Abstract
Piehler, M.F., J. Dyble, P.H. Moisander, A.D. Chapman, J. Hendrickson and H.W. Paerl. 2009. Interactions between nitrogen dynamics and the phytoplankton community in Lake George, Florida, USA. Lake Reserv. Manage. 25:1–14.
Nutrient addition bioassays were conducted to examine the relationship between inorganic nutrient enrichment and phytoplankton community structure and function in Lake George in the St. Johns River System, Florida, USA. Additionally, a nitrogen budget for the lake was developed and included data from the period of this study. We identified the factors that affected cyanobacterial productivity, prevalence, and nitrogen (N2) fixation. The importance of N2 fixation to the nitrogen (N) budget of the system was also assessed. We hypothesized that N2 fixation significantly contributed to the Lake George N budget and that changing the nutrient conditions in manipulative experiments would affect rates of N2 fixation and composition of the phytoplankton, particularly the N2-fixing community. Phytoplankton primary productivity in Lake George was stimulated by the addition of both N and phosphorus (P). Phytoplankton biomass accumulation was most often enhanced by the combined addition of N and P; however, N alone was also often stimulatory. When detected, N2 fixation was always stimulated by P additions. Short-term changes in phytoplankton community composition included taxonomic shifts in response to nutrient manipulations. Fixation of N2 appeared to be a significant contributor to the N load to the lake. Human impacts that change the loading of N, P, or both N and P to Lake George may affect phytoplankton community structure (composition and biomass) and function (primary productivity and N2 fixation). These changes could have consequences for biogeochemical cycling in Lake George and potentially through the freshwater-marine continuum. Continuing nutrient management efforts in this and other similar systems must account for the activity of N2-fixing cyanobacteria, as products (carbon fixation) and drivers (sources of new nitrogen) of eutrophication.
Human activities throughout the landscape can modify nutrient conditions in aquatic ecosystems, which in turn have been implicated in the expansion of cyanobacterial abundance and bloom formation (CitationPaerl 1998; CitationCanfield et al. 1989, CitationSmith 1990). Diversion and impoundment of rivers, streams, ponds, and lakes for water storage have exacerbated the problem by reducing base flow in some affected waters. Conversely, ditching and destruction of wetlands have decreased transport time of storm pulses through the land-water interface (CitationVitousek et al. 1997), thus reducing the opportunities for nutrient removal. Increased nutrient delivery from watersheds often causes cascading effects through the freshwater-marine continuum (CitationPaerl et al. 2004). Determining the impacts of changing nutrient regimes on phytoplankton community structure and function as well as the potential impacts of the activity of phytoplankton on aquatic system's nutrient regime is critical to effective management. Many of the processes that control nutrient limitation in these systems are biologically mediated (CitationHarris 1999) and thus not easily forecast, particularly in the context of a human-dominated environment (CitationPalmer et al. 2004).
Florida's largest river system, the St. Johns River System (SJRS), is experiencing excessive nutrient loading from anthropogenic sources (CitationPhlips et al. 2000). These sources include the typical results of intensive human development: domestic wastewater, industrial wastewater, stormwater, and agricultural sources of nutrients. Human expansion in this region of Florida is projected to continue at a fast pace, with the population in the region surrounding the SJRS expected to grow from 3.5 million (as of 2000) to 5 million by 2020 (CitationVergara 2000). There have been significant efforts to maintain and improve the water quality of the SJRS, including restoring wetlands and improving management to reduce the sources of nutrients. Despite these efforts, the SJRS contains more water bodies with declining rather than improving water quality (CitationWinkler and Ceric 2004).
This study focused on Lake George, the second largest freshwater lake in Florida, situated upstream of the St. Johns River Estuary (). Studies of nutrient limitation of phytoplankton growth in lakes and estuaries have generally found lakes to be phosphorus (P) limited and estuaries to be nitrogen (N) limited (CitationSchindler 1977; CitationHowarth 1988; CitationNational Research Council 2000). Historically, the St. Johns system has had extensive blooms of N2-fixing cyanobacteria, suggesting at least periodic N limitation. Because they can support their own N needs, N2-fixing cyanobacteria often enjoy a competitive advantage in waters where the ratio of N to P is low (CitationFlett et al. 1980, CitationSmith 1983, Citation1990, CitationSmith and Bennett 1999). Cyanobacterial nutritional flexibility could allow them to increase in prevalence in systems employing nutrient management plans that lower the ratio of N to P and to potentially provide a significant contribution to the overall system's N budget (CitationPiehler et al. 2002).
Figure 1 Location of Lake George and the St. Johns River within northeastern Florida. The river flows north and enters the Atlantic Ocean near the Florida-Georgia border.
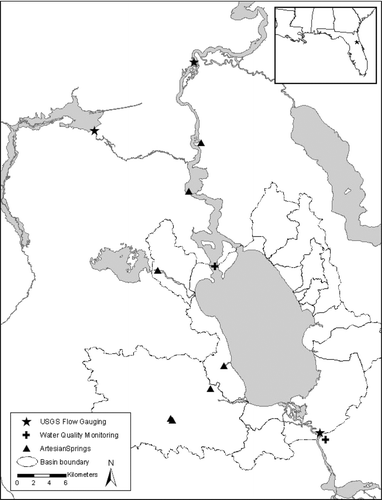
Using nutrient addition bioassays, we examined some of the environmental factors controlling phytoplankton community composition, primary production, N2 fixation, and biomass accumulation in Lake George. Our goals were to determine the effects of increased nutrient supplies on phytoplankton community structure at the group and species levels, detail the changes in prevalence and activity (N2 fixation) of N2-fixing cyanobacteria resulting from changes in nutrient supplies, and estimate the relative importance of biologically fixed N to the external nutrient supply of Lake George.
Methods
Site description
The St. Johns system is a lacustrine river system that drains the Atlantic coastal plain of northeast Florida. Lake George is 210 km from the river mouth and acts as the head of tide for the St. Johns River (), dampening tidal oscillation and upstream flow surges and establishing the point where the river becomes predominantly lacustrine. The lake has an 18,934 ha surface area and a 3.0 m mean depth. While there is no encroachment of oceanic salinity, the lake exhibits mild (median = 0.52 psu; 90th percentile = 0.77 psu) salinity associated with brackish artesian springs and connate water upwelling. As a result, it is inhabited by a variety of fresh and marine organisms (St Johns River Water Management District, unpublished data).
The dominant flow into the lake is the upper St. Johns River, on average accounting for 78.9 % of the lake volume, with direct precipitation, artesian spring flow, and local watershed runoff accounting for 8.7, 8.3, and 4.1% of the lake volume input (). Average annual volume replacement rate from 1996 through 2005 was 6.2 times. Lake residence time is greatest in spring and early summer (April–July) and is coincident with the timing of maximum phytoplankton productivity. Patterns of La Niña and El Niño strongly influence lake volume turnover, with El Niño years exhibiting the greatest turnover in winter, while La Niña years exhibit the greatest volume turnover during the summer tropical and convective thunder storm season (E. Phlips, Univ. Florida, pers. comm.). La Niña years typically exhibit dense cyanobacterial blooms early in the year that reach exogenous nitrogen limitation, leading to phytoplankton community dominance by N-fixing cyanobacteria.
Table 1 Ambient nutrient concentrations and ratios at Lake George (LG) during bioassays. Nutrient concentrations are μ M. Samples that were below the detection limit are indicated by BD. The limits of detection were 0.26 μ M for NO3 − + NO2 −, 0.31 μ M for NH4 + and 0.02 μ M for PO4 −3.
Table 2 Flow rate and nitrogen and phosphorus form concentrations for major inputs to Lake George, 1996–2005.
In situ bioassay protocol
Water was collected from our sampling site in Lake George in HCl-rinsed 20-L carboys (). Samples were taken from just below the surface and transported to the St. Johns River Water Management District (SJRWMD) field lab at Green Cove Springs, Florida. Treatments included the addition of 20 μ M inorganic N as NO3 − (+N), 5 μ M inorganic P as PO4 −3 (+P), and a combination of NO3 − and PO4 −3 (+NP). Nutrient additions were made once at the beginning of the experiment. Each treatment had five replicates of 5 L of water contained in 10-L Cubitainers (Hedwin Corp., Baltimore, MD) made of chemically inert polyethylene that transmits 85% of incident irradiance (CitationPaerl 1987). Cubitainers were placed in holding frames in the St. Johns River to maintain in situ temperature and irradiance conditions, and incubated for 96 h. Cubitainers were shaken daily to prevent settling of biomass and covered with one layer of neutral density nylon screening to prevent photoinhibition. Dissolved inorganic carbon (DIC) was replenished daily (1 mM addition) to prevent depletion. Subsamples were taken from each cubitainer after 96 h and analyzed for phytoplankton biomass as chlorophyll a (Chl a), primary productivity (14CO2 fixation), nitrogenase activity (acetylene reduction), phytoplankton group composition (HPLC diagnostic photopigment analysis), and species-specific responses (microscopic cell counts). Community responses were analyzed using a one-way analysis of variance (ANOVA). Data were natural log transformed when necessary to satisfy the normality assumption. Post-hoc comparisons of means were made using a Bonferroni multiple comparisons of means (CitationMoore and McCabe 1993). Data presented here are from bioassays performed in July 2000, October 2000, February 2001 and April 2001.
Phytoplankton biomass
Chlorophyll a concentration was measured using a nonacidification fluorometric method (CitationWelschmeyer 1994). A Turner (Sunnyvale, CA) TD-700 fluorometer was used, which incorporated a set of very narrow bandpass excitation and emission filters that nearly eliminated spectral interference caused by the presence of phaeophytin a and chlorophyll b. Subsamples of 50 ml were filtered within 0.5 h of collection onto 25 mm Whatman GF/F glass microfiber filters (Fisher Scientific, Pittsburgh, PA) and stored at −20°C for fewer than 14 days prior to analysis. A dose of 10 ml acetone (90% acetone: 10% deionized water) was added to filters in polypropylene centrifuge tubes. Filters were then sonicated on ice for 30 s and extracted for approximately 18 h at −20°C.
Primary productivity
Phytoplankton primary productivity was measured using the 14C method (CitationPaerl 1987). Samples were taken from each Cubitainer and dispensed into 20-ml clear borosilicate vials for incubation. Radio-labeled sodium bicarbonate (NaH14CO3) was added to samples (∼ 6 kBq ml−1 final activity) that were then incubated in a rack at the water surface. One layer of neutral density screening was used to reduce incident irradiance and prevent photoinhibition. Following 4 h incubations, samples were filtered onto 25 mm Whatman GF/F filters, fumed with concentrated HCl to remove unincorporated 14C, and air-dried. Dry filters were placed in 7-ml plastic scintillation vials with 5 ml Ecolume scintillation cocktail (ICN, Inc.). Radioactivity was quantified using a Beckman (Fullerton, CA) model LS5000TD liquid scintillation counter. Counts per minute (CPM) were converted to disintegrations per minute (DPM) using quench curves derived with a calibrated 14C-toluene standard. The DIC in water samples was determined using a Shimadzu (Kyoto, Japan) total organic carbon (TOC) analyzer.
Nitrogenase activity
Fixation of N2 (nitrogenase activity) was estimated using the acetylene reduction assay (CitationStewart et al. 1967). Sample water aliquots of 50 ml were added to 72 ml serum vials that were then capped with flanged red rubber stoppers. Acetylene was generated by adding calcium carbide (CaC2) to deionized water (DI) and injected into the headspace of the vial (∼ 20% of the total volume). Two types of blanks were run with each incubation. Blanks with acetylene and deionized water were run to account for abiotic generation of ethylene. Sample blanks consisted of serum vials with sample water but no acetylene and accounted for generation of ethylene from sources other than reduction of acetylene. Serum vials were incubated in situ in a rack at the water surface near Shands Pier in the St Johns River for 4 h under one layer of neutral density screening. Following the incubation, 5 ml headspace subsamples were transferred to evacuated 4-ml Vacutainers that were used to transport the gas samples. Samples were stored at room temperature in the Vacutainers for no longer than 72 h. Subsamples from the Vacutainers were transferred to 2-ml evacuated autosampler vials for analysis of ethylene using a Shimadzu GC 9A gas chromatograph equipped with a flame ionization detector (FID). The GC was fitted with a 2-m stainless steel Poropak T (Supelco, Bellefonte, PA) column held at 80°C with as high purity nitrogen as the carrier gas. Rates were expressed in terms of ethylene generated per unit time and volume.
Diagnostic photopigment analysis
Phytoplankton community composition was determined using diagnostic photopigment analyses (CitationMillie et al. 1993; CitationTester et al. 1995; CitationJeffery et al. 1997). Water samples were collected from Cubitainers at specified time intervals, filtered onto 25-mm Whatman GF/F filters, and frozen (−80°C). Photopigments were extracted in 90% aqueous acetone, using sonication. High performance liquid chromatography (HPLC) was used to separate and quantify diagnostic photopigments (chlorophylls and carotenoids) for major algal groups (cyanobacteria, diatoms, chlorophytes, cryptophytes, and dinoflagellates). An in-line photodiode array spectrophotometer (PDAS, Shimadzu SPD M10av) provided identification and quantification of individual photopigments based on characteristic absorption spectra (380–700 nm; Rowan 1989). Pigment concentrations from HPLC were analyzed using CHEMTAX to calculate the relative abundances of the major phytoplankton groups (CitationMackey et al. 1997). CHEMTAX is a matrix factorization program for calculating abundances of algal groups from concentrations of chemosystematic marker photopigments (chlorophylls and carotenoids; CitationMackey et al. 1996; CitationMackey et al. 1997; CitationWright et al. 1996; CitationPinckney et al. 1998). The resulting group composition matrix was expressed as a relative proportion of the total chlorophyll a.
Microscopy
Samples for microscopic enumeration were preserved in a 1% Lugol's solution and stored in 125-ml amber polyethylene bottles at the conclusion of the experiment. A cleaned Utermöhl counting chamber was constructed for each sample. Depending on the phytoplankton density of the sample, a settling tower of 5, 10, or 25 ml was used. A cover glass was placed on top of tower and the sample allowed to settle in the dark in a vibration-free location. Minimum settling times were 17 h for 5-ml samples, 34 h for 10-ml samples, and 74 h for 25-ml samples. Enumeration was performed using a Nikon (Tokyo, Japan) Eclipse TE200 inverted microscope equipped with phase contrast optics. One ocular was fitted with a Whipple disk, which projects a grid on each microscope slide, to define the area of fields to be counted. Specimens that went beyond the left and bottom edges of the Whipple grid were not counted. A minimum of 10 and a maximum of 50 fields were counted at both 400× and 200×. An additional scan of the entire slide was made at 100× to count large and/or rare taxa. A minimum of 400–600 natural units per slide were counted to give a 95% confidence interval of the estimate within ± 10% of the sample mean. Quality control checks were performed at least once for every 10 samples counted and included a check for random distribution of cells (standard error among total number of natural units/field was calculated as the count was performed and should be ≤ 15%) and a replicate count (the difference between counts should be ≤ 15%).
Microscopy data were utilized to calculate species richness. Species richness (s; number of species), was used as a simple index of phytoplankton diversity (CitationBegon et al. 1990). All species observed even once were included in the calculation of s. Changes in richness in response to nutrient additions were compared in different phytoplankton groups. Species abundances were reported for dominant taxonomic groups (at least 24 cells of each taxonomic group were counted).
Results
July 2000 bioassay
Nutrient concentrations in Lake George were low during the July 2000 bioassay (). The molar DIN:DIP ratio was 4.6, which predicted N limitation of phytoplankton productivity and growth when compared to the Redfield N:P ratio needed for balanced phytoplankton growth (16:1; Redfield 1958). Primary productivity responded positively to additions of both N and P individually and increased most profoundly in response to the addition of both N and P combined (Bonferroni, p < 0.05; ). Chlorophyll a concentration was significantly enhanced by the addition of N and P combined (Bonferroni, p < 0.05; ). Nitrogenase activity increased significantly in response to the P and NP treatments (Bonferroni, p < 0.05; ). The phytoplankton community included relatively equal proportions of cryptophytes and cyanobacteria, a smaller amount of chlorophytes and diatoms, and a very small but detectable proportion of dinoflagellates (). Group-specific increases in biomass relative to the control occurred in cyanobacteria with the P and NP treatments (Bonferroni, p < 0.05; ). Within the cyanobacteria, there were some species-specific increases in response to nutrient treatments. Aphanocapsa spp. and Planktolyngbya undulata cell counts increased in each of the nutrient treatments relative to the control (). Cylindrospermopsis raciborskii and Planktolyngbya contorta both showed an increase in cell counts in response to the N and NP treatments.
Table 3 Microscopic counts of natural units (filaments) of dominant cyanobacteria in each of the experiments.
Figure 2 Primary productivity measurements following the 96-hr bioassay incubations. Treatments include the unamended control, nitrogen addition (N), phosphorus addition (P) and nitrogen and phosphorus addition (NP). Bars are the mean of five replicates, error bars are one standard deviation, and the lower case letters group the means according the Bonferroni post-hoc comparison.
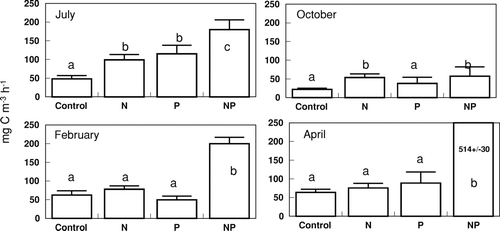
Figure 3 Chlorophyll a measurements following the 96-hr bioassay incubations. Treatments include the unamended control, nitrogen addition (N), phosphorus addition (P) and nitrogen and phosphorus addition (NP). Bars are the mean of five replicates, error bars are one standard deviation, and the lower case letters group the means according the Bonferroni post-hoc comparison.
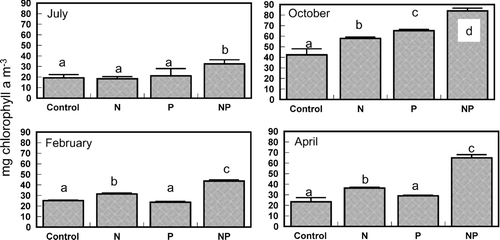
Figure 4 Acetylene reduction measurements following the 96-hr bioassay incubations. Treatments include the unamended control, nitrogen addition (N), phosphorus addition (P) and nitrogen and phosphorus addition (NP). Bars are the mean of five replicates, error bars are one standard deviation, and the lower case letters group the means according the Bonferroni post-hoc comparison. Acetylene reduction was not detected in February, as indicated by the ND notation.
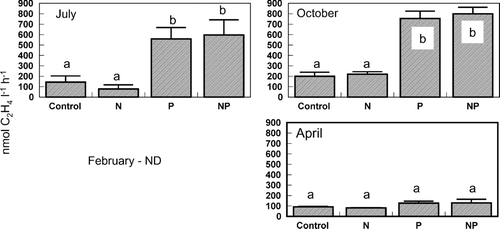
Figure 5 Taxonomic composition of the phytoplankton following the 96-hr bioassay incubations. HPLC measurements of diagnostic pigments were used to partition the total Chlorophyll a into taxonomic groups. Treatments include the unamended control (C), nitrogen addition (N), phosphorus addition (P) and nitrogen and phosphorus addition (NP). Bars are the mean of five replicates and represent the percentage of the phytoplankton community that was in each taxonomic group.
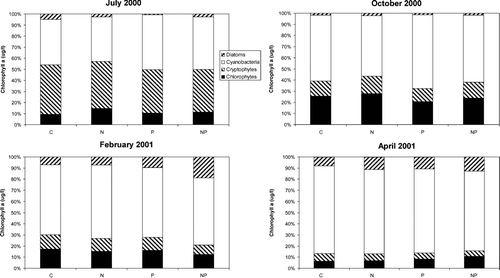
October 2000 bioassay
The DIN:DIP ratio during the October 2000 bioassay in Lake George was 18 (very close to the Redfield ratio) and predicted neither N nor P limitation of phytoplankton productivity and growth. Dissolved inorganic nutrient concentrations in Lake George were again relatively low (). Primary productivity was significantly higher relative to the control in the N or NP treatments (Bonferroni, p < 0.05; ). Chlorophyll a concentration was enhanced above the control in increasing amounts by the N, P, and NP treatments, respectively (Bonferroni, p < 0.05) (). Nitrogenase activity was again increased significantly above control rates by the P and NP treatments (Bonferroni, p < 0.05); ). Cyanobacteria were the dominant component of the phytoplankton community, followed by chlorophytes, cryptophytes, and a small proportion of diatoms (). Total biomass of cryptophytes, chlorophytes, and diatoms increased relative to the control only in the NP treatment (Bonferroni, p < 0.05). Gains in biomass relative to the control occurred in cyanobacteria with both the P and NP treatments (Bonferroni, p < 0.05; ). In terms of cell counts, only NP appeared to increase total cyanobacterial numbers (). The most abundant cyanobacteria present included Pseudoanabaena/Limnothrix spp., an unknown species, C. raciborskii, P. undulata, and Aphanocapsa spp., in descending order of prevalence (). Responses of the specific cyanobacteria to nutrient additions included no response (Pseudoanabaena/Limnothrix spp.), equivalent stimulation by the N and NP treatments (P. undulata), equivalent stimulation by the N and NP treatments and even higher stimulation by the P treatment (C. raciborskii), and stimulation only by NP treatment (unknown cyanophyte, Aphanocapsa spp.; ).
February 2001 bioassay
Both the DIN concentration (specifically the NH4 + concentration) and the DIN:DIP during the February 2001 bioassay were the highest measured during this set of experiments (). Primary productivity was only enhanced by the NP addition (Bonferroni, p < 0.05; ). Chlorophyll aconcentration was significantly higher than the control in the N treatment and further increased in response to the NP treatment (Bonferroni, p < 0.05; ). Nitrogenase activity was not detected during this experiment. The phytoplankton community during the February experiment was comprised mostly of cyanobacteria, with chlorophytes, cryptophytes, and diatoms making up the remainder of phytoplankton biomass (). Group-specific increases in biomass occurred in the NP treatment in the diatoms, chlorophytes, and cryptophytes (Bonferroni, p < 0.05; ). As a group, cyanobacteria were neither enhanced by any specific nutrient treatment () nor did they show evidence of large increase in biomass when examined at the species level (). The same response pattern was observed in the total cell counts (). There was some indication of enhancement by NP of an unknown filamentous species and unidentified spherical unicells (). However, the dominant cyanobacteria during this experiment (Aphanocapsa spp.) appeared to decrease in abundance in response to all nutrient additions ().
Table 4 Microscopic counts of taxonomic groups in each of the experiments. Cells belonging to Dinophyceae, Euglenophyceae, and Chrysophyceae were also detected at times but at much lower abundances than the groups shown.
April 2001 bioassay
Nutrient concentrations were relatively low again during the April 2001 bioassay. The DIN:DIP ratio was also low (∼ 6) and predicted N limitation of phytoplankton productivity (). There was a large increase in phytoplankton primary productivity relative to the control in the NP addition, but no significant increases in response to the other treatments (Bonferroni, p < 0.05; ). Chlorophyll a concentration, however, was enhanced by both the addition of N alone and enhanced further by NP treatments relative to the control (Bonferroni, p < 0.05; ). Nitrogenase activity was detected during the bioassay, but nutrient additions did not increase rates relative to the control (). Cyanobacteria were the dominant component of the phytoplankton community, which also included some diatoms and chlorophytes and a few cryptophytes (). Abundances of diatoms and chlorophytes were enhanced relative to the control by the NP treatment (Bonferroni, p < 0.05; ), the same pattern was observed in the total cell counts (). Cyanobacteria were also enhanced by the NP treatment compared to the control, as well as the P treatment (Bonferroni, p < 0.05; ). Cell counts showed no increase in cyanobacteria in response to the P treatment, but did indicate increases in response to N and NP (). The highest diversity in the cyanobacterial community in this set of bioassays was observed in April. Most of the cyanobacteria showed a positive response to NP. However, Pseudoananabaena/Limnothrix sp. and Glaucospira laxissima also responded favorably to the N treatment, and C. raciborskii did not respond to any nutrient additions ().
Species richness
Overall, species richness values were higher in the February and April experiments than in the July and October experiments (). There were no consistent changes in richness in response to the nutrient treatments. In the July experiment, the richness value for every nutrient addition treatment was higher than in the control. In October and February, there were only small differences among nutrient treatments in richness values. In April, richness was higher in the NP treatment than in the other treatments.
Table 5 Species richness values for each treatment at the end of each experiment.
Riverine and biologically fixed N
Moderate to strong La Niña conditions led to extended drought conditions that persisted from spring of 1999, which by 2000 had reduced the volume turnover rate in the 16 months from April 2000 through July 2001 to just 2.2 times. These conditions also increased the relative water volume contribution of artesian springs and direct precipitation to 20.0 and 20.3%, respectively, while reducing upstream input to 56% ().
Figure 6 Seasonal volume replacement for Lake George. Seasons are Dec–Mar, Apr–Jul, and Aug–Nov and conform generally to an annual pattern of cold-wet, hot-dry, and hot-wet. Upper St. Johns discharge obtained from USGS gauge 02236125, the St. Johns River at Astor. Artesian spring flow was determined by CitationStewart et al. (2006). Wet deposition was determined by Thiessen polygon from local rain gauges.
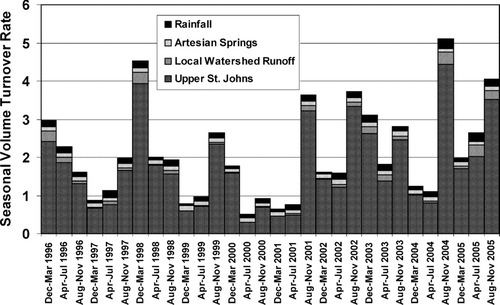
The impact of this N acquisition can be seen in a coarse nutrient budget for the lake, based on chemistry data collected at the inlet, outlet, and major inflows. From 1996 through 2005, the relative change between inlet and outlet (outlet–inlet) loads of conservative ions, based on chloride and total dissolved solids concentrations, was −1.9 and 1.3%, respectively, suggesting relatively good characterization of lake budget concentrations and flow volumes. During this time span, annual average total nitrogen concentration exiting the lake, after correcting for evapo-concentration, exceeded the incoming, flow-weighted concentration by 27%, while the exiting load exceeded incoming load by 17%. During moderate to low flow, long residence-time seasons, exiting-lake total nitrogen concentrations may exceed incoming concentration by more than 100% (), as was the case during the second year of this investigation. From 1996 through 2005, the average annual nitrogen load increase was 763 metric tons (MT) added per year, and ranged from 2,655 MT added in 1998, to 299 MT lost in 2002. Over this same time interval, exiting total phosphorus load decreased over incoming by 3.4%, representing a reasonably low settling rate for this large, shallow lake.
Figure 7 Percent change in Lake George outlet total nitrogen over volume-weighted inflow concentrations from 1996 through 2005. Lighter-dotted bars indicate investigation duration. Volume-weighting adjusted in-lake concentration based on fractions of volume added by major sources, and corrected for evapo-concentration.
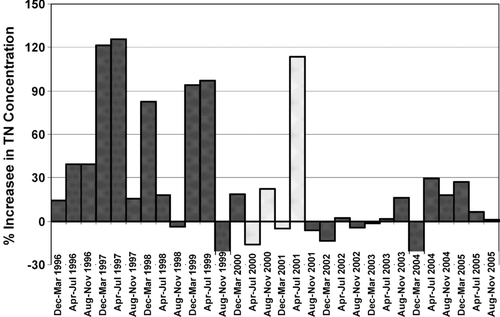
Water volume, nitrogen, phosphorus, and chloride budgets were calculated for Lake George for the period from 1996–2005. Whether a constituent was lost or gained as a result of processes in Lake George was determined by subtracting outflow load from inflow load (). During the 1996–2005 timeframe there were gains in the mass of total dissolved solids (TDS), total organic N, and total N. There were losses of water volume, chloride, inorganic N, PO4 and total phosphorus.
Table 6 Mean annual water volume, nitrogen, phosphorus, and chloride budgets for Lake George, 1996–2005.
Discussion
Nutrient manipulation bioassays allowed a direct measurement of the response of the phytoplankton community to changes in nutrient levels and permitted some determination of the nutrients that were limiting phytoplankton primary productivity and biomass accumulation. In Lake George, the phytoplankton-nutrient relationships were variable. Both N and P were limiting at different times, and the factors measured (chlorophyll a, primary productivity, diagnostic photopigment analysis, species composition) did not always show similar responses to nutrient additions. Considering the nature of the phytoplankton community in Lake George, particularly the presence of N2-fixing cyanobacterial populations, variable phytoplankton nutrient relationships were not surprising. Furthermore, CitationPhlips et al. (2000) found Lake George to be among the least light-limited parts of the St. Johns River System, underscoring the importance of nutrient controls of phytoplankton community dynamics in this lake.
Additions of N and P together most often enhanced phytoplankton biomass and primary production above levels measured in the individual additions of N and P. The responses of chlorophyll a and primary productivity were not always consistent among treatments, possibly because biomass changes account for the cumulative effects of nutrient manipulations, whereas primary productivity measurements reflect responses to nutrient conditions at specific periods of the experiment. Differences in the responses of primary productivity and biomass accumulation to nutrient manipulations were likely attributable to the fact that biomass changes are the result of cumulative effects of interactive nutrient limitations, such as changes in grazing and respiratory C loss, whereas primary productivity measurements showed the effects of the nutrient manipulations over a relatively short time interval. Environmental variables such as temperature and irradiance also contributed to determining the seasonal patterns in phytoplankton community structure and function.
The fact that P additions did not stimulate overall phytoplankton biomass accumulation and productivity during periods of dominance by N2 fixers was somewhat unexpected. Generally, N2-fixing cyanobacterial activity is thought to be stimulated by additional P (CitationHealy 1982, CitationPaerl 1990). Group- and species-level responses did reveal some positive responses of cyanobacteria, and more specifically N2-fixing cyanobacteria, to P additions. In the October experiment there was both a group-specific increase in cyanobacterial biomass and an increase in the abundance of the potentially toxic N2 fixer C. raciborskii. However, in the July experiment there was no positive response in cell numbers to P additions, and C. raciborskii was stimulated by N additions and not P additions. If DIN is available, N2-fixing cyanobacteria may preferentially use this source of N over fixing N2 due to the high energetic cost associated with N2 fixation. C. raciborskii in particular has a high uptake affinity for NH4 + that allows it to more effectively use this source of N (CitationPadisak and Reynolds 1998; Huszar et al. 2000). This will likely be a complicating factor in efforts to model N cycling and phytoplankton dynamics in this system, and will certainly need to be considered as management strategies are designed.
Diagnostic photopigment analyses of phytoplankton taxonomic groups indicated that phytoplankton community composition in Lake George was dominated by cyanobacteria throughout this study. However group-level identification was not completely sufficient in this case because of the presence of both N2 fixing and non-N2 fixing cyanobacteria in the system. Determining both the species and group level changes was critical to understanding the role of cyanobacteria in the biogeochemistry of the lake. Additionally, potentially toxic N2-fixing cyanobacteria such as C. raciborskii (CitationChapman and Schelske 1997) also have the potential to affect grazing and hence food web dynamics (CitationLeonard and Paerl 2005). Cell counts showed the lake's cyanobacteria were predominantly N2 fixers in the summer and fall and non-N2 fixers during winter and spring.
Fixation of N2 occurred at significant rates during the summer and fall and was enhanced by P additions (CitationSmith 1983, CitationSmith 1990). Although bioassays indicated that P was not the main nutrient controlling phytoplankton productivity and biomass accumulation, the potential increase in N from N2 fixation in P addition treatments was found to be potentially significant to the N budget. CitationHorne (1977) identified the potential for N2 fixation to supply excessive levels of N to lakes. In a Florida lake, CitationPhlips and Inhat (1995) found N2 fixation to be an important contributor to the lake nutrient budget. Here we considered the potential for the N from N2fixers to affect systems downstream of the lake. Because N controls are being implemented to control phytoplankton growth in the St. Johns River estuary, reducing P loads to Lake George may assist N control efforts downstream. Even without P additions, the potential contribution of N from N2 fixation was considerable. The Lake George N budget underscores the potential impact of N2 fixers activity on N exported from the lake downstream to the estuary.
Considerable progress has been made in identifying the physical-chemical conditions that favor the growth of cyanobacteria in natural waters (CitationSmith and Bennett 1999, CitationCanfield et al. 1989; CitationHavens 2003; CitationLevine and Schindler 1999). In Florida lakes, N:P ratio (CitationHavens 2003; CitationHavens et al. 2003) and total phytoplankton biomass (CitationDowning et al. 2001) have been found to be correlated with cyanobacterial dominance. In this study we found that experimentally lowering the N:P ratio through P additions increased the rates of N2 fixation when N2 fixers were present and increased the proportion of the community made up of cyanobacteria. However, the proportion of the phytoplankton comprised of cyanobacteria never decreased with an increase in the N:P ratio following N additions, despite the fact that N2-fixing cyanobacteria would be expected to be less competitive in higher N:P conditions.
Because the experimental portion of this study was based on relatively short term manipulations, it was not appropriate to draw conclusions about large scale and long term, continuous correlations between cyanobacteria and their chemical and physical environment. However, our data demonstrate some general difficulties associated with determining accurate predictors of changes in cyanobacterial dominance. One of the negative impacts of human activities that cause shifts towards nuisance species is the potential for loss of biodiversity. During periods in which N2-fixing cyanobacteria were dominant, species richness values were considerably lower than those times when N2-fixing cyanobacteria were not dominant. Among the dominant N2-fixing cyanobacteria was C. raciborskii. This invasive N2-fixing cyanobacterium has been increasing in prevalence in Florida's lakes and rivers (CitationChapman and Schelske 1997) because of increased nutrient supplies and could lead to a decrease in overall species richness in the lake phytoplankton.
Nitrogen-fixing cyanobacteria represented an important component of the phytoplankton of Lake George and their activity contributed to the N budget of the lake and thus the N load to the estuary. Efforts to reduce anthropogenic nutrient loading to the estuaries with significant freshwater areas like the St Johns, must consider the potential N contributions from upstream populations of N2-fixing cyanobacteria. Changes in the lake's nutrient regime will affect the abundance and activity of cyanobacteria, which in turn affect cyanobacterial activity and the nutrients that are transported downstream. Additionally, nitrogen-fixing cyanobacteria are also often toxic, which can be of concern for human and ecosystem health (CitationBourke et al. 1983; CitationSaker et al. 1999, CitationSaker and Eaglesham 1999). Finally, cyanobacteria are often less palatable than some other phytoplankton, and thus may disrupt trophic transfer if they become more prevalent (CitationHolm et al. 1983; CitationFulton and Paerl 1988; CitationSellner 1997; CitationLeonard and Paerl 2005).
Nutrient management in the freshwater marine continuum requires an understanding of the changes in sources and processing of nutrients throughout the range of aquatic systems. One of the products of research on phytoplankton-nutrient relationships in coastal freshwater systems is an improved understanding of to the links of the freshwater system to downstream estuarine ecosystems. Most often this interaction is effectively quantified by gauging the volume of freshwater delivered downstream and the load of important nutrients. We have presented an example of how complex nutrient management can become in systems with N2-fixing cyanobacteria. Cyanobacteria in the SJRS fixed significant quantities of N, dominated community structure, reduced phytoplankton diversity, and appeared to thrive on additional inorganic nitrogen. During this study, Lake George was a significant source of N to downstream systems. Eutrophication control in this and similar lake systems will likely require reductions of both nitrogen and phosphorus loading to improve their water quality and that of the estuaries downstream.
Acknowledgments
We thank the St. Johns River Water Management District and the U.S. EPA-STAR program (R82867701) and NSF DEB-0452324 for funding this work. B. Peierls, A. Waggener, S. Ensign, M. Waters and S. McMillan provided valuable editorial suggestions. The comments and suggestions of the reviewers significantly enhanced this manuscript. C. Stevenson, B. Chang, B. Hendrickson, A. Waggener, J. Leonard, P. Sanderson and J. Joyner contributed to field and laboratory analyses.
Notes
1Concentrations determined from land use—water quality relationships and flow predictions from the Lower St. Johns River Basin Watershed Model (CitationHendrickson and Konwinski 1998).
2From Pollman and Roy 2003.
References
- Begon , M. , Harper , J. L. and Townsend , C. R. 1990 . Ecology: Individuals, Populations and Communities , Oxford : Blackwell Scientific Publications .
- Bourke , A. T. C. , Hawes , R. B. , Neilson , A. and Stallman , N. D. 1983 . An outbreak of Hepato-enteritis (The Palm Island mystery disease) possibly caused by algal intoxication . Toxicon (Suppl) , 3 : 45 – 48 .
- Canfield , D. E. Jr. , Phlips , E. and Duarte , C. M. 1989 . Factors influencing the abundance of blue-green algae in Florida lakes . Can. J. Fish. Aquat. Sci. , 46 : 1232 – 1237 .
- Chapman , A. D. and Schelske , C. L. 1997 . Recent appearance of Cylindrospermopsis (Cyanobacteria) in five hypereutrophic Florida lakes . J. Phycol. , 33 : 191 – 195 .
- Downing , J. A. , Watson , S. B. and McCauley , E. 2001 . Predicting cyanobacterial dominance in lakes . Can. J. Fish. Aquat. Sci. , 58 : 1905 – 1908 .
- Flett , R. J. , Schindler , D. W. , Hamilton , R. D. and Campbell , N. E. R. 1980 . Nitrogen fixation in Canadian Precambrian Shield lakes . Can. J. Fish. Aquat. Sci. , 37 : 494 – 505 .
- Fulton , R. S. and Paerl , H. W. 1988 . Effects of the blue-green algae Microcystis aeruginosa on zooplankton competitive relations . Oecologia , 76 : 383 – 389 .
- Harris , G. P. 1999 . Comparison of the biogeochemistry of lakes and estuaries: ecosystem processes, functional groups, hysteresis effects and interactions between macro- and microbiology . Mar. Freshw. Res. , 50 : 791 – 811 .
- Havens , K. 2003 . Phosphorus-algal bloom relationships in large lakes of South Florida: Implications for establishing nutrient criteria . Lake Reserv. Manage. , 19 : 222 – 228 .
- Havens , K. E. , James , R. T. , East , T. L. and Smith , V. H. 2003 . N:P ratios, light limitation, and cyanobacterial dominance in a subtropical lake impacted by non-point source nutrient pollution . Environ. Pollut. , 122 : 379 – 390 .
- Healy , F. P. 1982 . “ Phosphate ” . In The Biology of Cyanobacteria , Edited by: Carr , N. G. and Whitton , B. A. 105 – 124 . Oxford : Blackwell Scientific Publications .
- Hendrickson , J. C. and Konwinski , J. 1998 . Seasonal Nutrient Import-Export Budgets for the Lower St. Johns River, Florida Final Report to the Florida Department of Environmental Protection, Contract No. WM598. 109 pp
- Holm , N. P. , Granfand , G. G. and Shapiro , J. 1983 . Feeding and assimilation rates of Daphnia pulex fed Aphanizomenon flos-aquae . Limnol. Oceanogr. , 28 : 677 – 687 .
- Horne , A. J. 1977 . Nitrogen fixation—a review of this phenomenon as a polluting process . Prog. Water Technol. , 8 : 359 – 37 .
- Howarth , R. W. 1988 . Nutrient limitation of net primary production in marine ecosystems . Ann. Rev. Ecol. Syst. , 19 : 89 – 110 .
- Huszer , V. L. M. , Silva , L. H. S. , Marinho , M. , Domingos , P. and Sant'Anna , C. L. 2000 . Cyanoprokaryote assemblages in eight productive tropical Brazilian waters . Hydrobiologia , 424 : 67 – 77 .
- Jeffrey , S. , Mantoura , R. and Wright , S. , eds. 1997 . Phytoplankton Pigments in Oceanography: Guidelines to Modern Methods , Paris : UNESCO .
- Leonard , J. and Paerl , H. W. 2005 . Zooplankton community structure, microzooplankotn grazing impact and seston energy content in the St Johns River System, Florida as influenced by the toxic cyanobacterium Cylindrospermopsis raciborskii. . Hydrobiologia , 537 : 89 – 97 .
- Levine , S. N. and Schindler , D. W. 1999 . Influence of nitrogen to phosphorus supply ratios and physicochemical conditions on cyanobacteria and phytoplankton species composition in the Experimental Lakes Area Canada . Can. J. Fish. Aquat. Sci. , 56 : 451 – 466 .
- Mackey , M. D. , Mackey , D. J. , Higgins , H. W. and Wright , S. W. 1996 . CHEMTAX–a program for estimating class abundances from chemical markers: application to HPLC measurements of phytoplankton . Mar. Ecol. Prog. Ser. , 144 : 265 – 283 .
- Mackey , M. D. , Higgins , H. W. , Mackey , D. J. and Wright , S. W. 1997 . CHEMTAX user's manual: a program for estimating class abundances from chemical markers–application to HPLC measurements of phytoplankton pigments , CSIRO Division of Marine Research .
- Millie , D. F. , Paerl , H. W. and Hurley , J. P. 1993 . Microalgal pigment assessments using high-performance liquid chromatography: A synopsis of organismal and ecological applications . Can. J. Fish. Aquat. Sci. , 50 : 2513 – 2527 .
- Moore , D. S. and McCabe , G. P. 1993 . Introduction to the Practice of Statistics , New York : W. H. Freeman and Co. .
- National Research Council . 2000 . Clean Coastal Waters: Understanding and Reducing the Effects of Nutrient Pollution , Washington , D. C. : National Academy Press .
- Padisak , J. and Reynolds , C. S. 1998 . Selection of phytoplankton associations in Lake Balaton, Hungary, in response to eutrophication and restoration measures, with special reference to the cyanoprokaryotes . Hydrobiologia , 384 : 41 – 53 .
- Paerl , H. W. 1987 . Dynamics of blue-green algal blooms in the lower Neuse River, North Carolina: causative factors and potential controls , Raleigh : UNC Water Resources Research Institute. North Carolina State University . Rep. No. 229
- Paerl , H. W. 1990 . “ Physiological ecology and regulation of N2 fixation in natural waters ” . In Advances in Microbial Ecology , Edited by: Marshall , K. C. 305 – 344 . Plenum Publishing Corporation .
- Paerl , H. W. 1998 . Structure and function of anthropogenically altered microbial communities in coastal waters . Curr. Opin. Microbiol. , 1 : 296 – 302 .
- Paerl , H. W. , Valdes , L. M. , Piehler , M. F. and Lebo , M. E. 2004 . Solving problems resulting from solutions: The evolution of a dual nutrient management strategy for the eutrophying Neuse River Estuary, North Carolina, USA . Environmental Science & Technology , 38 : 3068 – 3073 .
- Palmer , M. , Bernhardt , E. , Chornesky , E. , Collins , S. , Dobson , A. , Duke , C. , Gold , B. , Jocobson , R. , Kingsland , S. , Kranz , R. , Mappin , M. , Martinez , M. L. , Micheli , F. , Morse , J. , Pace , M. , Pascual , M. , Palumbi , S. , Reichman , O. J. , Simons , A. , Townsend , A. and Turner , M. 2004 . Ecology for a crowded planet . Science , 304 : 1251 – 1252 .
- Phlips , E. J. and Inhat , J. 1995 . “ Planktonic nitrogen fixation in a shallow subtropical lake (Lake Okeechobee, Florida, USA) ” . In Advances in Limnology Edited by: Aumen , N. G. and Wetzel , R. G. 191 – 201 . Schweizerbart, Stuttgart , , Germany
- Phlips , E. J. , Cichra , M. , Aldridge , F. J. , Jembeck , J. , Hendrickson , J. and Brody , R. 2000 . Light availability and variations in phytoplankton standing crops in a nutrient-rich blackwater river . Limnol. Oceanogr. , 45 : 916 – 929 .
- Piehler , M. F. , Dyble , J. , Moisander , P. H. , Pinckney , J. L. and Paerl , H. W. 2002 . Effects of modified nutrient concentrations and ratios on the structure and function of the native phytoplankton community in the Neuse River Estuary, North Carolina USA . Aquat. Ecol. , 36 : 371 – 385 .
- Pinckney , J. L. , Paerl , H. W. , Harrington , M. B. and Howe , K. E. 1998 . Annual cycles of phytoplankton community-structure and bloom dynamics in the Neuse River Estuary, North Carolina . Mar. Biol. , 131 : 371 – 381 .
- Pollman , C. D. and Roy , S. 2003 . Examination of atmospheric deposition chemistry and its potential effects on the Lower St. Johns Estuary , Gainesville , FL : Tetra Tech, Inc. . Final report submitted to St. Johns River Water Management District
- Redfield , A. C. 1958 . The biological control of chemical factors in the environment . Am. Sci. , 46 : 205 – 222 .
- Rowan , K. 1989 . Photosynthetic pigments of the algae , New York : Cambridge University Press .
- Saker , M. L. , Thomas , A. D. and Norton , J. H. 1999 . Cattle mortality attributed to the toxic cyanobacterium Cylindrospermopsis raciborskii in an outback region of north Queensland . Environ. Toxicol. , 14 : 179 – 183 .
- Saker , M. L. and Eaglesham , G. K. 1999 . The accumulation of cylindrospermopsin from the cyanobacterium Cylindrospermopsis raciborskii in tissues of the Redclaw crayfish Cherax quadricarinatus . Toxicon , 37 : 1065 – 1077 .
- Schindler , D. W. 1977 . Evolution of phosphorus limitation in lakes . Science , 195 : 260 – 262 .
- Sellner , K. G. 1997 . Physiology, ecology, and toxic properties of marine cyanobacteria blooms . Limnol. Oceanogr. , 42 : 1089 – 1104 .
- Smith , V. H. 1983 . Low nitrogen to phosphorus ratios favor dominance by blue-green algae in lake phytoplankton . Science , 221 : 669 – 671 .
- Smith , V. H. 1990 . Nitrogen, phosphorus, and nitrogen fixation in lacustrine and estuarine ecosystems . Limnol. Oceanogr. , 35 : 1852 – 1859 .
- Smith , V. H. and Bennett , S. J. 1999 . Nitrogen:phosphorus supply ratios and phytoplankton community structure in lakes . Arch. Hydrobiol. , 146 : 37 – 53 .
- Stewart , W. , Fitzgerald , G. and Burris , R. 1967 . In-situ studies of N2-fixation using the acetylene reduction technique . Proc. Natl. Acad. Sci. USA , 58 : 2071 – 2078 .
- Stewart Sucsy , J. P. and Hendrickson , J. Meteorological factors affecting estuarine conditions within Lake George in the St. Johns River, Florida . Proceedings of the 7th International Conference on HydroScience and Engineering .
- Tester , P. A. , Geesey , M. E. , Guo , C. , Paerl , H. W. and Millie , D. F. 1995 . Evaluating phytoplankton dynamics in the Newport River estuary (NC, USA) by HPLC-derived pigment profiles . Mar. Ecol. Prog. Ser. , 124 : 237 – 245 .
- Vergara , B. A. 2000 . District Water Supply Plan , Palatka, Fla. : St. Johns River Water Management District . Special Publication SJ2000-SP1
- Vitousek , P. M. , Mooney , H. A. , Lubchenco , J. and Melillo , J. M. 1997 . Human domination of earth's ecosystems . Science , 277 : 494 – 499 .
- Welschmeyer , N. A. 1994 . Fluorometric analysis of chlorophyll a in the presence of chlorophyll b and pheopigments . Limnol. Oceanogr. , 39 : 1985 – 1992 .
- Winkler , S. and Ceric , A. 2004 . Status and trends in water quality at selected sites in SJRWMD. Technical Publication SJ2004-3, St. Johns River Water Management District, Palatka, FL
- Wright , S. W. , Thomas , D. P. , Marchant , H. J. , Higgins , H. W. , Mackey , M. D. and Mackey , D. J. 1996 . Analysis of phytoplankton of the Australian sector of the Southern Ocean: comparisons of microscopy and size frequency data with interpretations of pigment HPLC data using the ‘CHEMTAX’ matrix factorisation program . Mar. Ecol. Prog. Ser. , 144 : 285 – 298 .