphological development and antibiotic production in Streptomyces [Citation110]. It is worth noting that heat shock negates the repression of the pathway-specific transcription factor JadR2 and alleviates inhibition of the biosynthesis of jadomycin B in S. venezuelae [Citation111]. However, in S. hygroscopicus, temperature-mediated regulation is attributable to the enhanced transcription levels of the stress response sigma factors, sigma B and sigma H, as well as the global regulators PhoRP and ROS [Citation112].
For temperature upshift and temperature downshift, Streptomyces pleiotropic regulators such as ROS, HSPs, and the two-component system are the effectors that respond to environmental changes or stress. These pleiotropic regulators always regulate both primary and secondary metabolism, including antibiotic synthesis. Because of the lack of extraneous materials and the ability to perform temperature shifts during the fermentation process, this strategy can be considered the simplest method for enhancing antibiotic production in Streptomyces.
Role of pH shock in signal transduction and antibiotic synthesis
Changes in culture pH can play an important role in signal transduction and secondary metabolism or antibiotic synthesis. In S. coelicolor, undecylprodiginine synthesis was increased by 1.8-fold (37.22 mg/g) and streptorubin B synthesis was enhanced by 2.5-fold (18.61 mg/g) following acidic pH shock (from pH 7.2 to 4.0) [Citation113]. Moreover, acidic pH shock also enhanced methylenomycin [Citation114] and ACT production in S. coelicolor cultures [Citation115] and ε-poly-l-lysine (ε-PL) production in S. albulus [Citation116]. A 27.43% increase in Val-A production was achieved through alkaline shock (pH 6.4–8.0) in S. hygroscopicus 5008 [117].
The mechanism of modulation induced by pH shock is complex and varies among Streptomyces species. In S. coelicolor, pathway-specific regulator genes, putative Na+/H+ antiporter genes (e.g. red [Citation113], actII-ORF4 [Citation118]), and two-component system genes (e.g. DraR/DraK) have been considered responsible for pH shock regulation. Among the many potential factors governing the response to pH variation, the putative Na+/H+ antiporter (sha) genes promote recovery after pH variation in S. coelicolor, and they are also associated with antibiotic synthesis [Citation119]. In S. albulus, pH shock influenced large-scale proteins involving in regulatory systems (e.g. mprA/mprB and pepD signal transduction system), fatty acid metabolism (changes the ratio of unsaturated to saturated fatty acids through the DesK/DesR pathway, TCA cycle and amino acid metabolism, which provides ATP and precursors for ε-PL), respiration (cytochrome c oxidase, ferredoxin reductase, and iron–sulfur protein genes), and ATP-binding cassette (ABC) transporter during fermentation [Citation120]. The transcription of several genes in S. hygroscopicus related to amino acid metabolism, carbon metabolism, and the electron respiratory chain is altered by pH shock, and these changes are accompanied by a substantial increase in respiratory activity and the glutamate concentration [Citation117].
Thus, antibiotic synthesis is promoted by a series of complex mechanisms in response to pH stress signals (). However, the mechanisms by which this environmental signal triggers secondary metabolism are poorly understood.
Appropriate dissolved oxygen is required to activate the antibiotic synthesis switch
As Streptomyces is an aerobic bacterium, its growth is influenced by the dissolved oxygen concentration (DOC). A linear relation between virginiamycin production by S. virginiae and the dissolved oxygen tension from 10% to 80% was found by Shioya et al. [Citation121]. Similarly, the chlortetracycline yield in S. aureofaciens, tylosin and macrosin yields in S. fradiae, avermectin yield in S. avermitilis, and cephamycin C yield in S. clavuligerus [Citation122] were changed by the DOC. However, Kaiser [Citation123] found that manumycin production by S. parvulus (Tü 64) decreased when the DOC exceeded the optimal concentration (equivalent to 315 mbar in a stirred tank fermentor), but at higher O2 concentrations, the metabolites 64p-A, 64p-B, and 64p-C are formed [Citation123]. In S. olindensis So20, 100% air saturation induced a 2-fold increase of ratamycin production during the growth phase. Conversely, a large oxygen supply during the production phase did not contribute to an improvement of retamycin production[Citation124].
Through research on the effects of the DOC of antibiotic production, the metabolic switch from primary to secondary metabolism was somewhat revealed. Mehmood did not detect pristinamycin production when the oxygen uptake rate was limited by free-surface oxygen transfer in S. pristinaespiralis pr11, and the study revealed that non-limited oxygen transfer and nitrogen and/or phosphate are both needed to induce pristinamycin production [Citation122]. Evidence of increased production of a purple pigment under the hypoxic conditions by the Streptomyces strain CNQ-525 was observed by Gallagher [Citation125]. Gamboa-Suasnavart found that a low oxygen transfer rate can activate the metabolic switch and increase RED synthesis in S. lividans [Citation126].
According to previous studies, metabolism, especially the antibiotic synthesis switch, is usually influenced by limitations of phosphate and nitrogen availability in Streptomyces species [Citation127]. However, the aforementioned results revealed that the switch from primary to secondary metabolism involves a complex regulatory process. Nutrient limitations and an appropriate DOC for the appropriate growth stage are required to activate the antibiotic synthesis switch. Although further investigations are needed, this strategy provides another potential approach to enhance or activate antibiotic production.
Novel microorganisms or microbial metabolic elicitors enhance or induce the production of cryptic metabolites in Streptomyces
Streptomyces spreads in a variety of environments, including soil, marine, or botanical environments, and it cohabits with other organisms. Microbial interactions occur incessantly in the cohabitation environments [Citation128]. The interspecific stimulation of morphogenesis and secondary metabolism occurs among various Streptomyces species and related organisms [Citation129]. Contact with cells or cell fragments from fungi, bacteria, and even pathogenic microorganisms can act as an elicitor to enhance the synthesis of natural metabolites or induce their cryptic production in Streptomyces.
Fungal elicitors enhance gene expression and regulate enzyme activity in antibiotic synthesis
Fungal elicitors comprise a group of chemicals that can stimulate secondary metabolite production in plants [Citation130] and microbial cells [Citation131]. Among the fungal elicitors, the fungal cell wall and the filtrate of the fermentation broth significantly influences antibiotic synthesis by Streptomyces. In S. natalensis HW-2, the cell walls of Aspergillus oryzae AS 3.2068 and Penicillium chrysogenum AS 3.5163, as well as the filtrate of their fermentation broths, can affect natamycin biosynthesis. The addition of elicitors obtained from P. chrysogenum AS 3.5163 increased the natamycin yield by nearly 2-fold. Interestingly, the elicitors obtained from P. chrysogenum AS 3.5163 inhibited the growth of S. natalensis HW-2 and accelerated glucose utilization [Citation132]. The fermentation broth of A. niger and Saccharomyces cerevisiae exert an analogous effect on natamycin production in S. nautilus [Citation133].
Natamycin is a typical polyene antibiotic that exerts antifungal activity through interactions with membrane sterols such as ergosterol, which is the major sterol found in fungal membranes [Citation134]. Therefore, it is conceivable that the addition of fungal elicitors induces the detection of ergosterol by Streptomyces and triggers antifungal production [Citation80]. Wang et al. [Citation135] investigated the mechanism by which fungal elicitors enhance natamycin production and identified increases in ROS and Ca2+ levels following exposure to fungal elicitors, as well as the alteration of the activities of enzymes involved in primary metabolism, such as enhanced pyruvate carboxylase and phosphoenolpyruvate carboxylase activity and decreased citrate synthase activity [Citation135], as potential mediators of this process. From these limited data and assumptions, we found a relationship among fungal elicitors, ROS, Ca2+, primary metabolism, and antifungal antibiotic synthesis in Streptomyces. This indicated that the fungal elicitor may be able to promote extracellular Ca2+ influx and alter primary metabolism to increase precursor production. Considering that antibiotic production is often associated with a morphophysiological differentiation program, which could be affected by Ca2+ [Citation136], a calcium-binding protein may be another regulator in natamycin-producing strains. Natamycin is a reduced polyene macrolide that can be oxidized by H2O2, which is a key component of ROS. Thus, the increased ROS production induced by fungal elicitors may be another regulation pathway or a “second messenger” of fungal elicitors in Streptomyces, similar to cyclic di-GMP in bacteria [Citation137] or H2O2 in eukaryotes [Citation138]. Although the details of this mechanism remain unknown, fungal elicitors provide a route for improving the yield of natamycin and other polyene antibiotics.
Complex communities induce cryptic antibiotic production
Streptomyces species, which are traditionally cultured in monoculture, are mainly found in soil, in which microorganisms inhabit communities with complex interactions. The absence of biotic and abiotic interactions limits the chemical diversity and leads to “poorer” chemical profiles [Citation139]. To simulate the natural environment, co-culture has emerged as an effective method for inducing the production of antibiotics in Streptomyces.
Ueda et al. [Citation129] reported that cross-feeding experiments with Streptomyces species resulted in enhanced antimicrobial activity. Charusanti [Citation140] also found that holomycin (an anti-staphylococcal agent) is produced in a co-culture of S. clavuligerus and methicillin-resistant Staphylococcus aureus N315. Imai et al. [Citation141] confirmed that the co-culture of S. coelicolor with the erythromycin, producer Saccharopolyspora erythraea or streptomycin producer S. griseus, enhanced the production of ACT by S. coelicolor. Moreover, the mycolic acid-containing bacteria Tsukamurella pulmonis, Rhodococcus erythropolis, and Corynebacterium glutamicum can improve or inhibit antibiotic biosynthesis in Streptomyces strains [Citation142] (). For example, ACT and RED production is induced by S. lividans co-cultured with T. pulmonis and C. glutamicum. S. endus S522 produced the novel antibiotics, alchivemycins A and B. upon co-culture with T. pulmonis TP-B0596. The production of several cryptic secondary metabolites, including arcyriaflavin E (cytotoxic to human cells) by S. cinnamoneus NBRC 13823 [Citation143], chojalactone (anti-cancer activity) by Streptomyces sp. CJ-5, niizalactam by Streptomyces sp. NZ-6 [Citation144], and 5-alkyl-1,2,3,4-tetrahydroquinolines (antifungal activity) by S. nigrescens HEK616 [Citation145,Citation146], can be activated via co-culture of these antibiotic-producing strains with T. pulmonis.
Table 3. Influence of co-culture on antibiotic production.
However, when mycolic acid extracted from T. pulmonis was added to the culture broth of S. lividans, ACT or RED production was not induced. However, the physical interaction of T. pulmonis with S. lividans can induce the production of these pigments [Citation142]. Thus, unlike fungal elicitors, this induction requires physical contact or an interaction with living cells. For the methicillin-resistant Staphylococcus aureus strain N315, erythromycin producer Saccharopolyspora erythraea, and streptomycin producer S. griseus, the activation is related to antibiotic resistance (details below). Meanwhile, T. pulmonis, R. erythropolis, and C. glutamicum belong to Actinomycetales as does Streptomyces. Similar metabolites, such as diffusible autoregulatory elicitors (e.g. γ-butanolide type autoregulators [Citation144,Citation147,Citation148]), in co-cultures have been proposed to play intraspecific and interspecific roles in different species, and their effects may be mutually reinforced. The latest research by Westhoff and van Wezel [Citation149] revealed another phenomenon deserving consideration. By co-culturing 24 antibiotic-producing strains of Streptomyces, they found that closely related Streptomyces strains more frequently inhibit each other’s growth. However, closely related strains or strains sharing a secondary metabolite biosynthetic gene cluster were more likely to induce antibiotic production than were competitors. In addition, resource limitations induce production declines during competition [Citation149]. These findings highlight that interactions between social and resource environments strongly regulate antibiotic production. Thus, further research of the effects of cell-to-cell physical contact, chemical substances such as A-factor-like autoregulators or goadsporin, or resource limitations is needed (). The induction of antibiotic production through co-cultures is likely to attract increasing research interest.
ROS influence antibiotic synthesis as intercellular and intracellular signals
ROS are formed upon the incomplete reduction of oxygen, superoxide dismutase, and proteins containing Fe-S clusters, flavin, or quinone [Citation150]. ROS include the superoxide anion, H2O2, and the hydroxyl radical. ROS mediate the toxicity of oxygen because of their marked chemical reactivity [Citation150]. In recent years, ROS have been recognized as important and common regulators of antibiotic metabolism in Streptomyces. In addition to alterations of ROS levels induced by DMSO, ethanol, nanoparticles, fungal elicitors, cholesterol oxidase, and temperature shifts, changes of ROS levels resulting from the variation of redox-active metabolites in Streptomyces have been found to influence antibiotic metabolism.
In S. peucetius, sod1 and sod2 overexpression decreases doxorubicin production [Citation151]. Hahn et al. investigated the roles of ROS in secondary metabolite production [Citation152]. The SodF-defective mutant of S. natalensis (CAM.02) exhibits lower intracellular H2O2 levels during the growth phase, and during the late exponential phase, natamycin production is reduced by 1.3-fold [Citation153]. Similar effects were confirmed in other Streptomyces strains [Citation112,Citation154,Citation155]. With the addition of the ROS inhibitor diphenyleneiodonium, intracellular ROS accumulation, and Val-A production are inhibited [Citation112]. The H2O2-resistant mutant S. coelicolor A3(2) HR40 produced lower levels of the blue antibiotic ACT than the wild-type strain. The ΔcatR mutant, which exhibited CatA overproduction (CatR is a redox-sensitive regulator that controls catalase), also displayed reduced ACT production [Citation152].
The intracellular H2O2 level is considered important for eliciting natamycin biosynthesis, and the regulatory mechanism has been investigated according to the cross-talk between ROS homeostasis and antibiotic synthesis during the late exponential phase [Citation153]. Global regulators, such as sigma factors, the two-component regulatory system, SoxR, OxyR, OhrR, and CatR-CatA appear to be the main factors that respond to ROS in Streptomyces [Citation150,Citation156]. In S. clavuligerus, sigma B and sigma H, as well as the global regulator PhoRP, might influence the biosynthesis of clavulanic acid and mediate the redox cycling signal pathway [Citation154,Citation155]. The [2Fe-2S]-containing transcription factor SoxR binds to the promoter regions of oxoreductase genes, ABC transporter genes and hypothetical protein genes, thereby activating their expression and promoting ACT production in S. coelicolor [Citation157,Citation158]. Recently, Cheng et al. [Citation159] demonstrated that OxyR positively regulates the transcription of IdeR, a DtxR-family global regulator that is essential for the oxidative stress response, which affects the antibiotic synthesis in S. avermitilis.
However, in various Streptomyces strains, ROS (H2O2 in particular) can negatively influence antibiotic production. Zeng et al. noted that high levels of ROS lead to a decrease in the ε-PL formation rate in Streptomyces sp. M-Z18 [Citation160]. Yan et al. recorded a sharp increase of ROS levels in Streptomyces sp. AF3-44 within 24 h, and the addition of the antioxidant glutathione, before this increase stimulated ε-PL synthesis during shake flask fermentation with a lower concentration of l-lysine [Citation161]. The biosynthesis of ε-PL is limited by l-lysine, which participates in amino acid metabolism and differs from these precursors, which are derived mainly from the TCA cycle.
Thus, ROS may act as intercommunal and intracellular signals to mediate secondary metabolism in Streptomyces. From the available data, targets of oxidative stress-sensing transcriptional regulators do not include the genes of antibiotic synthesis gene clusters. Thus, it appears that the observed changes on secondary metabolism are indirect effects arising from the changes of primary metabolism. Current knowledge regarding the function and mechanism of ROS in antibiotic synthesis is summarized in . Importantly, Al2O3 nanoparticles and ROS also reduced the initial time to RED and ACT synthesis in addition to increasing their production [Citation64]. Together with the analysis of ROS homeostasis and the biosynthesis of natamycin (ROS section), ROS induction strategies have great potential as new approaches for enhancing antibiotic production if ROS levels are suitably controlled during the aerobic fermentation process.
Figure 5. ROS influence secondary metabolism as intercellular and intracellular signals. ROS may act as intercommunal and intracellular signals to mediate secondary metabolism in Streptomyces. ACT: actinorhodin; CA: clavulanic acid; DXR: doxorubicin; God: goadsporin; ROS: reactive oxygen species; Val-A: validamycin A; ε-PL: ε-poly-l-lysine.
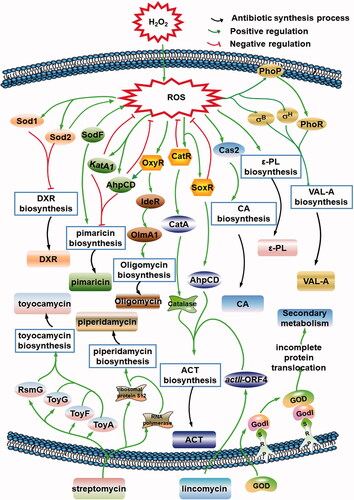
Antibiotics can strongly induce gene expression through their roles as signaling molecules
Antibiotics exert bactericidal or bacteriostatic effects [Citation162]. However, recent reports have indicated that antibiotics act as signaling molecules that can strongly induce gene expression and influence antibiotic outcomes in a specific strain or biosynthetic gene cluster in Streptomyces [Citation25,Citation162–164].
In S. lividans 1326, the production of several antibacterial compounds is induced by lincomycin at one-twelfth or one-third of the minimum inhibitory concentration, compared with lincomycin-free medium [Citation141]. Analogously, S. coelicolor A3(2) overproduced ACT because of increases in the expression of the regulatory gene actII-ORF4 in response to lincomycin at one-tenth of the minimum inhibitory concentration via high ATP levels and enhancement of the synthesis of S-adenosyl-l-methionine and cyclic AMP, ultimately leading to ACT overproduction [Citation141]. Hosaka et al. [Citation165] designed mutants resistant to the streptomycin, gentamicin, and rifampicin, observing both increased antibiotic production in seven Streptomyces strains and increased production of the novel antibiotic piperidamycin in S. mauvecolor 631689. Toyocamycin production was 24-fold higher in streptomycin- and paromomycin-resistant S. diastatochromogenes SD3145 strain than in the wild type. The dramatic overproduction of toyocamycin was attributed to enhanced expression of the rsmG (16S rRNA methyltransferase gene [Citation166]) and toyA (a LuxR-family transcriptional regulator gene for toyocamycin biosynthesis) genes, as well as increased expression of the toyocamycin biosynthesis genes, toyF and toyG [Citation38]. At the transcriptional level, rifampin resistance mutations can activate the cryptic or silent secondary metabolite biosynthetic gene clusters of Streptomyces, Saccharopolyspora and Amycolatopsis by up to 70-fold [Citation167]. A 4.5-fold increase of toyocamycin production was observed in a rifampicin-resistant S. diastatochromogenes 1628 strain [Citation168]. Strains resistant to antibiotics generated ε-PL at a concentration of 4.41 g/L, which was 2.75-fold higher than production of the parental strain [Citation169].
Antibiotics that induce secondary metabolite production in streptomycetes exhibit characteristic ribosome targeting. Streptomycin, lincomycin, and rifampicin target ribosomal protein S12 or RNA polymerase. Thus, ribosome or RNA polymerase mutations, which result in antibiotic resistance, result in increased antibiotic production but also the generation of novel antibiotics in Streptomyces and Bacillus subtilis [Citation170]. This approach is also called “ribosome engineering,” and it has been widely applied to promote antibiotic overproduction and novel antibiotic discovery. For example, the rsmG mutant of S. diastatochromogenes exhibited increased toyocamycin and the activation of silent genes involved in the biosynthesis of antibiotics [Citation171]. Yim proposed that antibiotics secreted by microbes have roles as cell-signaling molecules in the environment and regulate gene expression in microbial populations [Citation164]. This theory has been supported by Bachmann, who stated that environmental chemicals and biological stimuli can activate secondary metabolism [Citation172]. Thus the associated mechanism may be more complex than anticipated.
Goadsporin acts as a signaling molecule to modulate the expression of genes involved in antibiotic production
Goadsporin, containing an N-terminal acetyl moiety, six azoles, and two dehydroalanines in the peptidic main chain, is a secondary metabolite synthesized in the ribosomes in Streptomyces sp. TP-A0584 [Citation173,Citation174]. Goadsporin at low concentrations can promote secondary metabolism and morphogenesis, whereas high concentrations can inhibit the growth of actinomycetes. For example, 1 mM goadsporin stimulates the production of prodiginine antibiotics in S. lividans, whereas goadsporin at concentrations of >1 mM inhibits cell growth. Low concentrations of goadsporin have also been reported to promote pigment production and morphogenesis in 36 Streptomyces species [Citation175,Citation176].
The mechanism of antibiotic production induced by goadsporin is proposed to be associated with protein translocation and GodI. In S. avermitilis and S. coelicolor A3(2), GodI was found to be highly homologous to Ffh, which is essential for production of the signal recognition particle in Escherichia. coli. In E. coli, exposure to goadsporin results in the malfunction of Ffh or the abnormal translocation of membrane proteins [Citation177]. Conversely, in Streptomyces species, GodI is resistant to goadsporin because as goadsporin cannot bind to the protein, which results in the induction of secondary metabolism in these species () [Citation174,Citation176,Citation177]. Notably, the relationships between protein translocation and secondary metabolism have been rarely researched in actinomycetes, and such research may lead to the discovery of novel regulatory systems for antibiotic production.
Combined new methods based on elicitors are effective for inducing cryptic metabolite production
For natural product fermentation, microorganisms are mainly cultivated under standard conditions, and most gene clusters involved in secondary metabolism are silent or poorly expressed in Streptomyces. During stimulation of the silent gene clusters of Streptomyces, the limited capacity and inefficiency of single changes prompted the development of combined methods with the high-throughput screening approach (“HiTES”) and “one strain many compounds (OSMAC)” as the representative strategies.
Bioactivity-HiTES is a high-throughput elicitor screening for harvesting cryptic metabolites
Streptomyces, with its multitudinous biosynthetic gene clusters, is considered a massive reservoir of potentially novel small molecules. However, many clusters are silent or cryptic, necessitating their activation. To date, a major limitation to this aim has been the poor efficiency of the methods and the requirements of bioactivity-HiTES genetic procedures. Seyedsayamdost [Citation178] devised a chemogenetic high-throughput-HiTES to study the activation of silent clusters.
eGFP, as a readout for secondary metabolite synthesis, was inserted both at a neutral site and inside the biosynthetic cluster of interest in a high-throughput transcriptional assay format devised by Seyedsayamdost. Elicitors such as etoposide, cyclosporine A, dihydroergocristine, piceatannol, bromolaudanosine, and ivermectin were used, and a silent gene cluster in S. albus J1074 was successfully activated using this approach [Citation178]. A similar result also was observed in Burkholderia thailandensis using lacZ as the genetic reporter [Citation179]. Then, HiTES was upgraded to bioactivity-HiTES, which links the bioactivity of cryptic metabolites to their induction. Three actinomycete strains were revealed to produce cryptic antibiotics, including S. hiroshimensis, in which prodigiosin was elicited by the beta-blocker atenolol [Citation180]. HiTES combined with imaging mass spectrometry was developed and applied to S. canus NRRL B3980, leading to the discovery of canucins A and B [Citation181]. This rapid detection technology enables the interrogation of the global secondary metabolome of any culturable bacterium in response to 500–1000 conditions.
At present, the mechanism by which silent clusters are activated is unclear, but HiTES combining chemical elicitors and genetic reporters has already displayed the advantage of high efficiency, especially compared with induction by chemical elicitors or genetic reporters alone in Streptomyces spp.
OSMAC approach induces silent biogenetic clusters during conventional fermentation
The OSMAC strategy stimulates secondary metabolite production through the systematic variation of growth parameters, and it has been used in species such as Myxotrichum sp. [Citation182], Fusarium tricinctum [Citation183,Citation184], and marine microorganisms [Citation185,Citation186]. Several studies confirmed that OSMAC is a crucial method for the discovery of novel natural products from Streptomyces. Zazopoulos used selective growth conditions to induce the expression of macromomycin PKSs after the high-throughput genome scanning of gene clusters involved in natural product biosynthesis in the S. macromyceticus strains M480-M1 and NRRL B-5335 [Citation187]. Romano [Citation186] and Pan [Citation188] summarized the usage of OSMAC approaches, and we analyzed new findings. Inthomycin B, a bioactive compound isolated from the marine-derived strain Streptomyces sp. YB104, was found using a cultivation-dependent procedure involving: ISP2, ISP4, ACM, TSB, CQ-1, SAO-23, and FP-1 media with a yield of approximately 25 mg/L [Citation189]. Nocardamin glucuronide and nocardamin were obtained by applying OSMAC to the ascamycin-producing strain Streptomyces sp. 80H647 [Citation190].
Along with the development of interdisciplinary approaches, the incorporation of OSMAC into genomics[Citation191], proteomics [Citation28], metabonomics, and bioinformatics has been rapidly utilized. Because of the interdisciplinary characteristics of this strategy, we termed this approach “second-generation OSMAC,” which is distinct from the initial strategy in that only fermented parameters were changed. Based on the OSMAC approach (ISP2, BFM1-11, BFM13, and BFM14 media) and UPLC-HRMS-based metabolomics screening, terrosamycins A and B, two polycyclic polyether natural products with antibiotic activity against Gram-positive pathogens and breast cancer cell lines, were purified from Streptomyces sp. RKND004 [192]. Surugamides and two putative new analogs were detected in the marine strain Streptomyces sp. BRB081 by OSMAC (grown in six different media), HPLC-MS/MS, and Global Natural Product Social Molecular Networking (GNPS) [Citation193] analysis. Meanwhile, genome mining operated by Baltz [Citation13] and Bachmann [Citation194] was shown to be an efficient method for discovery of natural products. When genome mining coupled with OSMAC-based cultivation was used in Streptomyces sp. SM17, it resulted in >13-fold higher antibiotic levels in YD medium and 3-fold higher levels in SYP-NaCl medium than achieved using the closely related strain S. albidoflavus J1074 [Citation195]. OSMAC used in combination with HR-MS, NMR, SMART 2.0 technology, and GNPS analysis also facilitated the discovery of the new antibiotic legoncarbamate, which is a carbamate-containing heterobicyclic alkaloid with a minimum inhibitory concentration of 3.1 μg/mL against E. coli ATCC 25922 [Citation196]. Machushynets activated the production of quinazolinones A and B via OSMAC in glycerol with GNPS in Streptomyces sp. MBT27, which resulted in a quinazolinone-related network [Citation197].
Thus, OSMAC can lead to the stimulation of secondary metabolites and the discovery of novel metabolites with potent bioactivities by changing culture conditions and microbial physiology. Compared with the effects of genetic engineering methods, OSMAC is considered a simple approach for activating antibiotic production. Second-generation OSMAC, with interdisciplinary characteristics and high efficiency, offers greater potential for productive deployment in Streptomyces. It must be noted that OSMAC is more suitable for novel antibiotic discovery in novel microorganisms such as marine microorganisms and extremophiles.
Concluding remarks and future outlook
Antibiotics produced by various organisms have received special attention in both industrial and medical fields. Among antibiotic-producing organisms, Streptomyces has a key role in the production of antibiotics through secondary metabolism, but silent or poor expression of gene clusters is a significant impediment to antibiotic discovery and production. To enhance the production of antibiotics or activate silent antibiotic production in Streptomyces, several strategies have been employed in recent decades, and elicitation is an effective method. In this review, we described elicitors that have not received substantial attention despite obviously enhancing antibiotic production, such as certain chemicals, microbial metabolic elicitors, microbial interactions, enzymes, enzyme inhibitors, and environmental factors. In addition, we summarized the novel methods based on combined approaches. Those elicitors, especially when used together with genetic engineering (HiTES), as well as the use of OSMAC with genomics, metabonomics, and bioinformatics, have already displayed the ability to enhance or activate antibiotic production.
The rate of discovery of new antibiotics is slowing. Discovery of new antibiotics using elicitor-based activation and the possibility of rediscovery of known compounds are promising approaches. Traditional culturing methods for high production of antibiotics from microbes often require proportionate expression of many metabolic pathways and concordant metabolic flux between primary metabolites and secondary metabolites. Often, many cryptic gene clusters do not participate in metabolism except under specific conditions. The use of elicitors such as nutrients, trace elements, physical parameters, and chemical elicitors, as well as the co-cultivation of microbes and factors affecting epigenetic control, can be used to investigate antibiotic production [Citation186,Citation192,Citation195,Citation198,Citation199]. However, during the stimulation of silent gene clusters of Streptomyces, the limited capacity and inefficiency of single-condition changes prompted the development of interdisciplinary methods, such as HiTES and OSMAC. These interdisciplinary methods represent simple, rapid and effective approaches for enhancing chemodiversity and obtaining new antibiotics by activating silent gene clusters, highlighting their utility for overcoming challenges to antibiotic discovery and production. Reassuringly, van Wezel et al. provided a promising strategy for activating the production of cryptic metabolites or increasing the yield of known compounds, by identifying the cis elements and their corresponding transcription factors through bioinformatic approaches [Citation200]. With the help of bioinformatics, this strategy will be another high-efficient strategy for antibiotic production and discovery. Furthermore, to address future challenges in the discovery of new antibiotics using elicitors, the discovery of new Streptomyces strains combined with use of elicitors is a potential strategy. An important trend has emerged that marine microorganisms, especially marine Streptomyces, are being increasingly used as an antibiotic resource pool with the increased focus on marine science [Citation186]. Marine environments have geochemical features, and they cover >70% of the earth’s surface. In addition, these environments contain millions of phylogenetically divergent microorganisms with chemical biodiversity [Citation201]. However, the production of different secondary metabolites, production of antibiotics, and associated complex regulatory networks remain to be elucidated. These areas must be investigated to enhance the development of new strategies for improving secondary metabolite yield or activating novel antibiotic production.
Acknowledgments
The authors thank Scott Wysong and Joe Barber Jr, PhD, from Liwen Bianji (Edanz) (www.liwenbianji.cn/), for editing the English text of a draft of this manuscript.
Disclosure statement
No potential conflict of interest was reported by the authors.
Additional information
Funding
References
- Ruiz B, Chavez A, Forero A, et al. Production of microbial secondary metabolites: regulation by the carbon source. Crit Rev Microbiol. 2010;36(2):146–167.
- Ochi K. Insights into microbial cryptic gene activation and strain improvement: principle, application and technical aspects. J Antibiot (Tokyo). 2017;70(1):25–40.
- Weber T, Welzel K, Pelzer S, et al. Exploiting the genetic potential of polyketide producing Sreptomycetes. J Biotechnol. 2003;106(2–3):221–232.
- Bentley SD, Chater KF, Cerdeño-Tárraga A-M, et al. Complete genome sequence of the model actinomycete Streptomyces coelicolor A3(2). Nature. 2002;417(6885):141–147.
- Ikeda H, Ishikawa J, Hanamoto A, et al. Complete genome sequence and comparative analysis of the industrial microorganism Streptomyces avermitilis. Nat Biotechnol. 2003;21(5):526–531.
- Zong G, Zhong C, Fu J, et al. Complete genome sequence of the natamycin high-producing strain Streptomyces gilvosporeus F607. Genome Announc. 2018;6(1):e01402–e01417.
- Ohnishi Y, Ishikawa J, Hara H, et al. Genome sequence of the streptomycin-producing microorganism Streptomyces griseus IFO 13350. J Bacteriol. 2008;190(11):4050–4060.
- Genilloud O. The re-emerging role of microbial natural products in antibiotic discovery. Antonie Van Leeuwenhoek. 2014;106(1):173–188.
- Ochi K, Hosaka T. New strategies for drug discovery: activation of silent or weakly expressed microbial gene clusters. Appl Microbiol Biotechnol. 2013;97(1):87–98.
- Zhu H, Sandiford SK, van Wezel GP. Triggers and cues that activate antibiotic production by actinomycetes. J Ind Microbiol Biotechnol. 2014;41(2):371–386.
- Liu G, Chater KF, Chandra G, et al. Molecular regulation of antibiotic biosynthesis in Streptomyces. Microbiol Mol Biol Rev. 2013;77(1):112–143.
- Bachmann BO. Applied evolutionary theories for engineering of secondary metabolic pathways. Curr Opin Chem Biol. 2016;35:133–141.
- Baltz RH. Gifted microbes for genome mining and natural product discovery. J Ind Microbiol Biotechnol. 2017;44(4–5):573–588.
- Tang Z, Xiao C, Zhuang Y, et al. Improved oxytetracycline production in Streptomyces rimosus M4018 by metabolic engineering of the G6PDH gene in the pentose phosphate pathway. Enzyme Microb Technol. 2011;49(1):17–24.
- Schäper S, Steinchen W, Krol E, et al. AraC-like transcriptional activator CuxR binds c-di-GMP by a PilZ-like mechanism to regulate extracellular polysaccharide production. Proc Natl Acad Sci U S A. 2017;114(24):E4822–E4831.
- Covington BC, Xu F, Seyedsayamdost MR. A natural product chemist's guide to unlocking silent biosynthetic gene clusters. Annu Rev Biochem. 2021;90:763–788.
- Martín JF, Liras P. The balance metabolism safety net: integration of stress signals by interacting transcriptional factors in Streptomyces and related actinobacteria. Front Microbiol. 2019;10(3120):3120.
- Katz L, Baltz RH. Natural product discovery: past, present, and future. J Ind Microbiol Biotechnol. 2016;43(2–3):155–176.
- Craney A, Ahmed S, Nodwell J. Towards a new science of secondary metabolism. J Antibiot (Tokyo). 2013;66(7):387–400.
- Farrell K, Jahan MA, Kovinich N. Distinct mechanisms of biotic and chemical elicitors enable additive elicitation of the anticancer phytoalexin glyceollin I. Molecules. 2017;22(8):1261.
- Kummritz S, Louis M, Haas C, et al. Fungal elicitors combined with a sucrose feed significantly enhance triterpene production of a Salvia fruticosa cell suspension. Appl Microbiol Biotechnol. 2016;100(16):7071–7082.
- Rodríguez AR, Carmona NM, Villafán BR, et al. Interplay between carbon, nitrogen and phosphate utilization in the control of secondary metabolite production in Streptomyces. Antonie Van Leeuwenhoek. 2018;111(5):761–781.
- van Wezel GP, McDowall KJ. The regulation of the secondary metabolism of Streptomyces: new links and experimental advances. Nat Prod Rep. 2011;28(7):1311–1333.
- Daniel-Ivad M, Pimentel-Elardo S, Nodwell JR. Control of specialized metabolism by signaling and transcriptional regulation: opportunities for new platforms for drug discovery? Annu Rev Microbiol. 2018;72(72):25–48.
- Okada BK, Seyedsayamdost MR. Antibiotic dialogues: induction of silent biosynthetic gene clusters by exogenous small molecules. FEMS Microbiol Rev. 2017;41(1):19–33.
- Kong D, Wang X, Nie J, et al. Regulation of antibiotic production by signaling molecules in Streptomyces. Front Microbiol. 2019;10(2927):2927.
- Xia H, Li X, Li Z, et al. The application of regulatory Cascades in Streptomyces: yield enhancement and metabolite mining. Front Microbiol. 2020;11:406.
- Du C, van Wezel GP. Mining for microbial gems: integrating proteomics in the postgenomic natural product discovery pipeline. Proteomics. 2018;18(18):e1700332.
- van Bergeijk DA, Terlouw BR, Medema MH, et al. Ecology and genomics of actinobacteria: new concepts for natural product discovery. Nat Rev Microbiol. 2020;18(10):546–558.
- Tyurin AP, Alferova VA, Korshun VA. Chemical elicitors of antibiotic biosynthesis in actinomycetes. Microorganisms. 2018;6(2):52.
- Yoon V, Nodwell JR. Activating secondary metabolism with stress and chemicals. J Ind Microbiol Biotechnol. 2014;41(2):415–424.
- Beane WS, Morokuma J, Adams DS, et al. A chemical genetics approach reveals H,K-ATPase-mediated membrane voltage is required for planarian head regeneration. Chem Biol. 2011;18(1):77–89.
- van der Meij A, Worsley SF, Hutchings MI, et al. Chemical ecology of antibiotic production by actinomycetes. FEMS Microbiol Rev. 2017;41(3):392–416.
- Locatelli FM, Goo KS, Ulanova D. Effects of trace metal ions on secondary metabolism and the morphological development of Streptomycetes. Metallomics. 2016;8(5):469–480.
- Wang L, Li Y, Li Y. Metal ions driven production, characterization and bioactivity of extracellular melanin from Streptomyces sp. ZL-24. Int J Biol Macromol. 2019;123:521–530.
- Tanaka Y, Hosaka T, Ochi K. Rare earth elements activate the secondary metabolite-biosynthetic gene clusters in Streptomyces coelicolor A3(2). J Antibiot (Tokyo). 2010;63(8):477–481.
- Kawai K, Wang G, Okamoto S, et al. The rare earth, scandium, causes antibiotic overproduction in Streptomyces spp. FEMS Microbiol Lett. 2007;274(2):311–315.
- Shentu XP, Cao ZY, Xiao Y, et al. Substantial improvement of toyocamycin production in Streptomyces diastatochromogenes by cumulative drug-resistance mutations. PLoS One. 2018;13(8):e0203006.
- Wang C, Huang D, Liang S. Identification and metabolomic analysis of chemical elicitors for tacrolimus accumulation in Streptomyces tsukubaensis. Appl Microbiol Biotechnol. 2018;102(17):7541–7553.
- Braeken K, Moris M, Daniels R, et al. New horizons for (p)ppGpp in bacterial and plant physiology. Trends Microbiol. 2006;14(1):45–54.
- Ochi K, Tanaka Y, Tojo S. Activating the expression of bacterial cryptic genes by rpoB mutations in RNA polymerase or by rare earth elements. J Ind Microbiol Biotechnol. 2014;41(2):403–414.
- Tsuruta T. Accumulation of rare earth elements in various microorganisms. J Rare Earth. 2007;25(5):526–532.
- Tsuruta T. Selective accumulation of light or heavy rare earth elements using gram-positive bacteria. Colloids Surf B Biointerfaces. 2006;52(2):117–122.
- Barka EA, Vatsa P, Sanchez L, et al. Taxonomy, physiology, and natural products of actinobacteria. Microbiol Mol Biol Rev. 2016;80(1):1–43.
- Wang G, Hosaka T, Ochi K. Dramatic activation of antibiotic production in Streptomyces coelicolor by cumulative drug resistance mutations. Appl Environ Microbiol. 2008;74(9):2834–2840.
- Wang S, Liu F, Hou Z, et al. Enhancement of natamycin production on Streptomyces gilvosporeus by chromosomal integration of the Vitreoscilla hemoglobin gene (vgb). World J Microbiol Biotechnol. 2014;30(4):1369–1376.
- Wu H, Liu W, Shi L, et al. Comparative genomic and regulatory analyses of natamycin production of Streptomyces lydicus A02. Sci Rep. 2017;7(1):9114.
- Beniamino Y, Pesce G, Zannoni A, et al. SrnR from Streptomyces griseus is a nickel-binding transcriptional activator. J Biol Inorg Chem. 2020;25(2):187–198.
- Cundliffe E, Butler AR. Influence of dimethylsulfoxide on tylosin production in Streptomyces fradiae. J Ind Microbiol Biotechnol. 2001;27(1):46–51.
- Chen G, Wang GY, Li X, et al. Enhanced production of microbial metabolites in the presence of dimethyl sulfoxide. J Antibiot (Tokyo). 2000;53(10):1145–1153.
- Wang C, Wang J, Yuan J, et al. Generation of Streptomyces hygroscopicus cell factories with enhanced ascomycin production by combined elicitation and pathway-engineering strategies. Biotechnol Bioeng. 2019;116(12):3382–3395.
- Bhatia SK, Lee B-R, Sathiyanarayanan G, et al. Medium engineering for enhanced production of undecylprodigiosin antibiotic in Streptomyces coelicolor using oil palm biomass hydrolysate as a carbon source. Bioresour Technol. 2016;217:141–149.
- Wang J, Wang C, Song K, et al. Metabolic network model guided engineering ethylmalonyl-CoA pathway to improve ascomycin production in Streptomyces hygroscopicus var. ascomyceticus. Microb Cell Fact. 2017;16(1):169.
- Sekurova ON, Zhang J, Kristiansen KA, et al. Activation of chloramphenicol biosynthesis in Streptomyces venezuelae ATCC 10712 by ethanol shock: insights from the promoter fusion studies. Microb Cell Fact. 2016;15:85.
- Doull JL, Ayer SW, Singh AK, et al. Conditions for the production of jadomycin B by Streptomyces venezuelae ISP5230: effects of heat shock, ethanol treatment and phage infection. J Ind Microbiol. 1994;13(2):120–125.
- Zhou WW, Ma B, Tang YJ, et al. Enhancement of validamycin a production by addition of ethanol in fermentation of Streptomyces hygroscopicus 5008. Bioresour Technol. 2012;114:616–621.
- Xu G, Wang J, Wang L, et al. "Pseudo" gamma-butyrolactone receptors respond to antibiotic signals to coordinate antibiotic biosynthesis. J Biol Chem. 2010;285(35):27440–27448.
- Sarkale AM, Kumar A, Appayee C. Organocatalytic approach for short asymmetric synthesis of ( R)-paraconyl alcohol: application to the total syntheses of IM-2, SCB2, and A-Factor γ-butyrolactone autoregulators. J Org Chem. 2018;83(7):4167–4172.
- Ding C, Pan J, Jin M, et al. Enhanced uptake of antibiotic resistance genes in the presence of nanoalumina. Nanotoxicology. 2016;10(8):1051–1060.
- Jiang W, Mashayekhi H, Xing B. Bacterial toxicity comparison between nano- and micro-scaled oxide particles. Environ Pollut. 2009;157(5):1619–1625.
- Deckers AS, Loo S, L'hermite MM, et al. Size-, composition- and shape-dependent toxicological impact of metal oxide nanoparticles and carbon nanotubes toward bacteria. Environ Sci Technol. 2009;43(21):8423–8429.
- Liu X, Tang J, Wang L, et al. Mechanisms of oxidative stress caused by CuO nanoparticles to membranes of the bacterium Streptomyces coelicolor M145. Ecotoxicol Environ Saf. 2018;158:123–130.
- Liu X, Tang J, Wang L, et al. Mechanism of CuO nano-particles on stimulating production of actinorhodin in Streptomyces coelicolor by transcriptional analysis. Sci Rep. 2019;9(1):11253.
- Liu X, Tang J, Wang L, et al. Al2O3 nanoparticles promote secretion of antibiotics in Streptomyces coelicolor by regulating gene expression through the nano effect. Chemosphere. 2019;226:687–695.
- Liu X, Tang J, Wang L, et al. A comparative analysis of ball-milled biochar, graphene oxide, and multi-walled carbon nanotubes with respect to toxicity induction in Streptomyces. J Environ Manage. 2019;243:308–317.
- Qu X, Lei C, Liu W. Transcriptome mining of active biosynthetic pathways and their associated products in Streptomyces flaveolus. Angew Chem Int Ed Engl. 2011;50(41):9651–e9654.
- Sterner DE, Berger SL. Acetylation of histones and transcription-related factors. Microbiol Mol Biol Rev. 2000;64(2):435–459.
- Lombardi PM, Angell HD, Whittington DA, et al. Structure of prokaryotic polyamine deacetylase reveals evolutionary functional relationships with eukaryotic histone deacetylases. Biochemistry. 2011;50(11):1808–1817.
- Gregoretti IV, Lee YM, Goodson HV. Molecular evolution of the histone deacetylase family: functional implications of phylogenetic analysis. J Mol Biol. 2004;338(1):17–31.
- Milne JC, Lambert PD, Schenk S, et al. Small molecule activators of SIRT1 as therapeutics for the treatment of type 2 diabetes. Nature. 2007;450(7170):712–716.
- Moore JM, Bradshaw E, Seipke RF, et al. Use and discovery of chemical elicitors that stimulate biosynthetic gene clusters in Streptomyces bacteria. Methods Enzymol. 2012;517:367–385.
- Rigali S, Titgemeyer F, Barends S, et al. Feast or famine: the global regulator DasR links nutrient stress to antibiotic production by Streptomyces. EMBO Rep. 2008;9(7):670–675.
- Pazin MJ, Kadonaga JT. What's up and down with histone deacetylation and transcription? Cell. 1997;89(3):325–328.
- Wojnarowska Nowak R, Polit J, Sheregii EM. Interaction of gold nanoparticles with cholesterol oxidase enzyme in bionanocomplex—determination of the protein structure by Fourier transform infrared spectroscopy. J Nanopart Res. 2020;22(5):1–14.
- Aparicio JF, Fouces R, Mendes MV, et al. A complex multienzyme system encoded by five polyketide synthase genes is involved in the biosynthesis of the 26-membered polyene macrolide pimaricin in Streptomyces natalensis. Chem Biol. 2000;7(11):895–905.
- Du Y, Chen SF, Cheng LY, et al. Identification of a novel Streptomyces chattanoogensis L10 and enhancing its natamycin production by overexpressing positive regulator ScnRII. J Microbiol. 2009;47(4):506–513.
- Cao B, Yao F, Zheng X, et al. Genome mining of the biosynthetic gene cluster of the polyene macrolide antibiotic tetramycin and characterization of a P450 monooxygenase involved in the hydroxylation of the tetramycin B polyol segment. Chembiochem. 2012;13(15):2234–2242.
- Payero TD, Vicente CM, Rumbero A, et al. Functional analysis of filipin tailoring genes from Streptomyces filipinensis reveals alternative routes in filipin III biosynthesis and yields bioactive derivatives. Microb Cell Fact. 2015;14:114.
- Seco EM, Perez-Zuniga FJ, Rolon MS, et al. Starter unit choice determines the production of two tetraene macrolides, rimocidin and CE-108, in Streptomyces diastaticus var. 108. Chem Biol. 2004;11(3):357–366.
- Aparicio JF, Martin JF. Microbial cholesterol oxidases: bioconversion enzymes or signal proteins? Mol Biosyst. 2008;4(8):804–809.
- Mendes MV, Recio E, Anton N, et al. Cholesterol oxidases act as signaling proteins for the biosynthesis of the polyene macrolide pimaricin. Chem Biol. 2007;14(3):279–290.
- Wang M, Wang S, Zong G, et al. Improvement of natamycin production by cholesterol oxidase overexpression in Streptomyces gilvosporeus. J Microbiol Biotechnol. 2016;26(2):241–247.
- Beites T, Pires SDS, Santos CL, et al. Biotechnological production and application of the antibiotic pimaricin: biosynthesis and its regulation. Appl Microbiol Biotechnol. 2016;100(1):61–78.
- Beld J, Sonnenschein EC, Vickery CR, et al. The phosphopantetheinyl transferases: catalysis of a post-translational modification crucial for life. Nat Prod Rep. 2014;31(1):61–108.
- Kudo F, Kasama Y, Hirayama T, et al. Cloning of the pactamycin biosynthetic gene cluster and characterization of a crucial glycosyltransferase prior to a unique cyclopentane ring formation. J Antibiot. 2007;60(8):492–503.
- Zaleta-Rivera K, Charkoudian LK, Ridley CP, et al. Cloning, sequencing, heterologous expression, and mechanistic analysis of A-74528 biosynthesis. J Am Chem Soc. 2010;132(26):9122–9128.
- Mo X, Wang Z, Wang B, et al. Cloning and characterization of the biosynthetic gene cluster of the bacterial RNA polymerase inhibitor tirandamycin from marine-derived Streptomyces sp. SCSIO1666. Biochem Biophys Res Commun. 2011;406(3):341–347.
- Lombo F, Brana AF, Salas JA, et al. Genetic organization of the biosynthetic gene cluster for the antitumor angucycline oviedomycin in Streptomyces antibioticus ATCC 11891. Chembiochem. 2004;5(9):1181–1187.
- Li A, Piel J. A gene cluster from a marine Streptomyces encoding the biosynthesis of the aromatic spiroketal polyketide griseorhodin A. Chem Biol. 2002;9(9):1017–1026.
- Huang Y, Wendt-Pienkowski E, Shen B. A dedicated phosphopantetheinyl transferase for the Fredericamycin polyketide synthase from Streptomyces griseus. J Biol Chem. 2006;281(40):29660–29668.
- Yan X, Zhang B, Tian W, et al. Puromycin A, B and C, cryptic nucleosides identified from Streptomyces alboniger NRRL B-1832 by PPtase-based activation. Synth Syst Biotechnol. 2018;3(1):76–80.
- Zhang B, Tian W, Wang S, et al. Activation of natural products biosynthetic pathways via a protein modification level regulation. ACS Chem Biol. 2017;12(7):1732–1736.
- Jiang H, Wang Y-Y, Ran X-X, et al. Improvement of natamycin production by engineering of phosphopantetheinyl transferases in Streptomyces chattanoogensis L10. Appl Environ Microbiol. 2013;79(11):3346–3354.
- Wang L, McVey J, Vining LC. Cloning and functional analysis of a phosphopantetheinyl transferase superfamily gene associated with jadomycin biosynthesis in Streptomyces venezuelae ISP5230. Microbiology (Reading). 2001;147(Pt 6):1535–1545.
- Foley TL, Young BS, Burkart MD. Phosphopantetheinyl transferase inhibition and secondary metabolism. FEBS J. 2009;276(23):7134–7145.
- Lambalot R, Gehring A, Flugel R, et al. A new enzyme superfamily-the phosphopantetheinyl transferases. Chem Biol. 1996;3(11):923–936.
- Singh R. Enzymatic control of the related pathways of faty acid and undecylprodiginine biosynthesis in Streptomyces coelicolor. Dissertations and Teses. 2015;Paper :2112.
- Craney A, Ozimok CJ, Pimentel-Elardo SM, et al. Chemical perturbation of secondary metabolism demonstrates important links to primary metabolism. Chem Biol. 2012;19(8):1020–1027.
- Ahmed S, Craney A, Pimentel‐Elardo SM, et al. A synthetic, species-specific activator of secondary metabolism and sporulation in Streptomyces coelicolor. Chembiochem. 2013;14(1):83–91.
- Pimentel-Elardo SM, Sorensen D, Ho L, et al. Activity-independent discovery of secondary metabolites using chemical elicitation and cheminformatic inference. ACS Chem Biol. 2015;10(11):2616–2623.
- Ochi K, Okamoto S. A magic bullet for antibiotic discovery. Chem Biol. 2012;19(8):932–934.
- Zheng M, Storz G. Redox sensing by prokaryotic transcription factors. Biochem Pharmacol. 2000;59(1):1–6.
- Liao Y, Wei ZH, Bai L, et al. Effect of fermentation temperature on validamycin a production by Streptomyces hygroscopicus 5008. J Biotechnol. 2009;142(3–4):271–274.
- Li L, Bai L, Zhou X, et al. Enhanced validamycin production and gene expression at elevated temperature in Streptomyces hygroscopicus subsp. jingangensis 5008. Sci Bull. 2009;54(7):1204–1209.
- Doull JL, Ayer SW, Singh AK, et al. Production of a novel polyketide antibiotic, jadomycin B, by Streptomyces venezuelae following heat shock. J Antibiot (Tokyo). 1993;46(5):869–871.
- Bursy J, Kuhlmann AU, Pittelkow M, et al. Synthesis and uptake of the compatible solutes ectoine and 5-hydroxyectoine by Streptomyces coelicolor A3(2) in response to salt and heat stresses. Appl Environ Microbiol. 2008;74(23):7286–7296.
- Saito S, Kato W, Ikeda H, et al. Discovery of "heat shock metabolites" produced by thermotolerant actinomycetes in high-temperature culture. J Antibiot (Tokyo). 2020;73(4):203–210.
- Yun S-I, Yahya ARM, Cossar D, et al. Temperature downshift increases recombinant cytokine titer in Streptomyces lividans fermentation. Biotechnol Lett. 2001;23(23):1903–1905.
- Schumann W. Regulation of bacterial heat shock stimulons. Cell Stress Chaperones. 2016;21(6):959–968.
- Bucca G, Pothi R, Hesketh A, et al. Translational control plays an important role in the adaptive heat-shock response of Streptomyces coelicolor. Nucleic Acids Res. 2018;46(11):5692–5703.
- Servant P, Mazodier P. Negative regulation of the heat shock response in Streptomyces. Arch Microbiol. 2001;176(4):237–242.
- Wei ZH, Wu H, Bai L, et al. Temperature shift-induced reactive oxygen species enhanced validamycin a production in fermentation of Streptomyces hygroscopicus 5008. Bioprocess Biosyst Eng. 2012;35(8):1309–1316.
- Mo SJ, Kim J, Oh CH. Different effects of acidic pH shock on the prodiginine production in Streptomyces coelicolor M511 and SJM1 mutants. J Microbiol Biotechnol. 2013;23(10):1454–1459.
- Hayes A, Hobbs G, Smith C, et al. Environmental signals triggering methylenomycin production by Streptomyces coelicolor A3(2). J Bacteriol. 1997;179(17):5511–5515.
- Kim YJ, Song JY, Moon MH, et al. pH shock induces overexpression of regulatory and biosynthetic genes for actinorhodin productionin Streptomyces coelicolor A3(2). Appl Microbiol Biotechnol. 2007;76(5):1119–1130.
- Pan L, Chen XS, Liu MM, et al. Efcient production of ε-poly-l-lysine from glucose by two-stage fermentation using pH shock strategy. Process Biochem. 2017;63:8–15.
- Jiang J, Sun YF, Tang X, et al. Alkaline pH shock enhanced production of validamycin a in fermentation of Streptomyces hygroscopicus. Bioresour Technol. 2018;249:234–240.
- Yeo KJ, Hong YS, Jee JG, et al. Mechanism of the pH-induced conformational change in the sensor domain of the DraK histidine kinase via the E83, E105, and E107 residues. PLoS One. 2014;9(9):e107168.
- Kim YJ, Moon MH, Lee JS, et al. Roles of putative sodium-hydrogen antiporter (SHA) genes in S. coelicolor A3(2) culture with pH variation. J Microbiol Biotechnol. 2011;21(9):979–987.
- Pan L, Chen X, Wang K, et al. Understanding high ε-poly-l-lysine production by Streptomyces albulus using pH shock strategy in the level of transcriptomics. J Ind Microbiol Biotechnol. 2019;46(12):1781–1792.
- Shioya S, Morikawa M, Kajihara Y, et al. Optimization of agitation and aeration conditions for maximum virginiamycin production. Appl Microbiol Biotechnol. 1999;51(2):164–169.
- Mehmood N, Olmos E, Goergen JL, et al. Oxygen supply controls the onset of pristinamycins production by Streptomyces pristinaespiralis in shaking flasks. Biotechnol Bioeng. 2011;108(9):2151–2161.
- Kaiser D, Onken U, Sattler I, et al. Influence of increased dissolved oxygen concentration on the formation of secondary metabolites by manumycin-producing Streptomyces parvulus. Appl Microbiol Biotechnol. 1994;41(3):309–312.
- MartinsL RA, Guimarães M, Pamboukian CR, et al. The effect of dissolved oxygen concentration control on cell growth and antibiotic retamycin production in Streptomyces olindensis So20 fermentations. Braz J Chem Eng. 2004;21(2): 185–192.
- Gallagher KA, Wanger G, Henderson J, et al. Ecological implications of hypoxia-triggered shifts in secondary metabolism. Environ Microbiol. 2017;19(6):2182–2191.
- Gamboa-Suasnavart RA, Valdez-Cruz NA, Gaytan-Ortega G, et al. The metabolic switch can be activated in a recombinant strain of Streptomyces lividans by a low oxygen transfer rate in shake flasks. Microb Cell Fact. 2018;17(1):189.
- Nieselt K, Battke F, Herbig A, et al. The dynamic architecture of the metabolic switch in Streptomyces coelicolor. BMC Genomics. 2010;11:10.
- Brakhage AA, Schroeckh V. Fungal secondary metabolites – strategies to activate silent gene clusters. Fungal Genet Biol. 2011;48(1):15–22.
- Ueda K, Kawai S, Ogawa HO, et al. Wide distribution of interspecific stimulatory events on antibiotic production and sporulation among Streptomyces species. J Antibiot (Tokyo). 2000;53(9):979–982.
- Wang W, Yu L, Zhou P. Effects of different fungal elicitors on growth, total carotenoids and astaxanthin formation by Xanthophyllomyces dendrorhous. Bioresour Technol. 2006;97(1):26–31.
- Pettit RK. Small-molecule elicitation of microbial secondary metabolites. Microb Biotechnol. 2011;4(4):471–478.
- Wang D, Yuan J, Gu S, et al. Influence of fungal elicitors on biosynthesis of natamycin by Streptomyces natalensis HW-2. Appl Microbiol Biotechnol. 2013;97(12):5527–5534.
- Shi S, Tao Y, Liu W. Effects of fungi fermentation broth on natamycin production of Streptomyces. Prog Appl Microbiol. 2017;1:15–22.
- Douglas LM, Konopka JB. Fungal membrane organization: the eisosome concept. Annu Rev Microbiol. 2014;68(1):377–393.
- Wang D, Wei L, Zhang Y, et al. Physicochemical and microbial responses of Streptomyces natalensis HW-2 to fungal elicitor. Appl Microbiol Biotechnol. 2017;101(17):6705–6712.
- Zhao X, Pang H, Wang S, et al. Structural basis for prokaryotic calcium-mediated regulation by a Streptomyces coelicolor calcium binding protein. Protein Cell. 2010;1(8):771–779.
- Römling U, Galperin MY, Gomelsky M. Cyclic di-GMP: the first 25 years of a universal bacterial second messenger. Microbiol Mol Biol Rev. 2013;77(1):1–52.
- Sies H, Berndt C, Jones DP. Oxidative stress. Annu Rev Biochem. 2017;86(1):715–748.
- Kinkel LL, Schlatter DC, Bakker MG, et al. Streptomyces competition and co-evolution in relation to plant disease suppression. Res Microbiol. 2012;163(8):490–499.
- Charusanti P, Fong NL, Nagarajan H, et al. Exploiting adaptive laboratory evolution of Streptomyces clavuligerus for antibiotic discovery and overproduction. PLoS One. 2012;7(3):e33727.
- Imai Y, Sato S, Tanaka Y, et al. Lincomycin at subinhibitory concentrations potentiates secondary metabolite production by Streptomyces spp. Appl Environ Microbiol. 2015;81(11):3869–3879.
- Onaka H, Mori Y, Igarashi Y, et al. Mycolic acid-containing bacteria induce natural-product biosynthesis in Streptomyces species. Appl Environ Microbiol. 2011;77(2):400–406.
- Hoshino S, Zhang L, Awakawa T, et al. Arcyriaflavin E, a new cytotoxic indolocarbazole alkaloid isolated by combined-culture of mycolic acid-containing bacteria and Streptomyces cinnamoneus NBRC 13823. J Antibiot (Tokyo). 2015;68(5):342–344.
- Hoshino S, Wakimoto T, Onaka H, et al. Chojalactones A-C, cytotoxic butanolides isolated from Streptomyces sp. cultivated with mycolic acid containing bacterium. Org Lett. 2015;17(6):1501–1504.
- Sugiyama R, Nishimura S, Ozaki T, et al. Discovery and total synthesis of streptoaminals: antimicrobial [5,5]-spirohemiaminals from the combined-culture of Streptomyces nigrescens and Tsukamurella pulmonis. Angew Chem Int Ed Engl. 2016;55(35):10278–10282.
- Sugiyama R, Nishimura S, Ozaki T, et al. 5-Alkyl-1,2,3,4-tetrahydroquinolines, new membrane-interacting lipophilic metabolites produced by combined culture of Streptomyces nigrescens and Tsukamurella pulmonis. Org Lett. 2015;17(8):1918–1921.
- Zou Z, Du D, Zhang Y, et al. A γ-butyrolactone-sensing activator/repressor, JadR3, controls a regulatory mini-network for jadomycin biosynthesis. Mol Microbiol. 2014;94(3):490–505.
- Nodwell JR. Are you talking to me? A possible role for γ-butyrolactones in interspecies signalling. Mol Microbiol. 2014;94(3):483–485.
- Westhoff S, Kloosterman AM, Hoesel S, et al. Competition sensing changes antibiotic production in Streptomyces. mBio. 2021;12(1): e02729–e02720.
- D'Autréaux B, Toledano MB. ROS as signalling molecules: mechanisms that generate specificity in ROS homeostasis. Nat Rev Mol Cell Biol. 2007;8(10):813–824.
- Kanth BK, Jnawali HN, Niraula NP, et al. Superoxide dismutase (SOD) genes in Streptomyces peucetius: effects of SODs on secondary metabolites production. Microbiol Res. 2011;166(5):391–402.
- Hahn JS, Oh SY, Chater KF, et al. H2O2-sensitive fur-like repressor CatR regulating the major catalase gene in Streptomyces coelicolor. J Biol Chem. 2000;275(49):38254–38260.
- Beites T, Pires SDS, Santos CL, et al. Crosstalk between ROS homeostasis and secondary metabolism in S. natalensis ATCC 27448: modulation of pimaricin production by intracellular ROS. PLoS One. 2011;6(11):e27472.
- Kwon HJ, Kim SU. Enhanced biosynthesis of clavulanic acid in Streptomyces clavuligerus due to oxidative challenge by redox-cycling agents. Appl Microbiol Biotechnol. 1998;49(1):77–83.
- Kwon HJ, Kim SU. Molecular basis for enhanced biosynthesis of clavulanic acid by a redox-cycling agent, phenazine methosulfate, in Streptomyces clavuligerus. Appl Microbiol Biotechnol. 1999;53(1):57–62.
- Dubbs JM, Mongkolsuk S. Peroxide-sensing transcriptional regulators in bacteria. J Bacteriol. 2012;194(20):5495–5503.
- Shin JH, Singh AK, Cheon DJ, et al. Activation of the SoxR regulon in Streptomyces coelicolor by the extracellular form of the pigmented antibiotic actinorhodin. J Bacteriol. 2011;193(1):75–81.
- Cruz RD, Gao Y, Penumetcha S, et al. Expression of the Streptomyces coelicolor SoxR regulon is intimately linked with actinorhodin production. J Bacteriol. 2010;192(24):6428–6438.
- Cheng Y, Yang R, Lyu M, et al. IdeR, a DtxR family iron response regulator, controls iron homeostasis, morphological differentiation, secondary metabolism, and the oxidative stress response in Streptomyces avermitilis. Appl Environ Microbiol. 2018;84(22):e01503–e01518.
- Zeng X, Chen XS, Gao Y, et al. Continuously high reactive oxygen species generation decreased the specific ϵ-poly- l -lysine formation rate in fed-batch fermentation using glucose and glycerol as a mixed carbon source. Process Biochem. 2015;50(12):1993–2003.
- Yan P, Sun H, Lu P, et al. Enhancement of ε-poly-L-lysine synthesis in Streptomyces by exogenous glutathione. Bioprocess Biosyst Eng. 2018;41(1):129–134.
- Romero D, Traxler MF, Lopez D, et al. Antibiotics as signal molecules. Chem Rev. 2011;111(9):5492–5505.
- Chiang Y, Chang S, Oakley BR, et al. Recent advances in awakening silent biosynthetic gene clusters and linking orphan clusters to natural products in microorganisms. Curr Opin Chem Biol. 2011;15(1):137–143.
- Yim G, Wang HH, Davies J. Antibiotics as signalling molecules. Philos Trans R Soc Lond B Biol Sci. 2007;362(1483):1195–1200.
- Hosaka T, Ohnishi-Kameyama M, Muramatsu H, et al. Antibacterial discovery in actinomycetes strains with mutations in RNA polymerase or ribosomal protein S12. Nat Biotechnol. 2009;27(5):462–464.
- Tanaka Y, Tokuyama S, Ochi K. Activation of secondary metabolite-biosynthetic gene clusters by generating rsmG mutations in Streptomyces griseus. J Antibiot (Tokyo). 2009;62(12):669–673.
- Tanaka Y, Kasahara K, Hirose Y, et al. Activation and products of the cryptic secondary metabolite biosynthetic gene clusters by rifampin resistance (rpoB) mutations in actinomycetes. J Bacteriol. 2013;195(13):2959–2970.
- Ma Z, Luo S, Xu X, et al. Characterization of representative rpoB gene mutations leading to a significant change in toyocamycin production of Streptomyces diastatochromogenes 1628. J Ind Microbiol Biotechnol. 2016;43(4):463–471.
- Wang L, Li S, Zhao J, et al. Efficiently activated ε-poly-L-lysine production by multiple antibiotic-resistance mutations and acidic pH shock optimization in Streptomyces albulus. Microbiologyopen. 2019;8(5):e00728.
- Inaoka T, Ochi K. Activation of dormant secondary metabolism neotrehalosadiamine synthesis by an RNA polymerase mutation in Bacillus subtilis. Biosci Biotechnol Biochem. 2011;75(4):618–623.
- Shentu X, Liu N, Tang G, et al. Improved antibiotic production and silent gene activation in Streptomyces diastatochromogenes by ribosome engineering. J Antibiot (Tokyo). 2016;69(5):406–410.
- Covington BC, Spraggins JM, Ynigez-Gutierrez AE, et al. Response of secondary metabolism of hypogean actinobacterial genera to chemical and biological stimuli. Appl Environ Microbiol. 2018;84(19):e01125–e01118.
- Ozaki T, Yamashita K, Goto Y, et al. Dissection of goadsporin biosynthesis by in vitro reconstitution leading to designer analogues expressed in vivo. Nat Commun. 2017;8:14207.
- Onaka H. Novel antibiotic screening methods to awaken silent or cryptic secondary metabolic pathways in actinomycetes. J Antibiot (Tokyo). 2017;70(8):865–870.
- Onaka H. Biosynthesis of indolocarbazole and goadsporin, two different heterocyclic antibiotics produced by actinomycetes. Biosci Biotechnol Biochem. 2009;73(10):2149–2155.
- Onaka H, Tabata H, Igarashi Y, et al. Goadsporin, a chemical substance which promotes secondary metabolism and morphogenesis in Streptomycetes. I. Purification and characterization. J Antibiot (Tokyo). 2001;54(12):1036–1044.
- Onaka H, Nakaho M, Hayashi K, et al. Cloning and characterization of the goadsporin biosynthetic gene cluster from Streptomyces sp. TP-A0584. Microbiology (Reading). 2005;151(Pt 12):3923–3933.
- Xu F, Nazari B, Moon K, et al. Discovery of a cryptic antifungal compound from Streptomyces albus J1074 using high-throughput elicitor screens. J Am Chem Soc. 2017;139(27):9203–9212.
- Seyedsayamdost MR. High-throughput platform for the discovery of elicitors of silent bacterial gene clusters. Proc Natl Acad Sci U S A. 2014;111(20):7266–7271.
- Moon K, Xu F, Zhang C, et al. Bioactivity-HiTES unveils cryptic antibiotics encoded in actinomycete bacteria. ACS Chem Biol. 2019;14(4):767–774.
- Xu F, Wu Y, Zhang C, et al. A genetics-free method for high-throughput discovery of cryptic microbial metabolites. Nat Chem Biol. 2019;15(2):161–168.
- Yuan C, Guo YH, Wang HY, et al. Allelopathic polyketides from an endolichenic fungus Myxotrichum SP. by using OSMAC strategy. Sci Rep. 2016;6(1):19350.
- Hemphill CFP, Sureechatchaiyan P, Kassack MU, et al. OSMAC approach leads to new fusarielin metabolites from Fusarium tricinctum. J Antibiot (Tokyo). 2017;70(6):726–732.
- Selegato DM, Freire RT, Pilon AC, et al. Improvement of bioactive metabolite production in microbial cultures – a systems approach by OSMAC and deconvolution-based 1 HNMR quantification. Magn Reson Chem. 2019;57(8):458–471.
- Yue Y, Yu H, Li R, et al. Exploring the antibacterial and antifungal potential of jellyfish-associated marine fungi by cultivation-dependent approaches. PLoS One. 2015;10(12):e0144394.
- Romano S, Jackson S, Patry S, et al. Extending the “one strain many compounds” (OSMAC) principle to marine microorganisms. Mar Drugs. 2018;16(7):244.
- Zazopoulos E, Huang K, Staffa A, et al. A genomics-guided approach for discovering and expressing cryptic metabolic pathways. Nat Biotechnol. 2003;21(2):187–190.
- Pan R, Bai X, Chen J, et al. Exploring structural diversity of microbe secondary metabolites using OSMAC strategy: a literature review. Front Microbiol. 2019;10(294):294.
- Wu Q, Zhang G, Wang B, et al. Production and identification of inthomycin B produced by a deep-sea sediment-derived Streptomyces sp. YB104 based on cultivation-dependent approach. Curr Microbiol. 2018;75(7):942–951.
- Lopez JAV, Nogawa T, Futamura Y, et al. Nocardamin glucuronide, a new member of the ferrioxamine siderophores isolated from the ascamycin-producing strain Streptomyces sp. 80H647. J Antibiot (Tokyo). 2019;72(12):991–995.
- McAlpine JB, Bachmann BO, Piraee M, et al. Microbial genomics as a guide to drug discovery and structural elucidation: ECO-02301, a novel antifungal agent, as an example. J Nat Prod. 2005;68(4):493–496.
- Sproule A, Correa H, Decken A, et al. Terrosamycins a and B, bioactive polyether ionophores from Streptomyces sp. RKND004 from Prince Edward Island sediment. Mar Drugs. 2019;17(6):347.
- Tangerina MMP, Furtado LC, Leite VMB, et al. Metabolomic study of marine Streptomyces sp.: secondary metabolites and the production of potential anticancer compounds. PLoS One. 2020;15(12):e0244385.
- Bachmann BO, Van Lanen SG, Baltz RH. Microbial genome mining for accelerated natural products discovery: is a renaissance in the making? J Ind Microbiol Biotechnol. 2014;41(2):175–184.
- Almeida E, Kaur N, Jennings L, et al. Genome mining coupled with OSMAC-based cultivation reveal differential production of surugamide a by the marine sponge isolate Streptomyces sp. SM17 when compared to its terrestrial relative S. albidoflavus J1074. Microorganisms. 2019;7(10):394.
- Fang Q, Wu L, Urwald C, et al. Genomic scanning enabling discovery of a new antibacterial bicyclic carbamate-containing alkaloid. Synth Syst Biotechnol. 2021;6(1):12–19.
- Machushynets NV, Wu C, Elsayed SS, et al. Discovery of novel glycerolated quinazolinones from Streptomyces sp. MBT27. J Ind Microbiol Biotechnol. 2019;46(3–4):483–492.
- Ding H, Wang J, Zhang D, et al. Derivatives of holomycin and cyclopropaneacetic acid from Streptomyces sp. DT-A37. Chem Biodivers. 2017;14(9):e1700140.
- English A, Boufridi A, Quinn RJ, et al. Evaluation of fermentation conditions triggering increased antibacterial activity from a near-shore marine intertidal environment-associated Streptomyces species. Synth Syst Biotechnol. 2017;2(1):28–38.
- Rigali S, Anderssen S, Naome A, et al. Cracking the regulatory code of biosynthetic gene clusters as a strategy for natural product discovery. Biochem Pharmacol. 2018;153:24–34.
- Gordon G. Biological activity of recently discovered halogenated marine natural products. Mar Drugs. 2015;13(7):4044–4136.