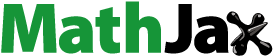
Abstract
Exponential increasing demands for base metals have made meaningful processing of their quite low-grade (>1%) resources. Froth flotation is the most important physicochemical pretreatment technique for processing low-grade sulfide ores. In other words, flotation separation can effectively upgrade finely liberated base metal sulfides based on their surface properties. Various sulfide surface characters can be modified by flotation surfactants (collectors, activators, depressants, pH regulators, frothers, etc.). However, these reagents are mostly toxic. Therefore, using biodegradable flotation reagents would be essential for a green transition of ore treatment plants, while flotation circuits deal with massive volumes of water and materials. Pyrite, the most abundant sulfide mineral, is frequently associated with valuable minerals as a troublesome gangue. It causes severe technical and environmental difficulties. Thus, pyrite should be removed early in the beneficiation process to minimize its problematic issues. Recently, conventional inorganic pyrite depressants (such as cyanide, lime, and sulfur-oxy compounds) have been successfully assisted or even replaced with eco-friendly and green reagents (including polysaccharide-based substances and biodegradable acids). Yet, no comprehensive review is specified on the biodegradable acid depression reagents (such as tannic, lactic, humic acids, etc.) for pyrite removal through flotation separation. This study has comprehensively reviewed the previously conducted investigations in this area and provides suggestions for future assessments and developments. This robust review has systematically explored depression performance, various adsorption mechanisms, and aspects of these reagents on pyrite surfaces. Furthermore, factors affecting their efficiency were analyzed, and gaps within each area were highlighted.
Introduction
Froth flotation as a physicochemical pretreatment technique is the most common and effective beneficiation method to enrich low-grade metal minerals. It assists in selectively separating valuable minerals from their non-valuable associated minerals (gangue phases) based on their surface properties [Citation1–3]. In flotation beneficiation, by using different surfactants (reagents: collectors, activators, depressants, pH regulators, frothers, etc.), the surface characteristics of various minerals would be modified (making hydrophobic or hydrophilic), and they can selectively be separated based on their floatability [Citation4,Citation5,Citation6] Since the grade of valuable metals from their primary resources has markedly decreased, and their demands have significantly increased, flotation separation deals with a tremendous amount of water and materials through the upgrading process. Thus, flotation reagents’ toxicity could be considered a substantial environmental challenge for processing plants (especially for pyrite () rejection) [Citation7].
Pyrite is the most abundant iron-bearing sulfide mineral. It is frequently observed in: massive hydrothermal deposits, veins and replacements, igneous rocks, and sedimentary beds. Pyrite can be occasionally hosted in precious elements such as gold; thus, it sometimes recovers and concentrates [Citation8,Citation9,Citation10–13]. Its various accompanying types of ore deposits (including: nonferrous base metals, precious metals, coal, and rare earth elements) impose technical difficulties and environmental problems during beneficiation processes [Citation14–18,Citation19,Citation20]. The reaction of pyrite with atmospheric oxygen and moisture leads to acid mine drainage (AMD) (EquationEquation (1)(1)
(1) ). AMD can acidify the adjacent aqueous zones and streams. Also, it can increase the concentration of hazardous heavy metals and metalloids [Citation21–23].
(1)
(1)
Moreover, the presence of pyrite in the base metal concentrates leads to a reduction in grade and quality as well as a rise in iron and sulfur content, leading to more smelting expenses [Citation24,Citation25]. Pyrite can potentially contain heavy metals, such as arsenic, and cause harmful dust and fumes through thermal processes, such as smelting [Citation26–29]. Coal deposits may also have a high pyrite content, which is essential to remove. Otherwise, burning coal produces SO2 and releases it into the atmosphere [Citation28–32], and results in an acid rain disaster [Citation30,Citation31]. Furthermore, in beneficiating base metals’ concentration by hydrometallurgical methods, pyrite can contaminate the solution with iron ions as a troublesome impurity [Citation32]. Thus, removing the pyrite content is a crucial pretreatment step in the beneficiation process of many ore deposits [Citation33].
Flotation separation has been widely used to selectively remove pyrite early in the beneficiation process (before hydro- or pyrometallurgical operations) [Citation34,Citation35]. Due to the natural hydrophobicity of pyrite particles, depressants play a significant role in deactivating the pyrite surface and separating it from other valuable hydrophobic minerals [Citation36,Citation37]. Conventional depression reagents used to deactivate pyrite surface are cyanide, lime, and sulfur-oxy species [Citation34,Citation35,Citation38–44]. Even though these inorganic depressants have been highly influential, their usage can lead to high costs and environmental concerns [Citation45,Citation46]. For example, sodium cyanide (as the most toxic chemical among mentioned reagents for pyrite depression) can be quite harmful to humans and the environment. The precipitated cyanide in the tailing dams could be a source of underground water pollution and an immense hazard to the environment in the case of dam damage or collapse [Citation47]. Therefore, nonbiodegradable, toxic flotation reagents may accumulate in plant and animal species through the bioaccumulation process. Therefore, biodegradable reagents for pyrite depression have gained substantial attention. Since froth flotation is the most important mineral enrichment method and reagents play a remarkable role in making the process selective, flotation reagent properties would have an essential impact on nature. Reducing consumption or replacing environmentally harmful substances with eco-friendly ones would be a significant practical step in moving toward green transition and sustainable development [Citation48].
The inhibitory effect of polysaccharide-based depressants (such as starch, dextrin, chitosan, guar gum, locust bean gum, etc.) as biodegradable reagents on pyrite surface has been partially investigated [Citation49–51]. Polysaccharide-based depressants have shown satisfactory performance for the depression of pyrite in various flotation systems. However, due to the continuous decrease in the grade and quality of various ore deposits and the increased environmental restrictions, it would be beneficial to introduce other eco-friendly depressants to replace or assist inorganic depression. Thus, recently several investigations have been conducted on the depression behavior of various biodegradable acid-based substances (such as lactic acid, tannic acid, etc.) on pyrite floatability. However, there is still a need-to-be-filled gap in the existence of a robust review article to comprehensively explore various adsorption aspects and facilitate an understanding of pyrite surface interactions with these green depressants. As a unique approach, the present review has effectively scrutinized the performance of biodegradable acids used as green depressants to separate pyrite from valuable minerals through the flotation technique. Numerous parameters that need to be considered for their applications have been discussed and assessed. Adsorption mechanisms and gaps within each area were analyzed and highlighted.
Main challenges for pyrite depression
Natural floatability-high collector adsorption affinity
Pyrite is a naturally hydrophobic mineral that can be floated without adding any collecting agent (collectors which make surface hydrophobic) under specific conditions. Additionally, its high affinity to xanthates (the most common collectors for sulfide minerals) causes it to be recovered in the froth zone, reducing the quality and grade of the valuable minerals (chalcopyrite “CuFeS2,” sphalerite “ZnS,” galena “PbS,” etc.) [Citation52,Citation36]. It has been reported that the process of xanthate adsorption onto the pyrite surface occurs through four main steps, including (1) oxidation of pyrite surface, (2) adsorption of xanthate ions onto pyrite surface, which leads to ferric xanthates formation, (3) oxidation of xanthate ions to di-xanthogen, (4) xanthate collector adsorption through interaction between ferric xanthates (on pyrite) and di-xanthogen [Citation53].
Electrochemical-potential
As many other metallic sulfides, pyrite is a semiconductor mineral. This leads to occurring various electrochemical reactions during flotation separation when pyrite is in the system [Citation54–58]. The rate of pyrite oxidation is the function of several parameters, including: pH, electrochemical potential, the type and dosage of oxidant, particle size, temperature, and stirring speed [Citation59–64]. It has been reported that the electrochemical potential of the slurry/particle interface is the most significant parameter that affects flotation systems containing pyrite [Citation65,Citation66]. The electrochemical-potential controls for the formation of surface species are enhancing pyrite floatability (such as polysulfides, elemental sulfur, and xanthate), and also surface species are depressing the pyrite surface (such as ferric oxide/hydroxide and sulfate) [Citation36]. These species are the products of the electrochemical oxidation of pyrite in aqueous solutions (). It is also worth mentioning that various pyrite samples are different in surface morphology, Fe/S ratio, and crystal structure, resulting in a different electrochemical reactivity [Citation8,Citation67].
Figure 2. Eh-pH diagram for FeS2–H2O system at 25 °C and 10−5 M dissolved species [Citation63].
![Figure 2. Eh-pH diagram for FeS2–H2O system at 25 °C and 10−5 M dissolved species [Citation63].](/cms/asset/58934a06-18a4-4c58-8499-144cf8c4d381/ibty_a_2238885_f0002_c.jpg)
Activation of pyrite
Pyrite without activation could be easily depressed at high pH levels. In general, the floatability of pyrite is highly pH-dependent, and it would be depressed by increasing pH (pH> 9) [Citation68]. However, the selective separation of pyrite in flotation systems is challenging because of its unintended activation. The activation can occur when metallic cations, more specifically Cu2+ and Pb2+, are in the flotation solution (come from associated copper and lead sulfides) [Citation69–72,Citation36,Citation37,Citation73,Citation74]. Cu2+ ions can electrochemically activate the pyrite surface. This process comprises one step in which copper cations quickly adsorb on the reactive sulfur sites of the pyrite surface. During the copper adsorption, cupric ion (Cu2+) reduces to cuprous (Cu+). In this reaction, Cu(I)-sulfide compounds and elemental sulfur are formed () [Citation75,Citation38,Citation76,Citation77]. Unlike copper activation, in the process of pyrite activation by lead cations, an electrochemical reaction does not occur. Some Pb-containing compounds (hydroxides or carbonates) precipitate on the pyrite surface during the lead activation process. The precipitation of these species is highly pH-dependent [Citation78]. Since these substances precipitated on the pyrite surface during the undesirable activation process have a strong affinity for xanthate ions, this phenomenon can disturb the pyrite depression system [Citation37,Citation69,Citation79].
Figure 3. Pyrite activation in the presence of (A) Cu2+ and (B) Pb2+ cations [Citation75].
![Figure 3. Pyrite activation in the presence of (A) Cu2+ and (B) Pb2+ cations [Citation75].](/cms/asset/dd21e247-2123-4344-a326-13952ffbd104/ibty_a_2238885_f0003_c.jpg)
Galvanization
The simultaneous presence of species with different rest potentials in the flotation system can cause galvanization. This electrochemical interaction may also occur between minerals and the grinding media [Citation72,Citation80–82]. One of the most traditional flotation processes is the separation of pyrite (which has to be depressed) from chalcopyrite. In this system, pyrite that possesses more rest potential than chalcopyrite plays a cathodic role in electrochemical reaction (). Therefore, pyrite is galvanically protected, and chalcopyrite (anode) is oxidized [Citation72,Citation83,Citation84]. It has been proven that when these minerals are galvanically coupled, the floatability of pyrite could be promoted, but chalcopyrite recovery would be reduced [Citation72].
Figure 4. Galvanization process between pyrite (cathode) and chalcopyrite (anode) [Citation72].
![Figure 4. Galvanization process between pyrite (cathode) and chalcopyrite (anode) [Citation72].](/cms/asset/b960bca5-38bc-488e-a712-f550bff581a7/ibty_a_2238885_f0004_c.jpg)
Biodegradable acids
Biodegradable acids (BAs) () are comparatively weak acidic compounds that do not completely dissociate in water [Citation85,Citation86]. BAs are widely distributed in nature as they can generate from: animals, plants, fungi, yeasts, and bacteria. They contain one or more carboxylic acid groups, which could be covalently linked into amides, esters, and peptides [Citation87]. The strength of BAs depends on the functionalities, such as carboxyl (–COOH), sulfonic (–SO3−), hydroxyl (–OH), etc. [Citation85,Citation88]. With the focus on green processes and the restrictions imposed on mining activities, these naturally occurring and eco-friendly chemicals have received more attention than before. Several investigations have been conducted to use them as pyrite depressants during flotation ().
Table 1. Biodegradable acids were used as a flotation reagent for depressing pyrite.
Table 2. Application of biodegradable acids as green depression reagents in pyrite flotation.
These studies indicated that pyrite’s isoelectric point (iep) could be varied in a wide pH range. This variation depends on the oxidation rate of the pyrite surface and has been reported from pH 2 (non-oxidized pyrite) to 7 (fully oxidized pyrite) [Citation99]. Reagents impact on the pyrite’s electrical surface charge (). It was found that LA and SA () can adsorb on the pyrite surface through simultaneous physical and chemical mechanisms and increase the iep on the pyrite surface [Citation89,Citation93]. However, other organic depressants (PGA, PA, NaHu, PCA, and TA) significantly decrease pyrite iep [Citation90–92,Citation94,Citation97,Citation98]. Zeta potential measurements revealed that the presence of Ca2+ ions (originated from lime dissolution) decreases the negative charge on the pyrite surface [Citation91].
Table 3. The isoelectric point of pyrite with and without the addition of biodegradable acids.
Lactic acid
As a monocarboxylic organic acid (C3H6O3), lactic acid (LA) is a widely used chemical substance with various applications (such as acidity regulator, food preservative, fragrance, anti-microbial agent, etc.). Since LA production can be performed through a biotechnological process (using herbal wastes and residues), it can be considered a renewable and environmentally friendly chemical substance [Citation100]. It was reported that LA addition considerably affected the number of sulfur species on the pyrite surface, which is beneficial for mineral depression [Citation101,Citation102]. Han et al. [Citation89] utilized LA as an effective depressant for separating pyrite from chalcopyrite in froth flotation (pH 8) when sodium butyl xanthate (SBX) was used as a collector. LA showed a satisfactory depression impact on pyrite particles, while chalcopyrite floatability was not affected (). Adsorption analyses revealed that LA reduced the adsorption amount of SBX on the surface of pyrite particles with negligible effect on the chalcopyrite mineral. Zeta potential and Infrared spectroscopy (IR) analyses demonstrated that the LA adsorption onto the pyrite surface occurred through chemical and physical mechanisms. LA adsorption on the pyrite surface formed a considerable quantity of hydrophilic compounds, increasing the pyrite hydrophilicity. Time-of-Flight secondary ion mass spectrometry (TOF-SIMS) and X-ray photoelectron spectroscopy (XPS) outcomes showed that LA significantly interacted with iron sites located on the surface of pyrite. This led to hydrophilic iron hydroxide forming on the pyrite surface [Citation89]. XPS analysis can distinguish the elemental components and chemical states of produced species of mineral-reagent reaction [Citation103]. The XPS results revealed that the pretreated surface of pyrite has a higher Fe concentration (13.40%) than the fresh sample (7.18%). The rise in iron sites on the surface of pyrite led to enhancing the formation of hydrophilic iron-oxy/hydroxy compounds. Also, after treatment with LA, the hydrophobic sulfur species decreased from 13.46% to 9.59%. These phenomena enhanced the hydrophilicity of the pyrite surface [Citation89].
Figure 5. The adsorption amount of SBX onto the surfaces of chalcopyrite and pyrite as a function of LA dosage (pH: 8, SBX: 10 mg/L) [Citation89].
![Figure 5. The adsorption amount of SBX onto the surfaces of chalcopyrite and pyrite as a function of LA dosage (pH: 8, SBX: 10 mg/L) [Citation89].](/cms/asset/0a3e55d2-9d20-4d99-a393-7c5903d698d0/ibty_a_2238885_f0005_c.jpg)
Polyglutamic acid
Polyglutamic acid (PGA), which is also named poly-γ-glutamic acid (γ-PGA), is a polymeric chemical derived from glutamic acid (GA). PGA molecule structure contains d-and l-GA units, which have been bound through amide connections between a-amino and g-carboxylic acid functionalities [Citation104–106]. PGA is an anionic renewable biopolymer with high solubility in water [Citation106,Citation107] and can be produced through the fermentation process by microorganisms. This method can be commercially used because of its: lower negative impact on the environment, higher purity of PGA, and milder production conditions than other techniques [Citation104]. However, the extensive use of this technology is restricted by its high production cost [Citation104,Citation107]. For PGA synthesis, glucose molecules (C6H12O6) need to be converted into L-GA at the first step (EquationEquation (2)(2)
(2) ). Subsequently, L-GA is racemized into D-GA via L-alanine and D-alanine. Lastly, D-GA and L-GA are incorporated into the growing biopolymer of PGA [Citation104].
(2)
(2)
Since PGA is a biodegradable biomolecule [Citation107], its degradation starts with its initial disintegration into D- and L-GA. More oxidation of GAs leads to the formation of ammonia water (NH4OH) and carboxylic acids (such as pyruvic, maleic, and oxalic acids). In the final step, produced carboxylic acids are gradually converted into H2O and CO2. PGA is biodegradable and nontoxic; thus, its optimal usage in mineral processing systems can considerably decrease the environmental threats of using inorganic materials [Citation106,Citation107].
As a green depressant, PGA can be used to selectively separate pyrite from chalcopyrite through the flotation technique (SBX was the collector). The depressive performance of PGA on the pyrite surface was significantly stronger than that on chalcopyrite at a pH range of 8–10 (acts as a selective depressant). The highest efficiency was observed in artificially mixed samples at pH 9 (recovery was ∼20% for pyrite and ∼85% for chalcopyrite). Adsorption assessments indicated a higher amount of PGA on the pyrite surface than on the chalcopyrite surface, which was also confirmed by zeta potential results. XPS and IR analyses indicated that PGA chemically interacted with the pyrite surface. Through the pretreatment, a strong layer of PGA formed on the pyrite surface; thus, its surface was passivated and could not adsorb SBX. On the other hand, the interaction between PGA and chalcopyrite was weak, and xanthate ions from SBX could effectively be oxidized to di-xanthogen on the surface of chalcopyrite, improving its floatability in PGA presence [Citation90].
Khoso et al. [Citation91] investigated the combined effect of PGA and lime in a pyrite-chalcopyrite flotation system (SBX as a collector). It was reported that the pretreatment of pyrite using lime and PGA could form hydrophilic compounds on its surface. This phenomenon blocked the electrochemical reaction between the pyrite surface and xanthate ions, which prevented di-xanthogen formation. This mixed depressant showed a slight inhibitory impact on chalcopyrite particles. Therefore, a significant recovery difference (approximately 60%) was achieved between chalcopyrite and pyrite using PGA + CaO in a broad pH range (7–12). XPS and FT-IR analysis illustrated a chemisorption mechanism responsible for the PGA adsorption on the surface of pyrite particles when using the mixture of PGA + CaO, leading to the formation of calcium-γ-PGA species () [Citation91].
Figure 6. The molecular structure of PGA and the two-step mechanism in the process of pyrite depression using lime and PGA [Citation91].
![Figure 6. The molecular structure of PGA and the two-step mechanism in the process of pyrite depression using lime and PGA [Citation91].](/cms/asset/1ebad817-8be0-46ad-b373-906f882b10ce/ibty_a_2238885_f0006_c.jpg)
Pyrogallic acid
Pyrogallic acid (PA), with the chemical formula C6H3(OH)3, is an organic water-soluble white powder; however, due to its sensitivity toward oxygen, its color typically changes to brownish. This bio-degradable compound is one of three isomers of trihydroxybenzenes (1,2,3-benzenetriol). PA has been widely utilized as a preservative in the food industry. Also, it is an essential ingredient in the chemicals industry for producing new photosensitive substances [Citation108–110]. Since it shows a solid affinity for metallic sites on the surface of minerals, PA has recently gained attention in the flotation of some ores, such as Ca-containing minerals [Citation111–113]. However, its application as a green inhibitor for pyrite depression has rarely been investigated.
Chen et al. suggested that carboxyl functionalities in PA’s chemical structure were not strong enough to apply sufficient reductive potential for a desorbing collector from a mineral surface [Citation10]. However, the micro-flotation experiments conducted by Han et al. [Citation92] exhibited that PA reduced the pyrite floatability under low-alkalinity conditions, and its impact on the pyrite surface was stronger than that of chalcopyrite, leading to selective separation. Surface analysis measurements indicated that sodium butyl xanthate (NaBX), used as a collector, was negligibly attached to the surface of pyrite particles at an optimum PA concentration at pH 8. IR and zeta potential analyses demonstrated that the chemisorption mechanism was responsible for PA adsorption on the pyrite surface. Also, according to results obtained from XPS analysis, PA molecules are probably adsorbed on the pyrite surface through chemisorption interaction with Fe atoms. This could strengthen the pyrite hydrophilicity and result in its depression. These promising results show that PA can be considered a clean selective depressant for the standard chalcopyrite-pyrite flotation system in low-alkaline environments [Citation92].
Salicylic acid
Salicylic acid (SA) (also known as 2-hydroxybenzoic acid) is a colorless, bitter-tasting organic chemical that is obtained from natural sources, particularly plants. It has been widely employed for treating skin problems due to its bactericidal, antibiotic, and antiseptic features [Citation114,Citation115]. SA is also used for producing cosmetics, such as dermatitis treatment, and clinical medicines, such as aspirin [Citation116,Citation117]. Carboxyl and phenolic hydroxyl groups present in SA’s structure are the functionalities that can interact with unsaturated atoms on the fractured mineral surface by oxygen atoms in the polar functional groups [Citation10,Citation118]. It has been reported that chelating agents with small molecules can be strong pyrite depressants in pyrite-chalcopyrite flotation systems [Citation89]. An SA molecule can quickly bond to a pyrite surface via its functional groups and hinder its collector adsorption. Pyrite particles that adsorbe SA become hydrophilic, facilitating the effective separation process of pyrite from chalcopyrite [Citation93]. Han et al. [Citation89] indicated that the collector adsorption (SBX) onto the surface of pyrite mineral is negligible when using high dosage of SA. Zeta potential and IR analytical measurements showed the simultaneous SA chemical-physical adsorption onto the surface of pyrite particles. XPS and ToF-SIMS analyses showed that active iron atoms on the pyrite surface could be appropriate sites for interaction with SA, and form hydrophilic species. This phenomenon led to increasing hydrophilicity of pyrite particles. SA showed high selectivity, and chalcopyrite, even in high SA dosages, exhibited excellent floatability [Citation93].
Thioglycolic acid
Thioglycolic acid (TGA) is the organic compound containing both thiol and carboxylic acid functional groups. It is a colorless liquid with a strong unpleasant odor. TGA has rarely been reported as an organic reagent in the flotation separation of sulfide minerals. Chen et al. [Citation10] investigated its inhibitory strength on the pyrite particles in a comparative study. The obtained results revealed that organic depressants that have only one functional group, such as hydroxyl (–OH), amino (–NH2), or carboxyl (–COOH), in their structure, cannot effectively depress pyrite. This is why oxalic acid, melocol, and glycerin are not powerful pyrite depressants. However, TGA, which possesses both carboxyl and thiol (–SH) functional groups, can enhance the depression of pyrite particles [Citation10]. Thiol is an intensive reductive functional group. This feature lowers the flotation environment potential as well as the pyrite rest potential, leading to its depression [Citation119].
Humic acids
Sodium humate (NaHu) is the salt of humic acid. Its commercial production can be performed through alkaline extraction of natural substances, such as lignite, weathered coal, peat, etc. Because of its affordability, availability, and eco-friendliness, NaHu can be used for a wide range of applications, such as removing heavy metals from wastewater (as a scavenger), oil drilling (as mud adjuster), and food industry (as additives) [Citation51,Citation94]. The obtained results from laboratory- and industrial-scale experiments indicated that NaHu is an efficient depression reagent for pyrite. It had a strong activity affected by the pulp pH through the flotation separation of pyrite from chalcopyrite. Even though the floatability of chalcopyrite was negligibly reduced in the presence of NaHu, pyrite particles were effectively depressed by NaHu in the alkaline environment. In the presence of ethyl and butyl xanthates as collectors and pH adjusted at 10–10.5, a high-grade chalcopyrite concentrate (24% Cu) was obtained with the addition of NaHu as a depressant (40–60 g/t). Using NaHu reduced CaO consumption, and the recovery of valuable metals (Au, Ag, and Mo) were improved in the froth zone of the flotation cell. IR spectra measurements revealed that NaHu could strongly interact with the pyrite surface through a chemical adsorption mechanism [Citation94].
Various micro-flotation tests revealed that the depression efficiency of NaHu on pyrite particles could be considerably enhanced by Ca-species originating from CaO dissolution. A mixture of CaO and NaHu could selectively inhibit the pyrite floatability with negligible effect on the sphalerite at high alkaline pH levels (11.5–12.0). Under optimum conditions (pH 11.5, 30 g/t NaHu, and 850 g/t CaO), a sphalerite concentrate with around 90% recovery and 46% grade was obtained. Adsorption analyses showed that Ca-containing cations, such as Ca(OH)+ and Ca2+, could considerably improve NaHu adsorption onto the pyrite surface. It was proven that functionalities present in the NaHu structure chemically interacted with adsorbed Ca on the pyrite surface. However, the adsorption of NaHu molecules onto sphalerite was weaker [Citation95]. The same trend has been observed when ammonium humate (NHxHu) as a salt of humic acid has also shown a high selectivity toward pyrite through its separation from chalcopyrite from a plant tailing by using iso-butyl ethionine (Z200) and sodium n-butyl xanthate (SNBX) as collectors. The FTIR and XPS analysis revealed that NHxHu chemically interacted with the pyrite surface, making pyrite particles hydrophilic. Thus, the comprehensive recycling of valuable minerals from the copper tailing was fulfilled successfully [Citation96].
Polypropylene carboxylic acid
Polypropylene carboxylic acid (PCA) is a recently introduced flotation surfactant utilized as a green depression reagent for separating pyrite from chalcopyrite. Micro-flotation test results indicated that, in the presence of a collector (SBX) without any depressant, the recovery of both minerals exceeded 93% in the pH range of 4–10 (no separation). With the addition of 60 mg/L PCA, a considerable decrease in pyrite recovery (from ∼94% to ∼23%) was observed. However, the chalcopyrite floatability was slightly affected (from 96.88% to 90.46%). After optimization of the affecting parameters (15 mg/L SBX, 60 mg/L PCA, pH 8.16), the process could be turned into a selective separation (90.88% for chalcopyrite and 1.29% for pyrite). FTIR, XPS, and Zeta potential measurements also confirmed the selective depressive mechanism of PCA on the two minerals. The obtained outcomes showed that PCA molecules preferentially chemisorbed on the pyrite surface’s iron sites, inhibiting collector adsorption. However, the interaction of PCA molecules with chalcopyrite was negligible, and the collector could attach to the chalcopyrite surface even after pretreatment with PCA [Citation98].
Tannic acid
Tannic acid (TA), with the chemical formula C76H52O46, is a biodegradable tannin-based chemical extracted from different parts of vegetation [Citation120]. The existence of phenols and hydroxyl (–OH) groups attached to a carbon atom in a benzene ring in the TA’s structure () has made it a weakly acidic liquid [Citation121]. TA has been used as a flocculation reagent in purifying wastewater to remove fine particles. Additionally, it is a potential green depressant for metal-bearing minerals [Citation122–124,Citation125]. Investigations on pyrite depression by TA are quite limited. Han et al. [Citation89] investigated pyrite depression by TA in a pyrite-chalcopyrite system at low-alkaline. After using SBX as a collector, the pyrite surface analysis () clearly showed that, at high TA concentration, the collector adsorption was negligible on the pyrite surface. IR and zeta potential analyses suggested both physical and chemical mechanisms simultaneously led to TA adsorption onto the pyrite surface. XPS and ToF-SIMS also indicated that TA interacted with active iron atoms on the pyrite surface. This led to forming many hydrophilic groups on pyrite and its surface deactivation. Flotation experiments confirmed these results and demonstrated that TA had a noticeable inhibitory impact on pyrite floatability. Chalcopyrite, however, invariably revealed efficient floatability regardless of the presence of TA under the same circumstance [Citation97].
Figure 7. Chemical structure of tannic acid containing phenols, hydroxyl groups, and benzene rings [Citation121].
![Figure 7. Chemical structure of tannic acid containing phenols, hydroxyl groups, and benzene rings [Citation121].](/cms/asset/6ff5cfb7-c3b4-4e3e-a947-21f540124d04/ibty_a_2238885_f0007_c.jpg)
Figure 8. TA dosage’s effect on SBX adsorption onto the surface of chalcopyrite and pyrite (pH: 8, SBX: 10 mg/L) [Citation97].
![Figure 8. TA dosage’s effect on SBX adsorption onto the surface of chalcopyrite and pyrite (pH: 8, SBX: 10 mg/L) [Citation97].](/cms/asset/bc742948-83f8-4711-bc97-40d0935faee0/ibty_a_2238885_f0008_c.jpg)
Summary
Pyrite, as the most abundant sulfide mineral, has low economic value, and its unfavorable association with various minerals causes serious challenges in mineral processing processes and environmental pollution. Froth flotation can be employed for pyrite rejection early in the beneficiation procedures. However, the selectivity problem is a main challenge in pyrite-containing flotation systems. Due to the natural hydrophobicity of pyrite particles through flotation separation and its high affinity to xanthates (the most common collectors for flotation of sulfide minerals), satisfying the desired selectivity could be exceptionally difficult without pyrite depression. Using inorganic and toxic depressants (e.g., cyanides, lime, and sulfur-oxy compounds) has been the conventional solution for overcoming this problem. They have been widely employed in flotation despite various practical problems related to: handling, high costs, toxicity, causing poisoning, and several environmental hazards. Therefore, alternative, eco-friendly, and inexpensive organic substances have been recently investigated.
Biodegradable acids are green and eco-friendly pyrite depressants that satisfy environmental requirements for moving toward cleaner and sustainable production. Various hydrophilic functionalities (such as hydroxyl, carboxyl, etc.) have made these chemicals promising for assisting or replacing traditional inorganic pyrite depressants. Also, their depression efficiency can be enhanced by having thiol (–SH) functional groups. Although the effective depression performance of these biocompatible chemicals on pyrite surfaces has been proven, detailed and comprehensive studies on their applications are pretty limited. Moreover, some parameters have not been considered through the conducted investigations, such as the effect of mineral particle size and reaction kinetics. It has been reported that the functional group of these green depressants (LA, TA, PA, and SA) can interact with the iron sites of the pyrite surface through simultaneous chemical and physical adsorption. However, the interaction mechanism of other depressants is still unknown, necessitating more surface measurements, such as zeta potential, XPS, TOF-SIMS, and FTIR, better to understand the interaction between them and various sulfide minerals.
Since pyrite is a semiconductor mineral, its depression using novel reagents can be extremely influenced by parameters, such as the electrochemical potential of the flotation environment, the presence of activating metallic cations (copper and lead), the oxygen content of slurry, etc. However, no research has been conducted in these areas. Additionally, the investigation on the depression behavior and performance of biodegradable acid depressants is limited to a few artificially mixed flotation systems and on the laboratory scale. They are mostly examined for separating pyrite from chalcopyrite at a limited size range (+38–74 µm). Therefore, their ability to remove pyrite from: polymetallic sulfides, complex systems, and other valuable minerals, as well as coal cleaning, both on a laboratory and industrial scale at a wide range (from ultrafine to coarse particles), needs to be investigated. There are some other biodegradable acids, such as gallic acid, citric acid, succinic acid, oxalic acid, and malic acid, of which their effects on pyrite depression can be examined. It should also be noted that these biodegradable acids are more expensive than polysaccharide-based depressants. However, a comparative study and optimization are necessary to comment on their economic superiority. Thus, several rooms are open for working on various aspects of pyrite depression using these green alternative depressants to address existent gaps by conducting further investigations.
Disclosure statement
No potential conflict of interest was reported by the author(s).
Additional information
Funding
References
- Chehreh Chelgani S, Hart B. TOF-SIMS studies of surface chemistry of minerals subjected to flotation separation - a review. Miner Eng. 2014;57:1–11. doi: 10.1016/j.mineng.2013.12.001.
- Mehri A, Haghani M, Mozaffari E. Flotation of potash for carnallite resources in Khur Playa of Iran using Jameson flotation cell. J Environ Anal Chem. 2019;6:2–9.
- Meng J, Tabosa E, Xie W, et al. A review of turbulence measurement techniques for flotation. Miner Eng. 2016;95:79–95. doi: 10.1016/j.mineng.2016.06.007.
- Asimi Neisiani A, Saneie R, Mohammadzadeh A, et al. Biodegradable hematite depressants for green flotation separation – an overview. Miner Eng. 2023;199:108114. doi: 10.1016/j.mineng.2023.108114.
- Chen Y, Li S, Lin S, et al. Promising energy-storage applications by flotation of graphite ores: a review. Chem Eng J. 2023;454:139994. doi: 10.1016/j.cej.2022.139994.
- Choi J, Lee E, Choi SQ, et al. Arsenic removal from contaminated soils for recycling via oil agglomerate flotation. Chem Eng J. 2016;285:207–217. doi: 10.1016/j.cej.2015.09.105.
- Chehreh Chelgani S, Asimi Neisiani A. (2022). Dry mineral processing. Cham: Springer Nature. https://link.springer.com/book/10.1007/978-3-030-93750-8.
- Abraitis PK, Pattrick RAD, Vaughan DJ. Variations in the compositional, textural and electrical properties of natural pyrite: a review. Int J Miner Process. 2004;74:41–59. doi: 10.1016/j.minpro.2003.09.002.
- Attia YA, El-Zeky M. Bioleaching of gold pyrite tailings with adapted bacteria. Hydrometallurgy. 1989;22:291–300. doi: 10.1016/0304-386X(89)90026-1.
- Chen JH, Li YQ, Long QR. Molecular structures and activity of organic depressants for marmatite, jamesonite and pyrite flotation. Trans Nonferrous Met Soc China. 2010;20:1993–1999. doi: 10.1016/S1003-6326(09)60407-6.
- Kydros KA, Matis KA, Papadoyannis IN, et al. Selective separation of arsenopyrite from an auriferous pyrite concentrate by sulphonate flotation. Int J Miner Process. 1993;38:141–151. doi: 10.1016/0301-7516(93)90070-Q.
- Makanza AT, Vermaak MKG, Davidtz JC. The flotation of auriferous pyrite with a mixture of collectors. Int J Miner Process. 2008;86:85–93. doi: 10.1016/j.minpro.2007.11.004.
- Sung YH, Brugger J, Ciobanu CL, et al. Invisible gold in arsenian pyrite and arsenopyrite from a multistage Archaean gold deposit: sunrise Dam, Eastern Goldfields Province, Western Australia. Miner Deposita. 2009;44:793–793. doi: 10.1007/s00126-009-0244-4.
- Bazar JA, Rahimi M, Fathinia S, et al. Talc flotation-an overview. Minerals. 2021;11:662. doi: 10.3390/min11070662.
- Chelgani SC, Rudolph M, Leistner T, et al. A review of rare earth minerals flotation: monazite and xenotime. Int J Mining Sci Technol. 2015;25:877–883. doi: 10.1016/j.ijmst.2015.09.002.
- Feng Q, Yang W, Wen S, et al. Flotation of copper oxide minerals: a review. Int J Mining Sci Technol. 2022;32:1351–1364. doi: 10.1016/j.ijmst.2022.09.011.
- Lu M, Sun Y, Duan H, et al. A working condition recognition method based on multivariable trend analysis for gold-antimony rougher flotation. Miner Eng. 2020;156:106493. doi: 10.1016/j.mineng.2020.106493.
- Mao Y, Xia W, Peng Y, et al. Ultrasonic-assisted flotation of fine coal: a review. Fuel Process Technol. 2019;195:106150. doi: 10.1016/j.fuproc.2019.106150.
- Wang S, Wang Y, Qi T, et al. Effect of redox agents on the reaction behavior of pyrite in sodium aluminate solution at elevated temperatures. Miner Eng. 2023;191:107974. doi: 10.1016/j.mineng.2022.107974.
- Yao W, Tsang M, Wortmann UG. Oxidation of pyrite during barite extraction. Chem Geol. 2022;607:121011. doi: 10.1016/j.chemgeo.2022.121011.
- Evangelou VPB, Zhang YL. A review: pyrite oxidation mechanisms and acid mine drainage prevention. Crit Rev Environ Sci Technol. 1995;25:141–199. doi: 10.1080/10643389509388477.
- Johnson DB, Hallberg KB. Acid mine drainage remediation options: a review. Sci Total Environ. 2005;338:3–14. doi: 10.1016/j.scitotenv.2004.09.002.
- Li Y, Li W, Xiao Q, et al. Acid mine drainage remediation strategies: a review on migration and source controls. Miner Metallur Process. 2018;35:148–158. doi: 10.19150/mmp.8464.
- Chandra AP, Gerson AR. The mechanisms of pyrite oxidation and leaching: a fundamental perspective. In Surface Science Reports. 2010;65:293–315. Issue doi: 10.1016/j.surfrep.2010.08.003.
- Wiersma CL, Rimstidt JD. Rates of reaction of pyrite and marcasite with ferric iron at pH2. Geochim Cosmochim Acta. 1984;48:85–92. doi: 10.1016/0016-7037(84)90351-X.
- Liu R, Yang Z, He Z, et al. Treatment of strongly acidic wastewater with high arsenic concentrations by ferrous sulfide (FeS): inhibitive effects of S(0)-enriched surfaces. Chem Eng J. 2016;304:986–992. doi: 10.1016/j.cej.2016.05.109.
- Mitovski AM, Mihajlovi IN, Štrbac ND, et al. Optimization of the arsenic removal process from enargite based complex copper concentrate. Hem Ind. 2015;69:287–296. doi: 10.2298/HEMIND140203042M.
- Padilla R, Rivas CA, Ruiz MC. Kinetics of pressure dissolution of enargite in sulfate-oxygen media. Metall Mater Trans B. 2008;39:399–407. doi: 10.1007/s11663-008-9151-9.
- Ruiz MC, Vera MV, Padilla R. Hydrometallurgy mechanism of enargite pressure leaching in the presence of pyrite. Hydrometallurgy. 2011;105:290–295. doi: 10.1016/j.hydromet.2010.11.002.
- Seo PW, Ahmed I, Jhung SH. Adsorptive removal of nitrogen-containing compounds from a model fuel using a metal-organic framework having a free carboxylic acid group. Chem Eng J. 2016;299:236–243. doi: 10.1016/j.cej.2016.04.060.
- Tailor R, Sayari A. Grafted propyldiethanolamine for selective removal of SO2 in the presence of CO2. Chemical Engineering Journal. 2016;289:142–149. doi: 10.1016/j.cej.2015.12.084.
- Asimi A, Gharibi K, Abkhoshk E, et al. Effects of operational parameters on the low contaminant jarosite precipitation process-an industrial scale study. Materials. 2020;13:4662. doi: 10.3390/ma13204662.
- Moslemi H, Gharabaghi M. A review on electrochemical behavior of pyrite in the froth flotation process. J Ind Eng Chem. 2017;47:1–18. doi: 10.1016/j.jiec.2016.12.012.
- Hu YH, Zhang SL, Qiu GZ, et al. Surface chemistry of activation of lime-depressed pyrite flotation. Trans Nonferr Metals Soc China. 2000;10:798–803.
- Li Y, Chen J, Kang D, et al. Depression of pyrite in alkaline medium and its subsequent activation by copper. Miner Eng. 2012;26:64–69. doi: 10.1016/j.mineng.2011.11.001.
- He S, Fornasiero D, Skinner W. Correlation between copper-activated pyrite flotation and surface species: effect of pulp oxidation potential. Miner Eng. 2005;18:1208–1213. doi: 10.1016/j.mineng.2005.07.016.
- Pecina E, Uribe A, Nava F, et al. The role of copper and lead in the activation of pyrite in xanthate and non-xanthate systems. Miner Eng. 2006;19:172–179. doi: 10.1016/j.mineng.2005.09.024.
- Chandra AP, Gerson AR. A review of the fundamental studies of the copper activation mechanisms for selective flotation of the sulfide minerals, sphalerite and pyrite. Adv Colloid Interface Sci. 2009;145:97–110. doi: 10.1016/J.CIS.2008.09.001.
- Guo B, Peng Y, Espinosa-Gomez R. Effects of free cyanide and cuprous cyanide on the flotation of gold and silver bearing pyrite. Miner Eng. 2015;71:194–204. doi: 10.1016/j.mineng.2014.11.016.
- Guo B, Peng Y, Parker G. Electrochemical and spectroscopic studies of pyrite–cyanide interactions in relation to the depression of pyrite flotation. Miner Eng. 2016;92:78–85. doi: 10.1016/j.mineng.2016.03.003.
- Hongjun H, Yuehua H, Wei S. Activation flotation and mechanism of lime-depressed pyrite with oxalic acid. Int J Mining Sci Technol. 2012;22:63–67. doi: 10.1016/j.ijmst.2011.07.007.
- Janetski ND, Woodburn SI, Woods R. An electrochemical investigation of pyrite flotation and depression. Int J Miner Process. 1977;4:227–239. doi: 10.1016/0301-7516(77)90004-7.
- Khmeleva TN, Skinner W, Beattie DA, et al. The effect of sulphite on the xanthate-induced flotation of copper-activated pyrite. Physicochem Probl Miner Process. 2002;36:185–195.
- Prestidge CA, Ralston J, Smart RSC. The competitive adsorption of cyanide and ethyl xanthate on pyrite and pyrrhotite surfaces. Int J Miner Process. 1993;38:205–233. doi: 10.1016/0301-7516(93)90076-M.
- Rath RK, Subramanian S, Pradeep T. Surface chemical studies on pyrite in the presence of polysaccharide-based flotation depressants. J Colloid Interface Sci. 2000;229:82–91. doi: 10.1006/jcis.2000.6990.
- Wang XH, Forssberg KSE. The solution electrochemistry of sulfide-xanthate-cyanide systems in sulfide mineral flotation. Miner Eng. 1996;9:527–546. doi: 10.1016/0892-6875(96)00041-6.
- Darabi H, Khodadadi-Darban A, Shahverdi M, et al. Possibility of sodium cyanide elimination from a flotation process. Adv Environ Agric Sci. 2015;:189–193.
- Kyzas GZ, Matis KA. The flotation process can go green. Processes. 2019;7:138. doi: 10.3390/pr7030138.
- Khoso SA, Hu Y, Tian M, et al. Evaluation of green synthetic depressants for sulfide flotation: synthesis, characterization and floatation performance to pyrite and chalcopyrite. Sep Purif Technol. 2021;259:118138. doi: 10.1016/j.seppur.2020.118138.
- Mweene L, Prasad G, Nambaje C. Experimental study on the separation of quartz from pyrite using alginate as a selective depressant substantiated by theoretical analysis on intermolecular bonding. Sep Purif Technol. 2021;276:119251. doi: 10.1016/j.seppur.2021.119251.
- Saim AK, Darteh FK. Eco-friendly and biodegradable depressants in chalcopyrite flotation: a review. Miner Process Extr Metall Rev. 2022:1–19. doi: 10.1080/08827508.2022.2091558.
- Buckley AN, Woods R. The surface oxidation of pyrite. Appl Surf Sci. 1987;27:437–452. doi: 10.1016/0169-4332(87)90153-X.
- Wang XH. Interfacial electrochemistry of pyrite oxidation and flotation: II. FTIR studies of xanthate adsorption on pyrite surfaces in neutral pH solutions. J Colloid Interface Sci. 1995;171:413–428. doi: 10.1006/jcis.1995.1198.
- Acres RG, Harmer SL, Beattie DA. Synchrotron XPS, NEXAFS, and ToF-SIMS studies of solution exposed chalcopyrite and heterogeneous chalcopyrite with pyrite. Miner Eng. 2010;23:928–936. doi: 10.1016/j.mineng.2010.03.007.
- Chander S. Electrochemistry of sulfide flotation: growth characteristics of surface coatings and their properties, with special reference to chalcopyrite and pyrite. Int J Miner Process. 1991;33:121–134. doi: 10.1016/0301-7516(91)90047-M.
- Genn G, Morrison R. Mineral conductivity measurements. Miner Eng. 2014;62:129–132. doi: 10.1016/j.mineng.2014.01.016.
- Riekkola-Vanhanen M. Talvivaara mining company - from a project to a mine. Miner Eng. 2013;48:2–9. doi: 10.1016/j.mineng.2013.04.018.
- Santos LRG, Barbosa AF, Souza AD, et al. Bioleaching of a complex nickel-iron concentrate by mesophile bacteria. Miner Eng. 2006;19:1251–1258. doi: 10.1016/j.mineng.2006.03.001.
- Caldeira CL, Ciminelli VST, Dias A, et al. Pyrite oxidation in alkaline solutions: nature of the product layer. Int J Miner Process. 2003;72:373–386. doi: 10.1016/S0301-7516(03)00112-1.
- Güler T. Dithiophosphinate-pyrite interaction: voltammetry and DRIFT spectroscopy investigations at oxidizing potentials. J Colloid Interface Sci. 2005;288:319–324. doi: 10.1016/j.jcis.2005.03.022.
- Hicyilmaz C, Altun NE, Ekmekci Z, et al. Pyrite-DTPI interaction as a function of pulp potential and pH. Colloids Surf A. 2004a;233:11–24. doi: 10.1016/j.colsurfa.2003.11.004.
- Hicyilmaz C, Altun NE, Ekmekci Z, et al. Quantifying hydrophobicity of pyrite after copper activation and DTPI addition under electrochemically controlled conditions. Miner Eng. 2004b;17:879–890. doi: 10.1016/j.mineng.2004.02.007.
- Kocabag D, Shergold HL, Kelsall GH. Natural oleophilicity/hydrophobicity of sulphide minerals, II. Pyrite. Int J Miner Process. 1990;29:211–219. doi: 10.1016/0301-7516(90)90054-3.
- Monte MBM, Dutra AJB, Albuquerque CRF, et al. The influence of the oxidation state of pyrite and arsenopyrite on the flotation of an auriferous sulphide ore. Miner Eng. 2002;15:1113–1120. doi: 10.1016/S0892-6875(02)00177-2.
- Buswell AM, Bradshaw DJ, Harris PJ, et al. The use of electrochemical measurements in the flotation of a platinum group minerals (PGM) bearing ore. Miner Eng. 2002;15:395–404. doi: 10.1016/S0892-6875(02)00061-4.
- Yuan XM, Pålsson BI, Forssberg KSE. Flotation of a complex sulphide ore I. Cu/Zn selectivity control by adjusting pulp potential with different gases. Int J Miner Process. 1996;46:155–179. doi: 10.1016/0301-7516(95)00094-1.
- Tao DP, Richardson PE, Luttrell GH, et al. Electrochemical studies of pyrite oxidation and reduction using freshly-fractured electrodes and rotating ring-disc electrodes. Electrochim Acta. 2003;48:3615–3623. doi: 10.1016/S0013-4686(03)00482-1.
- Fornasiero D, Ralston J. The interaction of ethyl xanthate with pyrite. Electrochem Miner l Metal Process. 1992;88:191–220.
- Barker GJ, Gerson AR, Menuge JF. The impact of iron sulfide on lead recovery at the giant Navan Zn-Pb orebody, Ireland. Int J Miner Process. 2014;128:16–24. doi: 10.1016/j.minpro.2014.02.001.
- Boulton A, Fornasiero D, Ralston J. Depression of iron sulphide flotation in zinc roughers. Miner Eng. 2001;14:1067–1079. doi: 10.1016/S0892-6875(01)00112-1.
- Dichmann TK, Finch J. The role of copper ions in sphalerite-pyrite flotation selectivity. Miner Eng. 2001;14:217–225. doi: 10.1016/S0892-6875(00)00177-1.
- Ekmekci Z, Demirel H. Effects of galvanic interaction on collectorless flotation behaviour of chalcopyrite and pyrite. Int J Miner Process. 1997;52:31–48. doi: 10.1016/S0301-7516(97)00050-1.
- Owusu C, Brito S, Skinner W, et al. The influence of pyrite content on the flotation of chalcopyrite/pyrite mixtures. Miner Eng. 2014;55:87–95. doi: 10.1016/j.mineng.2013.09.018.
- Zhang Q, Xu Z, Bozkurt V, et al. Pyrite flotation in the presence of metal ions and sphalerite. Int J Miner Process. 1997;52:187–201. doi: 10.1016/S0301-7516(97)00064-1.
- Castell I, Toro N, Edelmira G, et al. Froth flotation of chalcopyrite/pyrite ore : a critical review. Materials. 2022;15:6536. doi: 10.3390/ma15196536.
- Chandra AP, Puskar L, Simpson DJ, et al. Copper and xanthate adsorption onto pyrite surfaces: implications for mineral separation through flotation. Int J Miner Process. 2012;114-117:16–26. doi: 10.1016/j.minpro.2012.08.003.
- Peng Y, Wang B, Gerson A. The effect of electrochemical potential on the activation of pyrite by copper and lead ions during grinding. Int J Miner Process. 2012;102-103:141–149. doi: 10.1016/j.minpro.2011.11.010.
- Finkelstein NP. The activation of sulphide minerals for flotation: a review. Int J Miner Process. 1997;52:81–120. doi: 10.1016/S0301-7516(97)00067-7.
- Leppinen JO. FTIR and flotation investigation of the adsorption of ethyl xanthate on activated and non-activated sulfide minerals. Int J Miner Process. 1990;30:245–263. doi: 10.1016/0301-7516(90)90018-T.
- Huang G, Grano S. Galvanic interaction between grinding media and arsenopyrite and its effect on flotation Part I. Quantifying galvanic interaction during grinding. Int J Miner Process. 2006;78:182–197. doi: 10.1016/j.minpro.2005.10.008.
- Huang G, Grano S, Skinner W. Galvanic interaction between grinding media and arsenopyrite and its effect on flotation: part II. Effect of grinding on flotation. Int J Miner Process. 2006;78:198–213. doi: 10.1016/j.minpro.2005.10.009.
- Peng Y, Grano S, Fornasiero D, et al. Control of grinding conditions in the flotation of chalcopyrite and its separation from pyrite. Int J Miner Process. 2003;69:87–100. doi: 10.1016/S0301-7516(02)00119-9.
- Owusu C, Addai-Mensah J, Fornasiero D, et al. Estimating the electrochemical reactivity of pyrite ores-their impact on pulp chemistry and chalcopyrite flotation behaviour. Adv Powder Technol. 2013;24:801–809. doi: 10.1016/j.apt.2013.05.006.
- Qing You L, Heping L, Li Z. Study of galvanic interactions between pyrite and chalcopyrite in a flowing system: implications for the environment. Environ Geol. 2007;52:11–18. doi: 10.1007/s00254-006-0444-5.
- Adeleke R, Nwangburuka C, Oboirien B. Origins, roles and fate of organic acids in soils: a review. S Afr J Bot. 2017;108:393–406. doi: 10.1016/j.sajb.2016.09.002.
- Cherrington CA, Hinton M, Mead GC, et al. Organic acids: chemistry, antibacterial activity and practical applications. Adv Microb Physiol. 1991;32:87–108. doi: 10.1016/s0065-2911(08)60006-5.
- Gurtler JB, Mai TL. Traditional preservatives-organic acids. In: Batt CA, Tortorello ML, editors. Encyclopedia of food microbiology. 2nd ed. Amsterdam: Elsevier; 2014. p. 119–130. doi: 10.1016/B978-0-12-384730-0.00260-3.
- Mattey M. The production of organic acids. Crit Rev Biotechnol. 1992;12:87–132. doi: 10.3109/07388559209069189.
- Han G, Wen S, Wang H, et al. Lactic acid as selective depressant for flotation separation of chalcopyrite from pyrite and its depression mechanism. J Mol Liq. 2019;296:111774. doi: 10.1016/j.molliq.2019.111774.
- Ahmed S, Hu Y, Lyu F, et al. Selective separation of chalcopyrite from pyrite with a novel non-hazardous biodegradable depressant. J Cleaner Prod. 2019;232:888–897. doi: 10.1016/j.jclepro.2019.06.008.
- Khoso SA, Gao Z, Tian M, et al. The synergistic depression phenomenon of an organic and inorganic reagent on FeS2 in Cu-S flotation scheme. J Mol Liq. 2020;299:112198. doi: 10.1016/j.molliq.2019.112198.
- Han G, Wen S, Wang H, et al. Pyrogallic acid as depressant for flotation separation of pyrite from chalcopyrite under low-alkalinity conditions. Sep Purif Technol. 2021;267:118670. doi: 10.1016/j.seppur.2021.118670.
- Han G, Wen S, Wang H, et al. Selective adsorption mechanism of salicylic acid on pyrite surfaces and its application in flotation separation of chalcopyrite from pyrite. Sep Purif Technol. 2020b;240:116650. doi: 10.1016/j.seppur.2020.116650.
- Chen J, Li Y, Chen Y. Cu-S flotation separation via the combination of sodium humate and lime in a low pH medium. Miner Eng. 2011;24:58–63. doi: 10.1016/j.mineng.2010.09.021.
- Wei Q, Dong L, Jiao F, et al. The synergistic depression of lime and sodium humate on the flotation separation of sphalerite from pyrite. Miner Eng. 2021;163:106779. doi: 10.1016/j.mineng.2021.106779.
- Lv CC, Wang YL, Qian P, et al. Separation of chalcopyrite and pyrite from a copper tailing by ammonium humate. Chin J Chem Eng. 2018;26:1814–1821. doi: 10.1016/j.cjche.2018.02.014.
- Han G, Wen S, Wang H, et al. Interaction mechanism of tannic acid with pyrite surfaces and its response to flotation separation of chalcopyrite from pyrite in a low-alkaline medium. Integr Med Res. 2020a;9:4421–4430. doi: 10.1016/j.jmrt.2020.02.067.
- Wang X, Liu J, Zhu Y, et al. Physicochemical and engineering aspects adsorption and depression mechanism of an eco-friendly depressant PCA onto chalcopyrite and pyrite for the efficiency flotation separation. Colloids Surf A. 2021;620:126574. doi: 10.1016/j.colsurfa.2021.126574.
- Shen WZ, Fornasiero D, Ralston J. Flotation of sphalerite and pyrite in the presence of sodium sulfite. Int J Miner Process. 2001;63:17–28. doi: 10.1016/S0301-7516(00)00067-3.
- Djukić-Vuković A, Mladenović D, Ivanović J, et al. Towards sustainability of lactic acid and poly-lactic acid polymers production. Renewable Sustainable Energy Rev. 2019;108:238–252. doi: 10.1016/j.rser.2019.03.050.
- Feng Q, Zhao W, Wen S. Surface modification of malachite with ethanediamine and its effect on sulfidization flotation. Appl Surf Sci. 2018;436:823–831. doi: 10.1016/j.apsusc.2017.12.113.
- Zhao W, Liu D, Feng Q, et al. DFT insights into the electronic properties and adsorption mechanism of HS − on smithsonite (1 0 1) surface. Miner Eng. 2019;141:105846. doi: 10.1016/j.mineng.2019.105846.
- Feng Q, Wen S, Bai X, et al. Surface modification of smithsonite with ammonia to enhance the formation of sulfidization products and its response to flotation. Miner Eng. 2019;137:1–9. doi: 10.1016/j.mineng.2019.03.021.
- Luo Z, Guo Y, Liu J, et al. Microbial synthesis of poly‑γ‑glutamic acid: current progress, challenges, and future perspectives. Biotechnol Biofuels. 2016;9:134. doi: 10.1186/s13068-016-0537-7.
- Ogawa Y, Yamaguchi F, Yuasa K, et al. Efficient production of γ-polyglutamic acid by Bacillus subtilis (natto) in jar fermenters. Biosci Biotechnol Biochem. 1997;61:1684–1687. doi: 10.1271/bbb.61.1684.
- Zhang C, Wu D, Qiu X. Stimulatory effects of amino acids on γ-polyglutamic acid production by Bacillus subtilis. Sci Rep. 2018;8:17934. – doi: 10.1038/s41598-018-36439-4.
- Ogunleye A, Bhat A, Irorere VU, et al. Poly-γ-glutamic acid: production, properties and applications. Microbiology. 2015;161:1–17. doi: 10.1099/mic.0.081448-0.
- Cilurzo F, Puoci F, Selmin F, et al. Pyrogallic acid-PLGA conjugate as new biodegradable material suitable for final sterilization by irradiation. Polym Adv Technol. 2011;22:2201–2205. doi: 10.1002/pat.1746.
- Singh S, Gaikwad KK, Lee YS. Development and application of a pyrogallic acid-based oxygen scavenging packaging system for shelf life extension of peeled garlic. Sci Hortic. 2019;256:108548. doi: 10.1016/j.scienta.2019.108548.
- Wang H, Pan L, Wu C, et al. Pyrogallic acid coated polypropylene membranes as separators for lithium-ion batteries. J Mater Chem A. 2015;3:20535–20540. doi: 10.1039/C5TA06381G.
- Chen W, Feng Q, Zhang G, et al. Utilization of pyrogallol in flotation separation of scheelite from calcite. Separat Sci Technol. 2021;56:738–745.) doi: 10.1080/01496395.2017.1377249.
- Gao J, Sun W, Hu Y, et al. Propyl gallate: a novel collector for flotation separation of fluorite from calcite. Chem Eng Sci. 2019;193:255–263. doi: 10.1016/j.ces.2018.09.017.
- He J, Hu Y, Sun W, et al. Computational insights into the adsorption mechanism of gallic acid-bearing reagents on calcium-bearing mineral surfaces. Miner Eng. 2020;156:106485. doi: 10.1016/j.mineng.2020.106485.
- Senaratna T, Touchell D, Bunn E, et al. Acetyl salicylic acid (Aspirin) and salicylic acid induce multiple stress tolerance in bean and tomato plants. Plant Growth Regulat. 2000;30:157–161. doi: 10.1023/A:1006386800974.
- Zhang Y, Li X. Salicylic acid: biosynthesis, perception, and contributions to plant immunity. Curr Opin Plant Biol. 2019;50:29–36. doi: 10.1016/J.PBI.2019.02.004.
- Hsieh PW, Aljuffali IA, Fang CL, et al. Hydroquinone-salicylic acid conjugates as novel anti-melasma actives show superior skin targeting compared to the parent drugs. J Dermatol Sci. 2014;76:120–131. doi: 10.1016/J.JDERMSCI.2014.08.013.
- Xu B, Shanmugalingam R, Chau K, et al. The effect of acetyl salicylic acid (Aspirin) on trophoblast-endothelial interaction in vitro. J Reprod Immunol. 2017;124:54–61. doi: 10.1016/J.JRI.2017.10.044.
- Ahmadi M, Gharabaghi M, Abdollahi H. Effects of type and dosages of organic depressants on pyrite floatability in microflotation system. Adv Powder Technol. 2018;29:3155–3162. doi: 10.1016/j.apt.2018.08.015.
- Chen J-H, Feng Q, Lu Y. Electrochemical Mechanism on Interaction of TGA with Sulphide Minerals. Conserv Utili Miner Resour. 2000;6:34–38.
- Zhang Y, Su Y, Peng J, et al. Composite nanofiltration membranes prepared by interfacial polymerization with natural material tannic acid and trimesoyl chloride. J Membr Sci. 2013;429:235–242. doi: 10.1016/j.memsci.2012.11.059.
- Dbira S, Bensalah N, Zagho MM, et al. Oxidative degradation of tannic acid in aqueous solution by UV/S2O82- and UV/H2O2/Fe2+ processes: a comparative study. Appl Sci. 2019;9:156. doi: 10.3390/app9010156.
- Negari MS, Ostad Movahed S, Ahmadpour A. Separation of polyvinylchloride (PVC), polystyrene (PS) and polyethylene terephthalate (PET) granules using various chemical agents by flotation technique. Sep Purif Technol. 2018;194:368–376. doi: 10.1016/j.seppur.2017.11.062.
- Ozcan O, Bulutcu AN. Electrokinetic, infrared and flotation studies of scheelite and calcite with oxine, alkyl oxine, oleoyl sarcosine and quebracho. Int J Miner Process. 1993;39:275–290. doi: 10.1016/0301-7516(93)90020-B.
- Pita F, Castilho A. Separation of plastics by froth flotation. The role of size, shape and density of the particles. Waste Manag. 2017;60:91–99. doi: 10.1016/j.wasman.2016.07.041.
- Zhang C, Wei S, Hu Y, et al. Selective adsorption of tannic acid on calcite and implications for separation of fluorite minerals. J Colloid Interface Sci. 2018;512:55–63. doi: 10.1016/j.jcis.2017.10.043.