ABSTRACT
The presence of a circadian clock in the retinal pigment epithelium (RPE) was discovered recently. However, little is known about mechanisms or processes regulated by the RPE clock. We cultured ARPE-19 monolayers in a transwell culture system, and we found rhythmic mRNA expression of the sodium-potassium-chloride co-transporter SLC12A2. We localized the corresponding protein product, NKCC1, on the apical membrane of ARPE-19 cells. We found that concentrations of sodium, potassium, and chloride oscillated in apical supernatants. The ion concentration gradients between supernatants strongly correlated with SLC12A2 mRNA expression. Our results suggest that the circadian clock regulates ion transport by the RPE via NKCC1 expression.
Introduction
Numerous physiological processes within the mammalian eye show day-night variations, such as: ion channel sensitivity (Ko et al. Citation2001), melatonin synthesis (Tosini and Menaker Citation1996), photoreceptor disc shedding (LaVail Citation1976) and intraocular pressure (Rowland et al. Citation1981). These daily alterations are driven by light and circadian clocks. On a molecular level, these circadian oscillations are generated by interlocking transcriptional and translational feedback loops comprised of core clock genes: Per1-3, Cry1-2, Bmal1, Rev-erbα-β and Clock (Ko and Takahashi Citation2006). Many ocular tissues harbor circadian oscillators including the retinal pigment epithelium (RPE) (Baba et al. Citation2010).
The RPE consists of a monolayer of neuro-epithelial cells that closely communicates with retinal photoreceptors and performs numerous tasks essential for retinal survival (Booij et al. Citation2010; Strauss Citation2005). The RPE forms a tight barrier between the photoreceptors/subretinal space at the apical side and Bruch’s membrane/choriocapillaris at the basolateral side. Across the RPE, active transport of ions, nutrients, and waste products take place. The excitability of photoreceptors depends on subretinal concentrations of K+ which are regulated by coordinated activities of various ion channels and transporters of the RPE (Giblin et al. Citation2016; Wimmers et al. Citation2007). One such co-transporter is NKCC1 which is expressed on the apical membrane of the RPE and mediates the transfer of Na+, K+, and Cl− in a strict ratio across the cellular membrane (Russell Citation2000). The activity of this channel plays an important role in regulating ion concentrations and removal of excess fluid from the subretinal space as reviewed by Crewther (Citation2000); Reichhart and Strauss (Citation2014).
Phagocytosis of photoreceptor outer segments (POS) another process essential for retinal survival is regulated, in part, by the RPE circadian clock (reviewed by Mazzoni et al. Citation2014). However, little is known about other functions and processes that are regulated by the RPE clock. This clock is not affected by the most potent environmental temporal cue (“zeitgeber”) – light (Baba et al. Citation2010; Ikarashi et al. Citation2017) and, thus, relies on signals originating from the retina in order to remain in synchrony with the environment. Bioluminescence studies showed that the RPE clock is entrained by dopamine via DRD2 receptors (Baba et al. Citation2017). Additional in vitro studies have also suggested that the RPE clock is also entrained via histaminergic (Morioka et al. Citation2018) and cholinergic signaling (Ikarashi et al. Citation2017).
In this study, we tested the hypothesis that the circadian clock regulates ion transport by the RPE. We used an in vitro ARPE-19 monolayer model to examine the temporal expression profile of SLC12A2 mRNA. We next analyzed apical and basolateral supernatants from ARPE-19 monolayer cultures at 6 h time-intervals. Using a Spearman’s correlation analysis we found a relationship between SLC12A2 mRNA levels and ion concentration gradients. Our findings suggest that ARPE-19 monolayers provide an interesting tool for studying circadian regulation of physiological processes within the RPE.
Materials and methods
Cell culture
ARPE-19 cells (ATCC, Manassas, VA, USA) were seeded at a density of 1.6 × 105 cells/cm2 on Matrigel (Corning, NY, USA) coated transwell filters (#3460 Costar, Kennebunk, ME, USA) and cultured in Dulbecco’s modified Eagle’s medium DMEM/F12 with L-glutamine, HEPES, 1% fetal calf serum (FCS) and 1% penicillin/streptomycin at 37°C and 5% CO2. In experiments, we used the same batch and passages of cells (maximum of 6 passages after ATCC provided stock) according to ATCC and Molecular Vision guidelines (Boatright et al. Citation2013). The medium was changed 3 times per week with the basolateral compartment of each well filled with 1 ml and apical compartment with 0.5 ml of medium. Confluent monolayer formation was achieved by culturing for 28 days and confirmed using microscopy and measuring the transepithelial electrical resistance of at least 20 Ωcm2. Synchronization was performed with the ‘serum-shock’ procedure using 50% FCS in DMEM/F12 between time-points 0 and 2 h (Balsalobre et al. Citation1998). Cells were harvested at 3 h or 6 h intervals. Matrigel was dissolved from the cells by incubating in Cell Recovery solution (Corning, Bedford, MA, USA) for 30 min at 4°C.
RNA isolation, cDNA synthesis, and mRNA quantification
Total RNA was extracted using the RNeasy mini kit (Qiagen, Valencia, CA, USA) according to the manufacturer’s instruction. Complementary DNA was synthesized from 50 ng of total RNA using oligo(dT) primed reactions with Superscript III reverse transcriptase (Life technologies, Waltham, MA, USA). The synthesized cDNA was amplified with transcript-specific, intron-spanning primers: EF1α forward 5’-AAATAAGCGCCGGCTATGCC-3’; reverse 5’-CAAAGCGACCCAAAGGTGGAT-3’; SLC12A2 forward 5’-GGCAAGACTGCAACTCAACC- 3’; reverse 5’-TCTGCCGGTATGTCTTGGTC- 3’. The PCR amplification cycles were optimized for product quantification. PCR products were electrophorezed on 2% agarose gels containing ethidium-bromide and images were captured using the FujiFilm LAS300 (Tokyo, Japan). Bands were quantified using Aida image analyzer v4.26. Normalization was performed by dividing SLC12A2 band intensities with the values for the EF1α reference gene. Ratios were rescalled by dividing with the average value of SLC12A2/EF1α ratios within an experiment.
Immunocytochemistry and imaging
Cells were fixed with 4% paraformaldehyde for 10 min at room temperature, blocked with 1% BSA, 10% normal donkey serum, 0.2% Triton X-100 in PBS. Incubation with the NKCC1 primary antibody (1:250; Chemicon, AB3560P) was performed for 16h at 4°C. Incubation with Cy3-conjugated donkey anti-rabbit secondary antibody (711–165-152, Jackson ImmunoResearch, USA) was performed for 60 min at room temperature. Samples were mounted with Vectashield antifade medium with DAPI (Vector laboratories, Burlingame, CA, USA). Confocal stacks were acquired with a Leica TSC SP-8 mounted on a Leica DMI6000 inverted microscope using an HC Plan Apochromat x63 objective.
Biochemical analysis
Apical and basolateral supernatants were sampled from ARPE-19 monolayers immediately before cell harvesting. An aliquot of 100 μl was used for measuring ion concentrations (Cobas 8000 ISE900 Chemistry Analyzer, Roche Diagnostics, Rotkreuz, Switzerland) at the Department of Clinical Chemistry, Amsterdam UMC. Sodium, potassium, and chloride were measured according to the manufacturer’s instructions (Roche Diagnostics, Rotkreuz, Switzerland). Concentration gradients were calculated by subtracting the concentrations of measured apical and basolateral supernatants. We weighed tubes of each supernatant to calculate total volumes of each well.
Statistics
Plots were generated using GraphPad Prism software (La Jolla, CA, USA) or SigmaPlot (Systat Software, San Jose, CA, USA). Normality of distribution was confirmed using the Kolmogorov–Smirnov test. Circadian profiles in gene expression or ion concentrations were evaluated by nonlinear least-squares fitting to a sine wave function using SigmaPlot: y = y0 + c ∙ cos [2π (t-ϕ)/τ], where τ represents period, ϕ acrophase and c amplitude. The function featured the following constraints: 20 h < τ < 28 h; ϕ < τ and c > 0. The effect of time and supernatant compartment on ion concentrations/fluid weights was assessed using a two-way ANOVA. We used the non-parametric Spearman’s ρ test to assess the relationship between gene expression and ion gradients or fluid difference. A cross-correlation matrix was performed to assess the relationship between ion gradients and between ion gradients and fluid volume differences. Power calculations of performed statistical tests are provided in the Supplementary file.
Results
The SLC12A2 co-transporter was found to be expressed in ARPE-19 cells cultured as either dispersed cells or monolayers (). The SLC12A2 amplicon was also present in the control sample cDNA (brain, retina, and placenta) and confirmed using Sanger sequencing (data not shown). In ARPE-19 monolayers the protein product NKCC1 was expressed on the apical membrane () similar to the in vivo RPE situation (Bialek et al. Citation1995). We synchronized ARPE-19 cells using the “serum-shock” procedure (see methods) and harvested them every 3 h for 54 h. By using RT-PCR analysis, we found that ARPE-19 monolayers showed rhythmic mRNA expression of SLC12A2 (; non-linear regression, p < .05). SLC12A2 mRNA oscillated with an amplitude of 56.17 ± 16.43%, acrophase 6.48 ± 2.39 h and period of 28 ± 2.40 h, thus indicating that the expression of this transporter is under circadian control.
Figure 1. The circadian clock likely regulates ion transport by the ARPE-19 monolayers via NKCC1. a. Both ARPE-19 monolayers (ARPE-19M) and dispersed cell cultures (ARPE-19CC) expressed SLC12A2 mRNA. Amplification products were confirmed by sequencing. b. Confocal microscopy revealed that NKCC1 localized on the apical membrane of ARPE-19 monolayers (white arrows). c. The mRNA expression of SLC12A2 was rhythmic in ARPE-19 monolayers as determined by RT-PCR analysis. d. In RPE monolayers, two-way ANOVA revealed that apical supernatants had higher Na+, K+ and Cl− concentrations than basolateral supernatants. Time significantly affected K+ concentrations and tended to affect Na+. e. Spearman’s correlation analysis of the expression of SLC12A2 mRNA and the calculated inter-compartmental ion concentration gradients or fluid difference (Api – BL). Values are plotted c, e. or represented as means ± SEM d. n = 3.
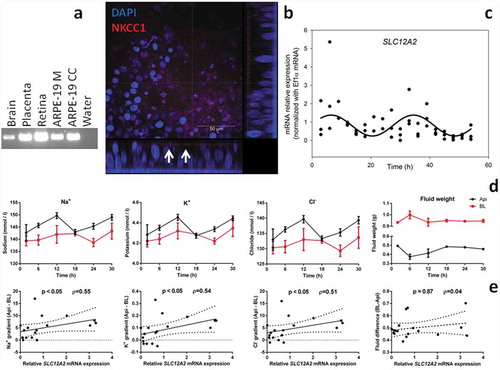
To functionally test the hypothesis that the circadian clock regulates ion transfer by the RPE, we sampled supernatants from synchronized ARPE-19 monolayers (apart from cell lysates for RT-PCR) at 6 h intervals and performed biochemical analysis (). Considering the fact that NKCC1 also regulates the volume of subretinal fluid via ion transport (Russell Citation2000), we also weighed the tubes containing the supernatants. Two-way ANOVA analysis revealed that apical supernatants had higher concentrations of Na+ (p < .001), K+ (p < .01) and Cl− (p < .01) than basolateral supernatants (). As expected based on initial compartment volume, basolateral supernatants were heavier than apical ones (p < .0001). Time-affected concentrations of K+ (p < .05) tended to affect Na+ (p = .10), but not Cl− (p = .15) or supernatant fluid weight (p = .35). Time x compartment was significant for fluid weight (p < .01), but not Na+ (p = .59), K+ (p = .75) and Cl− (p = .71) concentrations. We found that ion concentrations and fluid volumes displayed circadian variations in apical supernatants, but not basolateral ones (). The lack of rhythmicity in basolateral supernatants is likely due to the disproportionally higher volume of these compartments relative to apical ones. Thus, changes in supernatant compositions are more likely to be detected in apical ones. Taken together these results suggest that the transport of ions and fluid by ARPE-19 monolayers are likely regulated by the circadian clock.
Table 1. Statistical analysis of rhythmicity in ARPE-19 supernatant components. Units for ion amplitudes are in mmol/l, for fluid amplitudes in ml. Data were fitted to the equation y = y0 + c⋅cos[2π⋅(t-ϕ)/τ] by non-linear regression. Bold values represent p < .05.
To test whether the transport of ions could be mediated via NKCC1, we first calculated the concentration gradients for each ion by subtracting concentrations of basolateral from apical supernatants (data not graphed). We then performed correlation analyses in order to confirm the relationship between SLC12A2 mRNA and ion transport (). Spearman’s ρ testing showed that SLC12A2 mRNA expression levels correlated with concentration gradients of Na+ (ρ = 0.55; p < .05), K+ (ρ = 0.54; p < .01) and Cl− (ρ = 0.51; p < .01), but not fluid difference (ρ = 0.04; p = .87). NKCC1 transports ions in strictly defined ratios causing a significant flow of water across the cellular membrane (Russell Citation2000). To determine whether there is a relationship between the concentrations of each ion and water transport, we performed a cross-correlation analysis (analysis not graphed). We found that the concentration gradients between compartments correlated significantly (Na+ vs. K+, p < .001, R = 0.98; Na+ vs. Cl−, p < .001, R = 0.99; K+ vs. Cl−, p < .001, R = 0.98). In addition, ion concentration gradients correlated with inter-compartmental (BL-Api) volume differences (Na+ vs. Vol, p < .05, R = 0.51; K+ vs. Vol, p < .05, R = 0.50; Cl− vs. Vol, p < .05, R = 0.54). Therefore, it is likely that, in our cellular model, the observed inter-compartmental ion concentration differences are caused, at least in part, by NKCC1.
Discussion
Photoreceptors depolarize during darkness, which results in continuous release of K+ into the subretinal space. Photons interact with photoreceptors leading to hyperpolarization of these cells and alterations in the activity of photoreceptor ion pumps (Wimmers et al. Citation2007). Consequently, upon illumination, potassium concentration in the subretinal space rapidly decreases from 5 mM to 2 mM (Reichhart and Strauss Citation2014). This decrease in subretinal K+ hyperpolarizes the apical membrane of the RPE thus inhibiting the NKCC1-mediated absorption of Na+, K+ and Cl− (Crewther Citation2000). Furthermore, it was proposed that the flux of ions through NKCC1 reverses upon light onset in order to compensate for the light-induced decrease in subretinal K+ (Reichhart and Strauss Citation2014). Considering that the RPE encompasses a circadian clock (Baba et al. Citation2010), and given our results, we speculate that the RPE circadian clock regulates SLC12A2 mRNA transcription in anticipation of changes in subretinal K+ concentration. In this study, we report that, similar to RPE in vivo (Bialek et al. Citation1995), the ARPE-19 cell line, cultured as a monolayer, expressed the SLC12A2 protein product NKCC1 on the apical membrane. Analysis of supernatant ion concentrations revealed that apical RPE supernatants showed rhythmic changes in Na+, K+ and Cl− concentrations. We found that the mRNA expression of SLC12A2 was rhythmic and correlated with ion concentration gradients suggesting that their translocation is mediated via the circadian regulation of NKCC1.
The ARPE-19 cell line encompasses several key advantages in studying RPE physiological processes such as a normal karyotype, fast proliferation, capability of forming tight junctions, and acquiring a “honey-comb”-like epithelial appearance (Lehmann et al. Citation2014). However, this cell line has also a number of limitations: an altered gene expression pattern relative to native RPE for some pathways (Strunnikova et al. Citation2010), deficient ion channel expression (such as Bestrophin-1) and “flipped” polarity of certain ion channels (Lehmann et al. Citation2014). Despite these deficiencies, ARPE-19 cells have been used successfully in studying ion channels such as the L-type calcium channel Cav 1.3 (Reichhart et al. Citation2015b), calcium-dependent chloride channels (Keckeis et al. Citation2017) and TRPV2 (Reichhart et al. Citation2015a). In addition, we report that ARPE-19 adequately polarized the expression of the NKCC1 channel (), thus suggesting that this cell line holds potential in studying ion channel physiology.
The correlation between SLC12A2 mRNA and the inter-compartmental concentration gradients of Na+, K+ and Cl− could be explained in several ways: (I) NKCC1 and ion concentration gradients might be both modulated by the circadian clock where the ion concentration gradients are established by yet unidentified transporter(s). (II) The transport of these ions might be mediated by the clock-regulated NKCC1 cotransporter. Results from our study support explanation (II). A unique characteristic of the NKCC1 cotransporter is that all three ions have to be simultaneously present on the same side of the membrane in order for ion translocation to occur (Russell Citation2000). The remarkably high cross-correlation of Na+, K+ and Cl− concentration gradients observed in our study, coupled with the highly similar acrophases in ion concentrations in apical compartments () suggests that all of these ions are translocated simultaneously through the apical membrane, a process which is, thus, likely mediated via NKCC1. Although the regulation of ion concentrations by the RPE is mediated by a plethora of various ion channels (Wimmers et al. Citation2007), results from our ARPE-19 cell culture suggest that NKCC1 regulates subretinal ion concentrations in a rhythmic manner.
Previous work revealed that the RPE possesses a circadian oscillator independent of the central clock located in the suprachiasmatic nucleus in the brain (Baba et al. Citation2010). Emerging evidence suggests that this independent clock regulates ion transport by the RPE. Namely, day and night differences were observed in the mRNA expression of the L-type calcium channel Cav 1.3 (Muller et al. Citation2014) as well as the reorganization of voltage-gated sodium channels Navs (Johansson et al. Citation2017). Our data suggest that the transcription of SLC12A2 co-transporter is under circadian control. This gene also showed rhythmic day-night expression in baboon tissues: putamen, testicles, visual cortex, abdominal muscles and lateral globus pallidus (Mure et al. Citation2018) as well as in rat colon (Sotak et al. Citation2011), but this is the first report of rhythmic mRNA expression of SLC12A2 in the human RPE.
Recent work showed that the retinal and RPE clocks are affected by aging (Baba and Tosini Citation2018). In addition to lower PER2 bioluminescence amplitudes, aged retinas and RPE had significantly different acrophases, suggesting that these clocks decouple during aging (Baba and Tosini Citation2018). It is, therefore, reasonable to speculate that the decoupling of the RPE and retinal clocks disrupts clock-regulated processes such as regulation of ion composition of the subretinal space (Ko et al. Citation2009). We speculate that this mechanism might underlie the reduced retinal sensitivity observed in aged clock-disrupted Bmal1 mutant mice (Baba et al. Citation2018). Whether aging disrupts the clock-regulated activity and/or expression of NKCC1 in the RPE remains an open question.
In conclusion, these results suggest that the circadian clock is involved in regulating RPE-mediated ion transport, at least in part via rhythmic transcription of the SLC12A2 cotransporter.
Declaration of interest statement
The authors have no conflicts of interest to disclose.
Supplemental Material
Download MS Excel (13 KB)Acknowledgments
This research was supported by the NeuroTime Erasmus+ program of the European Commission, RSB Rotterdam, Nelly Reef foundation, Foundation Ooglijders, and Het Lot SVLB. We extend our gratitude to Cristina Sandu, INCI, University of Strasbourg and Femke Schrauwen, Trialcoordinator LAKC, Laboratory of Clinical Chemistry, Amsterdam UMC for technical assistance.
Additional information
Funding
References
- Baba K, DeBruyne JP, Tosini G. 2017. Dopamine 2 receptor activation entrains circadian clocks in mouse retinal pigment epithelium. Sci Rep. 7:5103.
- Baba K, Piano I, Lyuboslavsky P, Chrenek MA, Sellers JT, Zhang S, Gargini C, He L, Tosini G, Iuvone PM (2018). Removal of clock gene Bmal1 from the retina affects retinal development and accelerates cone photoreceptor degeneration during aging. Proc Natl Acad Sci USA. 115:13099–13104. doi:10.1073/pnas.1808137115.
- Baba K, Sengupta A, Tosini M, Contreras-Alcantara S, Tosini G. 2010. Circadian regulation of the PERIOD 2:: LUCIFERASEbioluminescence rhythm in the mouse retinal pigment epithelium-choroid. Mol Vis. 16:2605–2611.
- Baba K, Tosini G. 2018. Aging alters circadian rhythms in the mouse eye. J Biol Rhythms. 33:441–445.
- Balsalobre A, Damiola F, Schibler U. 1998. A serum shock induces circadian gene expression in mammalian tissue culture cells. Cell. 93:929–937.
- Bialek S, Joseph DP, Miller SS. 1995. The delayed basolateral membrane hyperpolarization of the bovine retinal pigment epithelium: mechanism of generation. J Physiol. 484(Pt 1):53–67.
- Boatright JH, Cammarata PR, Nickerson JM, Zhang Q. 2013. On authentication of cell lines. Mol Vis. 19:1848–1851.
- Booij JC, Baas DC, Beisekeeva J, Gorgels TG, Bergen AA. 2010. The dynamic nature of Bruch’s membrane. Prog Retin Eye Res. 29:1–18.
- Crewther DP. 2000. The role of photoreceptors in the control of refractive state. Prog Retin Eye Res. 19:421–457.
- Giblin JP, Comes N, Strauss O, Gasull X. 2016. Ion Channels in the Eye: Involvement in Ocular Pathologies. Adv Protein Chem Struct Biol. 104:157–231.
- Ikarashi R, Akechi H, Kanda Y, Ahmad A, Takeuchi K, Morioka E, Sugiyama T, Ebisawa T, Ikeda M, Ikeda M. 2017. Regulation of molecular clock oscillations and phagocytic activity via muscarinic Ca(2+) signaling in human retinal pigment epithelial cells. Sci Rep. 7:44175.
- Johansson JK, Ihalainen TO, Skottman H, Nymark S. 2017. Fast voltage sensitivity in retinal pigment epithelium: sodium channels and their novel role in phagocytosis. bioRxiv. 223719. doi:10.1101/223719
- Keckeis S, Reichhart N, Roubeix C, Strauss O. 2017. Anoctamin2 (TMEM16B) forms the Ca(2+)-activated Cl(-) channel in the retinal pigment epithelium. Exp Eye Res. 154:139–150.
- Ko CH, Takahashi JS. 2006. Molecular components of the mammalian circadian clock. Hum Mol Genet. 15(Spec No 2):R271–277.
- Ko GY, Ko ML, Dryer SE. 2001. Circadian regulation of cGMP-gated cationic channels of chick retinal cones. Erk MAP Kinase and Ca2+/calmodulin-dependent protein kinase II. Neuron. 29:255–266.
- Ko GY, Shi L, Ko ML. 2009. Circadian regulation of ion channels and their functions. J Neurochem. 110:1150–1169.
- LaVail MM. 1976. Rod outer segment disk shedding in rat retina: relationship to cyclic lighting. Science. 194:1071–1074.
- Lehmann GL, Benedicto I, Philp NJ, Rodriguez-Boulan E. 2014. Plasma membrane protein polarity and trafficking in RPE cells: past, present and future. Exp Eye Res. 126:5–15.
- Mazzoni F, Safa H, Finnemann SC. 2014. Understanding photoreceptor outer segment phagocytosis: use and utility of RPE cells in culture. Exp Eye Res. 126:51–60.
- Morioka E, Kanda Y, Koizumi H, Miyamoto T, Ikeda M. 2018. Histamine Regulates Molecular Clock Oscillations in Human Retinal Pigment Epithelial Cells via H1 Receptors. Front Endocrinol (Lausanne). 9:108.
- Muller C, Mas Gomez N, Ruth P, Strauss O. 2014. CaV1.3 L-type channels, maxiK Ca(2+)-dependent K(+) channels and bestrophin-1 regulate rhythmic photoreceptor outer segment phagocytosis by retinal pigment epithelial cells. Cell Signal. 26:968–978.
- Mure LS, Le HD, Benegiamo G, Chang MW, Rios L, Jillani N, Ngotho M, Kariuki T, Dkhissi-Benyahya O, Cooper HM, et al. 2018. Diurnal transcriptome atlas of a primate across major neural and peripheral tissues. Science. 359(6381):p.eaao0318.
- Reichhart N, Keckeis S, Fried F, Fels G, Strauss O. 2015a. Regulation of surface expression of TRPV2 channels in the retinal pigment epithelium. Graefes Arch Clin Exp Ophthalmol. 253:865–874.
- Reichhart N, Markowski M, Ishiyama S, Wagner A, Crespo-Garcia S, Schorb T, Ramalho JS, Milenkovic VM, Fockler R, Seabra MC, et al. 2015b. Rab27a GTPase modulates L-type Ca2+ channel function via interaction with the II-III linker of CaV1.3 subunit. Cell Signal. 27:2231–2240.
- Reichhart N, Strauss O. 2014. Ion channels and transporters of the retinal pigment epithelium. Exp Eye Res. 126:27–37.
- Rowland JM, Potter DE, Reiter RJ. 1981. Circadian rhythm in intraocular pressure: a rabbit model. Curr Eye Res. 1:169–173.
- Russell JM. 2000. Sodium-potassium-chloride cotransport. Physiol Rev. 80:211–276.
- Sotak M, Polidarova L, Musilkova J, Hock M, Sumova A, Pacha J. 2011. Circadian regulation of electrolyte absorption in the rat colon. Am J Physiol Gastrointest Liver Physiol. 301:G1066–1074.
- Strauss O. 2005. The retinal pigment epithelium in visual function. Physiol Rev. 85:845–881.
- Strunnikova NV, Maminishkis A, Barb JJ, Wang F, Zhi C, Sergeev Y, Chen W, Edwards AO, Stambolian D, Abecasis G, et al. 2010. Transcriptome analysis and molecular signature of human retinal pigment epithelium. Hum Mol Genet. 19:2468–2486.
- Tosini G, Menaker M. 1996. Circadian rhythms in cultured mammalian retina. Science. 272:419–421.
- Wimmers S, Karl MO, Strauss O. 2007. Ion channels in the RPE. Prog Retin Eye Res. 26:263–301.