Abstract
Objective
Athletes are susceptible to acute respiratory tract infections, including SARS-CoV-2, which can affect cardiovascular function. We aimed to evaluate the impact of COVID-19 infection and quarantine on cardiac function in male and female collegiate athletes.
Methods
We conducted a single-center, prospective, case-control study and performed transthoracic echocardiography in a diverse group of convalescent SARS-CoV-2-positive athletes following a 10–14–day quarantine, matched to non-SARS-CoV-2 athletes. Data collection occurred from August 1, 2020, to May 31, 2021.
Results
We evaluated 61 SARS-CoV-2-positive athletes (20 ± 1 years, 39% female) and 61 controls (age 20 ± 2 years, 39% female). Echocardiography in SARS-CoV-2-positive athletes was performed on average 40 ± 38 days after infection diagnosis. All SARS-CoV-2-positive athletes had clinically normal systolic left ventricular function (LVEF > 50%). However, SARS-CoV-2-positive athletes exhibited mildly lower LVEF compared to controls (65 ± 6% vs. 72 ± 8%, respectively, p < 0.001), which remained significant when evaluated separately for female and male athletes. Sub-analysis revealed these differences occurred only when imaging occurred within a mean average of 27 days of infection, with a longer recovery period (≥27 days) resulting in no differences. SARS-CoV-2-positive male athletes exhibited higher left ventricular end-diastolic volume and mitral filling velocities compared to male controls.
Conclusion
Our study reveals unique sex-specific cardiac changes in collegiate athletes following SARS-CoV-2 infection and quarantine compared to controls. Despite a mild reduction in LVEF, which was only observed in the first weeks following infection, no clinically significant cardiac abnormalities were observed. Further research is required to understand if the changes in LVEF are directly attributed to the infection or indirectly through exercise restrictions resulting from quarantine.
Introduction
In the early stages of the Coronavirus disease 2019 (COVID-19) pandemic, collegiate athletes diagnosed with severe acute respiratory syndrome coronavirus 2 (SARS-CoV-2) infection were subjected to a series of direct and indirect perturbations that could alter cardiovascular function. Possible direct SARS-CoV-2 cardiac involvement included myocardial and pericardial abnormalities, with reports from multiple large-scale prospective studies suggesting a prevalence of approximately 1% in competitive athletes across various disciplines [Citation1–3]. Notably, the potential direct cardiac insult associated with COVID-19 occurs in the context of additional concomitant indirect effects that may combine to alter cardiovascular function and athletic performance adversely. Following diagnosis with COVID-19, athletes are required to undergo a quarantine period with concurrent training restrictions. These procedures aimed to reduce viral transmission and minimize the risk of health complications. Depending on the initial level of physical conditioning and the severity of detraining, reductions in circulating blood volume, left ventricular wall thickness, and chamber size may occur within the early stages of reduced training load in elite competitive athletes [Citation4,Citation5]. Thus, collegiate athletes presenting with COVID-19, or similar acute respiratory illnesses requiring time off from training may be vulnerable to changes in the cardiovascular system that may cause symptoms or reduced exercise performance in the weeks following infection.
An important consideration is that although a similar proportion of female collegiate athletes have been reported to test positive for SARS-CoV-2 infection, the impact of the combined direct and indirect effects of COVID-19 on cardiac morphology in female athletes remains poorly understood [Citation1,Citation6]. To date, the sex-disaggregated symptomatic data in collegiate athletes suggest that males and females report a similar proportion of persistent symptoms (symptoms lasting >3 weeks) following SARS-CoV-2 infection, but a greater proportion of female competitive athletes reported exertional cardiopulmonary symptoms compared to males [Citation7]. Moreover, the physiological and morphological differences reported between males and females following exercise adaptation suggest that the cardiac adaptation to prescribed detraining following infection may also differ.
Before the COVID-19 pandemic, Valtonen et al. (2019) demonstrated that many respiratory viruses identified in Olympic athletes following acute respiratory illnesses were certain strands of coronaviruses [Citation8]. However, since the COVID-19 outbreak began, SARS-CoV-2 has been the primary pathogen causing acute respiratory infection in the general population [Citation9] and in athletes [Citation10,Citation11]. In addition, recent work found an incidence of 4.7 (per 1000 athlete days) of acute respiratory illness in athletes [Citation12]. Therefore, given the high prevalence of acute respiratory infections in collegiate athletes, which can cause absence from training and competition, important lessons can be learned from evaluating the cardiovascular changes following COVID-19 diagnosis and subsequent quarantine procedures [Citation12,Citation13].
We therefore prospectively planned the present study to define the echocardiographic features of male and female collegiate athletes in the weeks following SARS-CoV-2 infection and a strict quarantine procedure with exercise restriction. We hypothesized that athletes following SARS-CoV-2 infection and quarantine would demonstrate significant sex-specific cardiac remodeling changes compared to matched healthy, non-SARS-CoV-2 infected athlete controls that would persist for several weeks following the quarantine period. We also hypothesized that these differences would only be evident in the athletes tested within the first few weeks following infection. In order to scrutinize this hypothesis, we conducted transthoracic echocardiography on each athlete in a timeframe after they resumed athletic activity. Our objective was to ascertain the presence of any noteworthy modifications in cardiovascular function.
Methods
Study design
The current investigation was a single-center case-control study involving current NCAA Division 1 collegiate athletes with a confirmed positive SARS-CoV-2 diagnosis during the fall 2020 semester and matched non-SARS-CoV-2 athlete controls. Individuals were eligible to enroll if they were currently a student-athlete at Kansas State University, actively training or competing, and undergoing weekly screening for SARS-CoV-2 infection.
Following a positive SARS-CoV-2 test confirmed via standard clinical laboratory procedures (PCR or antigen) performed by Kansas State University medical staff and completion of mandatory quarantine, athletes were contacted about the study. All enrolled SARS-CoV-2-recovered participants were propensity score matched (1:1) to non-SARS-CoV-2 athlete controls, as determined by negative clinical laboratory procedures (PCR, antigen, or antibody) based on age, biological sex, race, and body mass index to improve statistical power as is common in case-control studies [Citation14]. SARS-CoV-2-recovered participants were scheduled for transthoracic echocardiography following medical clearance for return to regular activity. The duration between diagnosis and echocardiography measurement varied due to scheduling challenges with individual athletes, with a mean average of 40 days (27-day median average). This study protocol was in accordance with the ethical standards of the institution or national research committee. Approval was received by the Institutional Review Board of Kansas State University and conformed to the standards set by the Declaration of Helsinki (Kansas State University Research Compliance Office Federalwide Assurance: FWA00000865). Verbal and written informed consent were obtained from all participants included in the study.
Measurements
Transthoracic echocardiography (GE Medical Systems Ltd., Vivid S6) was performed, using the American College of Cardiology guidelines for competitive athletes and highly active people for return to play status after SARS-CoV-2 diagnosis as guidance [Citation15]. All non-SARS-CoV-2 participants completed a transthoracic echocardiography evaluation following a negative SARS-CoV-2 test. A trained echocardiographer conducted all echocardiography measures with a 1.5- to 4.3-MHz phased array transducer. Standard views were acquired, and measurements of the left ventricular chamber were quantified per published guidelines [Citation16]. Cutoff values for suspected SARS-CoV-2-induced myocardial injury included left ventricular end-diastolic diameter (LVEDD) of >70 mm in men and >60 in women as a measure of severe left ventricular (LV) dilatation and LV ejection fraction (LVEF) <50% as previously described [Citation15]. In addition, we assessed arterial blood pressure via an automated blood pressure monitor before imaging following a 10 min rest period in the supine position (Omron HealthCare Co. Ltd. HEM-907XL Kyoto, Japan).
Statistical analysis
Participant characteristics are described with continuous data presented as mean ± SD, and categorical data are presented as counts and percentages. For normally distributed outcomes, comparisons between positive SARS-CoV-2 and controls were analyzed with independent samples t-test. Non-normally distributed data were analyzed with the Mann-Whitney U-test. Given the potential differences in PCR testing relative to antigen for SARS-CoV-2, we performed a subanalysis that included only PCR-confirmed SARS-CoV-2 infection. All significance tests were two-sided, using p < 0.05 as the level of statistical significance. Given the variability in time between SARS-CoV-2 and echocardiography measurements, positive SARS-CoV-2 were grouped based on the duration between diagnosis and study enrollment. Groups were defined as within 27 days of diagnosis and to ≥27 days and was chosen as it represented the median duration from diagnosis in our study sample. Prism (version 7.04, Graphpad software, INC., La Jolla, CA) data analysis software was used for all statistical analysis.
Results
Study population
A total of 68 athletes had a positive SARS-CoV-2 and agreed to participate in the study; however, 7 were excluded from the analysis due to missing/incomplete cardiac images. In the 61 SARS-CoV-2-positive athletes (20 ± 1 yrs., 39% female), cardiac images were obtained on average 40 days post-infection and compared to 61 matched controls (age 20 ± 2 yrs., 39% female). SARS-CoV-2 positive athletes were involved in the following sports: baseball (6), cheerleading (5), football (26), men’s basketball (2), rowing (4), track & field (6), volleyball (1), women’s basketball (2), and women’s soccer (9). SARS-CoV-2 negative athletes (n = 61) participated in the following sports: baseball (25), cheerleading (5), football (1), men’s basketball (4), rowing (8), track & field (4), volleyball (2), women’s basketball (2), women’s soccer (7), and women’s tennis (3). At the time of infection, 44% of the SARS-CoV-2-positive athletes reported having mild illness, and 56% were asymptomatic. No athlete reported cardiac-related symptoms.
Upon analysis, most cardiac wall and cavity dimensions were similar in the athletes with a history of SARS-CoV-2 infection compared to matched controls ( and ). Left ventricular end-diastolic diameter (LVEDD) was similar between SARS-CoV-2 positive athletes compared to controls. This similarity remained in the female athletes (). However, males exhibited a slightly higher absolute LVEDD than controls (5.2 ± 0.5 vs. 5.0 ± 0.4 cm, p = 0.01) (). Upon further investigation, in SARS-CoV-2 positive male athletes imaged within 27 days of diagnosis, LVEDD was significantly higher compared to controls (3.2 ± 0.4 vs. 3.5 ± 0.5, p = 0.02), but in those SARS-CoV-2 positive male athletes imaged ≥27 days post-diagnosis there was no significant difference (3.3 ± 0.6 vs. 3.6 ± 0.3 cm, p = 0.2). Importantly, no athletes with a history of SARS-CoV-2 had the presence of disproportionate or severe LV dilation (LVEDD >7 cm in males and >6 cm in females). Left ventricular intraventricular septal dimension in diastole (IVSDd) was similar between SARS-CoV-2 positive athletes compared to controls (1.0 ± 0.2 vs. 1.1 ± 0.2 mm, p = 0.07) (). However, when separating female and male athletes, the COVID-positive male athletes had a lower IVSDd than controls (1.1 ± 0.2 vs.1.2 ± 0.2 mm, p = 0.04) (), with no differences detected in females (1.0 ± 0.2 vs. 1.0 ± 0.2 mm, p = 0.7) ().
Figure 1. Comparison of interventricular septal wall thickness at end-diastole (IVSDd), left ventricular ejection fraction (LVEF), and Tissue-Doppler ratio of myocardial early (E) and late (A) ventricular filling velocity in collegiate athletes during the convalescent stage of SARS-CoV-2 recovery compared to non-SARS-CoV-2 athlete controls. The dotted line represents a LVEF of 50%.
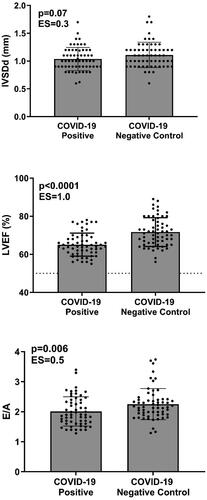
Figure 2. Comparisons of left ventricular end-diastolic diameter (LVEDD), left ventricular ejection fraction (LVEF), Tissue-Doppler myocardial early filling (e’), and interventricular septal wall thickness at end-diastole (IVSDd) in female athletes. LVEF was mildly lower in SARS-CoV-2 athletes compared to matched controls. The dotted line represents a LVEF of 50%.
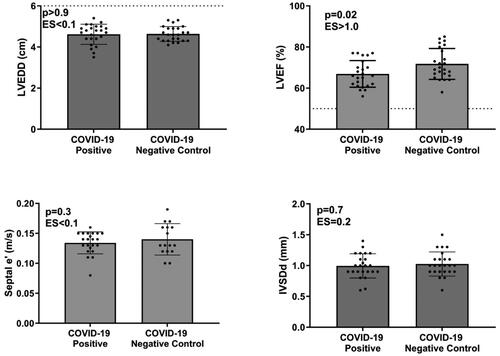
Figure 3. Comparisons of left ventricular end-diastolic diameter (LVEDD), left ventricular ejection fraction (LVEF), Tissue-Doppler myocardial early filling (e’), and interventricular septal wall thickness at end-diastole (IVSDd) in male athletes. LVEF was mildly lower in SARS-CoV-2 athletes compared to matched controls. The dotted line represents a LVEF of 50%.
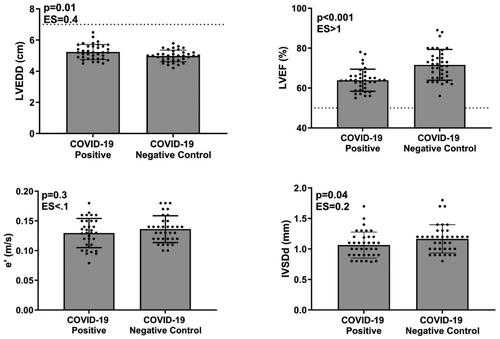
Table 1. Demographics, clinical characteristics, and echocardiographic imaging findings in SARS-CoV-2-positive athletes compared to matched negative controls.
LVEF was significantly lower in SARS-CoV-2 positive athletes compared to controls (65 ± 6% vs. 72 ± 8%, p < 0.0001) (). In contrast, peak systolic tissue velocity, an important marker of LV systolic function, was not different in SARS-CoV-2 positive athletes compared to controls. The difference in LVEF persisted when females and males were analyzed separately (male, 64 ± 6% vs. 72 ± 8%, p < 0.001 (); female, 67 ± 6% vs. 72 ± 8%, p = 0.02) (). A sub-analysis was performed to determine if the time between diagnosis and imaging influenced this finding. In SARS-CoV-2 positive female athletes imaged within 27 days of diagnosis, LVEF was significantly lower compared to controls (64 ± 6% vs. 73 ± 7% respectively, p = 0.01). A similar finding was observed in SARS-CoV-2 positive male athletes compared to controls (62 ± 4 vs. 72 ± 7% respectively, p < 0.001). However, in SARS-CoV-2 positive male and female athletes tested ≥27 days post-diagnosis, there were no significant differences compared to controls (female: 68 ± 6 vs. 71 ± 8%, p = 0.3; male: 65 ± 6 vs. 71 ± 9, p = 0.09). Importantly, LVEF was greater than 50% in all SARS-CoV-2-positive athletes in all instances, indicating normal systolic LV function. Moreover, peak systolic tissue velocity was not different when females and males were analyzed separately, supporting normal systolic LV function. These are clinically significant findings, as a value less than 50% has been identified as a ‘red flag’ for the plausibility of SARS-CoV-2 myocardial injury [Citation15].
Mitral valve early filling (E) and atrial (A) velocities were not different between groups; however, when assessing the mitral E and A diastolic filling velocities (E/A) ratio, SARS-CoVo2-positive athletes exhibited a lower E/A than controls (2.0 ± 0.5 vs. 2.3 ± 0.52, p = 0.006) (). The male athletes also noted this difference (1.89 ± 0.48 vs. 2.14 ± 0.47, p = 0.008). Tissue-Doppler assessments of the lateral myocardial wall revealed similar early filling (e’) velocities across groups. All athletes with a history of SARS-CoV-2 had age-specific Tissue-Doppler e’ ( and ) with a similar E/’e between groups. Tissue-Doppler myocardial systolic (s’) velocity was not different between groups.
Data were collected during the early stage of COVID testing for diagnosis status, and thus, some of our student-athletes had only received antigen (rapid) testing. Although antigen and PCR tests are relatively similar and both result in high sensitivity to detect SARS-CoV-2 [Citation17] we included a sub-analysis of only PCR-confirmed SARS-CoV-2 infection. This resulted in a total of 45 PCR-confirmed positive SARS-CoV-2 infections. Within this sub-analysis, LVEDD was similar across all groups. However, LVEF remained lower in the COVID-19-positive athletes compared to controls (67 ± 6 vs. 71 ± 7, p < 0.001). This difference persisted across male (65 ± 6 vs. 71 ± 7, respectively, p = 0.07) and female athletes (66 ± 6 vs. 71 ± 7, respectively, p = 0.003). Mitral valve or tissue-Doppler velocities were similar across all groups.
Discussion
This study aimed to examine the transthoracic imaging outcomes of female and male collegiate athletes during the early convalescent stage of COVID-19 infection after a mandatory quarantine period including exercise training restrictions. Our study revealed that the LVEF of recently recovered athletes was slightly lower than that of matched controls but still within the clinically normal range and occurred independently in both female and male athletes. Moreover, our analysis revealed that the lower LVEF only occurred in athletes imaged within 27 days of diagnosis and that athletes who had a longer recovery between diagnosis and imaging (i.e. ≥ 27 days) had no difference in LVEF compared to matched controls. In addition, and clinically meaningful, none of the athletes displayed an LVEF that would suggest SARS-CoV-2-induced cardiac injury, indicating that cardiovascular deconditioning caused by detraining during the quarantine period may have been the cause. This finding was supported by similar peak systolic LV tissue velocity, an index of systolic function, between groups. We also observed that SARS-CoV-2 positive male athletes had lower IVSDd and E/A with higher LVEDD than healthy controls, but we did not observe these differences in female athletes. The lower LVEDD in male athletes was also dependent on time, with no differences in LVEDD observed in SARS-CoV-2 positive male athletes imaged ≥27 days post-diagnosis compared to controls. Therefore, our analysis demonstrated that neither female nor male athletes exhibited clinical cardiac abnormalities consistent with direct SARS-CoV-2 cardiac injury, with similar responses in both groups [Citation15].
Previous reports regarding the impact of COVID-19 on cardiac function in competitive athletes have yielded mixed findings using varying methodologies to assess cardiac dysfunction over a relatively short-duration following infection. Recent work by Moulson et al. (2021) reported that the prevalence of clinically significant SARS-CoV-2 cardiac involvement in collegiate athletes is <1% when assessed via transthoracic echocardiography in early stages (∼10 days post SARS-CoV-2 diagnosis) [Citation1]. Clark et al. (2021) supported this finding and reported ∼2.5% of professional athletes with COVID-19 exhibited cardiac injury in the early stages of infection [Citation18]. Within that study, COVID-19 positive athletes all had normal troponin and left ventricular strain imaging, with only one athlete meeting the cardiac magnetic resonance (CMR) criteria for myocarditis. Although no athletes in the current study presented with myocarditis, we recently published a case series of cardiac abnormalities in athletes after COVID-19 infection. All four athletes had an abnormal left ventricular global longitudinal strain, and one presented with recent myocarditis [Citation19]. Moreover, a recent study investigated twenty-six COVID-19 positive student-athletes and reported that none of the athletes had markers of elevated troponin, but 46% showed the presence of CMR-indicated late gadolinium enhancement – an imaging technique used to assess myocardial injury clinically [Citation20]. Additionally, athletes with persistent symptoms had no SARS-CoV-2 sequelae following evaluations. Still, within athletes who had chest pain upon return to exercise, 20.8% of cases had probable or definite SARS-CoV-2 cardiac involvement on CMR [Citation7]. Others have reported that ∼40% of COVID-19 athletes exhibit late pericardial enhancement, with over half of athletes showing subclinical myocardial and pericardial disease [Citation21].
Limited prospective data exist evaluating sex-specific differences in cardiac involvement among athletes following diagnosis and quarantine for respiratory infections like SARS-CoV-2. We observed a significantly lower but clinically normal LVEF in female and male athletes during the convalescent phase of recovery compared to matched controls. Moreover, this finding was dependent on the timing of imaging relative to diagnosis, with only those athletes imaged within 27 days of diagnosis having a significantly lower LVEF. The similar response between females and males for LVEF was mirrored by most echocardiographic-derived variables, with minor discrepancies between sexes. Moreover, neither female nor male athletes exhibited any previously identified ‘red-flags’ for SARS-CoV-2 cardiac injury. Like the present study, in a cohort of 100 patients recovering from SARS-CoV-2 infection, Puntmann et al. (2020) [Citation22] reported relatively mild decreases in LVEF compared to healthy controls but within standard criteria. However, this is not a consistent finding, with numerous reports of an unchanged LVEF following SARS-CoV-2 infection in the acute recovery and convalescent phases [Citation23, Citation24]. An essential consideration in the present study is the initial training status of collegiate athletes. Although we did not assess cardiorespiratory fitness, all recruited athletes were actively training or competing in their respective sports.
Unfortunately, we could not assess global left ventricular strain and strain rate with myocardial work analysis, an emerging method incorporating left ventricular afterload into global longitudinal strain analysis [Citation25]. While LVEF is the classical index for evaluating LV function, it often lacks sensitivity is influenced by ventricular loading (e.g. afterload) and LV geometry, factors that have been previously reviewed [Citation26]. Therefore, to mitigate this limitation, we also measured peak LV systolic velocity via tissue Doppler as a secondary qualitative assessment of LV function [Citation27]. Notably, peak systolic LV tissue velocity was not different within both male and female athlete groups. Therefore, coupled with the preserved stroke volume and LVEF within the normal range for this population, these findings suggest that LV systolic function was relatively normal following infection and quarantine. A second important limitation is that we had no pre-infection cardiac volumes/function measurements in these athletes. This limitation precludes us from determining if the lower LVEF is new, possibly due to direct SARS-CoV-2 involvement or an indirect consequence of the SARS-CoV-2 quarantine protocol restricting exercise training.
The minor changes observed in the present study may be due to alterations in training status that occur with strict quarantine protocols. It is well established that removal or reduction in exercise volume can result in loss of athletic cardiac adaptations related to regular training. During data collection (August 2020–May 2021), a positive COVID-19 diagnosis resulted in a 10–14-day isolation period with cessation of exercise training, which would result in an acute detraining phase in this highly trained group of athletes [Citation28]. In a recent review of detraining effects on cardiac structure and function in athletes, reduction in LV wall thickness and chamber dimensions are experienced within the first couple weeks of detraining [Citation29]. However, there is limited evidence of exercise detraining studies eliciting reductions in LVEF. Thus, the minor reductions in LVEF we see in SARS-CoV-2 positive athletes compared to controls may be a combination of both the infection and detraining effects.
The sex-specific alterations observed in the present study, due to SARS-CoV-2 infection and detraining, provide additional insight into the sex-based differences in cardiovascular structure and outcomes in athletes. As recently reviewed, sex-specific remodeling with exercise detaining is inconclusive due to the relative paucity of females in published work, particularly female collegiate athletes [Citation29]. However, the cardiac adaption to detraining, like exercise training, may be influenced by physiological and morphological distinctions between males and females [Citation29,Citation30]. Extreme detraining via strict bed rest has been shown to elicit similar reductions in left ventricular mass in men and women [Citation31]; however, this may not translate to short-duration reduced sport-specific training loads. Interestingly, a recent study of men and women showed a weak association between plasma volume and ejection fraction following prescribed detraining [Citation5]. Unfortunately, the changes in blood/plasma volume were not assessed in the present study, and a similar relationship could not be assessed. Some studies suggest that blood volume changes with physical deconditioning may be different between men and women [Citation32], which may partially explain the sex-specific observations in the present study. It remains unclear if the different adaptations to exercise training for men and women are mirrored in the detraining period. As reviewed by Finocchiaro et al. [Citation33] and Petek et al. [Citation34], female athletes engaged in dynamic-type sports primarily exhibit eccentric LV remodeling compared to the concentric LV remodeling observed in men engaged in similar sports. This varied type of remodeling to exercise training may partially explain the decreased IVSDd, and LVEDD observed in the male participants in the present study. Despite the current paucity of information on sex-specific exercise detraining, particularly with concomitant respiratory infection, the present study supports the ongoing work that sex influences cardiac adaptation. Moreover, this work supports the need for additional work determining the impact of sex on cardiovascular adaptations to exercise.
The present study is unique in that various athletes across multiple sports were imaged, resulting in potential variations in the level of intensity of dynamic and static exercise required for sport completion that may influence exercise-induced cardiac remodeling. Sports that are predominantly strength-specific will result in concentric hypertrophy in which the athlete experiences an increase in LV mass and wall thickness, while sports that require more endurance training will result in eccentric hypertrophy, which consists of enlargement in the atria along with dilation of both ventricles [Citation35]. These sport-specific cardiac changes can also vary depending on the athlete’s sex, as females in dynamic sports have a higher prevalence of eccentric left ventricular hypertrophy, while males in dynamic sports tend to display concentric LV remodeling [Citation33]. Notably, most athletes in the present study performed mixed exercise-type sports. Using recently published classification resulted in 92% of athletes engaged in moderate/high dynamic and 85% in low/moderate static intensity levels [Citation30]. However, this is only an estimate as many athletes were engaged in various levels of strength, power, and endurance training separate from their specific sports competition. As such, the mean average absolute RWT in the present study was 0.43 ± 0.08, suggesting a similar type of cardiac remodeling across our study sample [Citation33].
Notably, the current study’s findings could extend beyond SARS-CoV-2 infections and into other viral infections that lead to time off from training. Respiratory tract infections, including the flu, respiratory syncytial virus, and COVID-19, are the most common type of illness in athletes [Citation11], with elite athletes having a higher incidence than recreationally competitive athletes and normally active individuals [Citation36]. Recent work by Derman et al. (2022) further revealed that a pooled incidence of multiple types of acute respiratory infections reported upwards of 4.9 infections per 1000 athlete days [Citation12], potentially impacting athlete health and performance. The present study highlights how both direct and indirect effects of infection can result in alterations in left ventricular structure and function. While the mild differences observed in the SARS-CoV-2 athletes here were still within clinically normal limits, they may negatively affect exercise performance. Thus, the athlete, coaching, strength and conditioning staff, athletic training, and medical personnel should consider these changes when making decisions and setting expectations regarding a return to full training and competition. Moreover, given that cardiac images were obtained on average 40 days post-infection (and thus 26–30 days post-quarantine), the present study suggests that several weeks may be required to recover full cardiac function following reduced LVEF following SARS-CoV-2 infection and detraining during quarantine.
Limitations
This study presents a few notable limitations. First, this study was completed in a single-center setting, which may limit generalizing the observations to other institutions or athletic populations. Secondly, the time duration of training status for each student-athlete was not recorded; thus, we cannot report potential differences following varying years of participation in their respective sport. Additionally, we did not have pre-SARS-CoV-2 infection measurements, limiting our ability to evaluate any causal relationships surrounding the indirect effects of quarantine detraining or direct effects of SARS-CoV-2 infection on cardiac function. Thus, our findings represent associations, and we cannot determine causality. Our data were also collected before any vaccines were available; thus, all student-athletes were unvaccinated, and we cannot provide insight into the potential efficacious effects of vaccination status. Similarly, we did not assess cardiorespiratory fitness throughout SARS-CoV-2 recovery. Given the potential for cardiorespiratory detraining, which can occur rapidly within a few days of inactivity [Citation37], we cannot delineate the true etiology of the observed cardiac differences following SARS-CoV-2.
An important experimental consideration of the present study is the limited ability to assess differences between athletes with and without symptoms during their SARS-CoV-2 infection. Less than half of the study sample self-reported mild non-specific symptoms (e.g. cough, sore throat, nasal congestion) during the time of infection and thus limited sample size and ability to directly assess differences based on the type of symptoms reported. Also, limited information on the duration of symptoms was available. This is, however, an essential factor to consider, given that 2 weeks of convalescence without resumption of exercise after symptom resolution has been recommended. Therefore, the duration of quarantine and reduced training load may have varied between athletes and introduced additional variability in our study outcomes.
Conclusions
We present data from a cohort of collegiate athletes during the convalescent phase of SARS-CoV-2 infection who underwent a 10–14-day quarantine period with restricted exercise training. We observed a relatively mild lower, but clinically normal, LVEF between SARS-CoV-2-positive athletes and non-SARS-CoV-2 controls, with no athletes exhibiting clinically defined pathologic SARS-CoV-2 cardiac involvement. Importantly, these differences in LVEF were only observed in athletes imaged within the first few weeks (<27 days) of infection. Athletes imaged ≥27 days post-infection (i.e. longer convalescent period), no difference in LVEF was observed compared to matched controls. These findings are consistent with other studies showing minimal cardiac injury during COVID-19 recovery and suggest that indirect effects of cardiovascular detraining during quarantine may have occurred. However, future studies are warranted to determine if the lower LVEF observed in SARS-CoV-2 infected and quarantined athletes truly impacts performance and recovery duration.
Authors Contributors
CJA, KVG, and VRGT Conceptualized and designed the study. VRGT, SKP, STH, ZJW, PJT, CH, and CJA contributed substantially to data acquisition. VRGT, and CJA performed the data analysis. VRGT and CJA drafted the manuscript. VRGT, SKP, STH, ZJW, PJT, CH, JAD, and CJA critically revised the manuscript. All authors provided final approval of the version to be submitted and agreed to be accountable for all aspects of the work in ensuring that questions related to the accuracy or integrity of any part of the work are appropriately investigated and resolved.
Abbreviations | ||
ACE2 | = | Angiotensin-converting enzyme 2 |
CMR | = | cardiac magnetic resonance imaging |
e’ | = | Tissue-Doppler myocardial early filling |
IVSDd | = | Intraventricular septal diastolic diameter |
LVEDD | = | LV end-diastolic diameter |
LVEDDi | = | Left ventricular end-diastolic diameter index |
LVEF | = | Left ventricular ejection fraction |
PCR | = | Polymerase chain reaction |
PEF | = | Peak expiratory flow |
R | = | Resistance |
s’ | = | Tissue-Doppler myocardial systolic |
SARS-CoV-2 | = | severe acute respiratory syndrome coronavirus 2 |
TMPRSS2 | = | Transmembrane serine protease 2 |
TTE | = | Transthoracic echocardiography |
Disclosure statement
No potential conflict of interest was reported by the author(s).
Data availability statement
Data are available on reasonable request. All data relevant to the study are included in the article. In addition, the data supporting this study’s findings are available from the corresponding author upon reasonable request.
Additional information
Funding
References
- Moulson N, Petek BJ, Drezner JA, et al. SARS-CoV-2 cardiac involvement in young competitive athletes. Circulation. 2021;144(4):1–10. doi:10.1161/CIRCULATIONAHA.121.054824.
- Phelan D, Kim JH, Elliott MD, et al. Screening of potential cardiac involvement in competitive athletes recovering from COVID-19: an expert consensus statement. JACC Cardiovasc Imaging. 2020;13(12):2635–2652. doi:10.1016/j.jcmg.2020.10.005.
- Martinez MW, Tucker AM, Bloom OJ, et al. Prevalence of inflammatory heart disease among professional athletes with prior COVID-19 infection who received systematic return-to-Play cardiac screening. JAMA Cardiol. 2021;6(7):745–752. doi:10.1001/jamacardio.2021.0565.
- Neufer PD. The effect of detraining and reduced training on the physiological adaptations to aerobic exercise training. Sports Med. 1989;8(5):302–320. doi:10.2165/00007256-198908050-00004.
- Pedlar CR, Brown MG, Shave RE, et al. Cardiovascular response to prescribed detraining among recreational athletes. J Appl Physiol (1985). 2018;124(4):813–820. doi:10.1152/japplphysiol.00911.2017.
- Bruinvels G, Lewis NA, Blagrove RC, et al. COVID-19-Considerations for the female athlete. Front Sports Act Living. 2021;3:606799. doi:10.3389/fspor.2021.606799.
- Petek BJ, Moulson N, Baggish AL, et al. Prevalence and clinical implications of persistent or exertional cardiopulmonary symptoms following SARS-CoV-2 infection in 3597 collegiate athletes: a study from the outcomes registry for cardiac conditions in athletes (ORCCA). Br J Sports Med. 2022;56(16):913–918. doi:10.1136/bjsports-2021-104644.
- Valtonen M, Waris M, Vuorinen T, et al. Common cold in team Finland during 2018 winter olympic games (PyeongChang): epidemiology, diagnosis including molecular point-of-care testing (POCT) and treatment. Br J Sports Med. 2019;53(17):1093–1098. doi:10.1136/bjsports-2018-100487.
- Schwellnus M, Adami PE, Bougault V, et al. International olympic committee (IOC) consensus statement on acute respiratory illness in athletes part 1: acute respiratory infections. Br J Sports Med. 2022. doi:10.1136/bjsports-2022-105759.
- Pedersen L, Lindberg J, Lind RR, et al. Reopening elite sport during the COVID-19 pandemic: experiences from a controlled return to elite football in Denmark. Scand J Med Sci Sports. 2021;31(4):936–939. doi:10.1111/sms.13915.
- Meyer T, Mack D, Donde K, et al. Successful return to professional men’s football (soccer) competition after the COVID-19 shutdown: a cohort study in the german bundesliga. Br J Sports Med. 2021;55(1):62–66. doi:10.1136/bjsports-2020-103150.
- Derman W, Badenhorst M, Eken MM, et al. Incidence of acute respiratory illnesses in athletes: a systematic review and meta-analysis by a subgroup of the IOC consensus on ‘acute respiratory illness in the athlete. Br J Sports Med. 2022;56(11):630–638. doi:10.1136/bjsports-2021-104737.
- Hosey RG, Rodenberg RE. Training room management of medical conditions: infectious diseases. Clin Sports Med. 2005;24(3):477–506, vii. doi:10.1016/j.csm.2005.03.003.
- Hennessy S, Bilker WB, Berlin JA, et al. Factors influencing the optimal control-to-case ratio in matched case-control studies. Am J Epidemiol. 1999;149(2):195–197. doi:10.1093/oxfordjournals.aje.a009786.
- Phelan D, Kim JH, Chung EH. A game plan for the resumption of sport and exercise after coronavirus disease 2019 (COVID-19) infection. JAMA Cardiol. 2020;5(10):1085–1086. doi:10.1001/jamacardio.2020.2136.
- Mitchell C, Rahko PS, Blauwet LA, et al. Guidelines for performing a comprehensive transthoracic echocardiographic examination in adults: recommendations from the American society of echocardiography. J Am Soc Echocardiogr. 2019;32(1):1–64. doi:10.1016/j.echo.2018.06.004.
- Smith RL, Gibson LL, Martinez PP, et al. Longitudinal assessment of diagnostic test performance over the course of acute SARS-CoV-2 infection. J Infect Dis. 2021;224(6):976–982. doi:10.1093/infdis/jiab337.
- Clark DE, Parikh A, Dendy JM, et al. COVID-19 myocardial pathology evaluation in athletes with cardiac magnetic resonance (COMPETE CMR). Circulation. 2021;143(6):609–612. doi:10.1161/CIRCULATIONAHA.120.052573.
- Turpin VG, et al. A case series of diverse cardiac abnormalities in collegiate athlete with COVID-19: role for multimodality imaging. Case Rep Cardiol. 2022;2022:3259978.
- Rajpal S, Tong MS, Borchers J, et al. Cardiovascular magnetic resonance findings in competitive athletes recovering from COVID-19 infection. JAMA Cardiol. 2021;6(1):116–118. doi:10.1001/jamacardio.2020.4916.
- Brito D, et al. High prevalence of pericardial involvement in college student athletes recovering from COVID-19. JACC Cardiovasc Imaging. 2020;14(3):541–555.
- Puntmann VO, Carerj ML, Wieters I, et al. Outcomes of cardiovascular magnetic resonance imaging in patients recently recovered from coronavirus disease 2019 (COVID-19). JAMA Cardiol. 2020;5(11):1265–1273. doi:10.1001/jamacardio.2020.3557.
- Churchill TW, Bertrand PB, Bernard S, et al. Echocardiographic features of COVID-19 illness and association with cardiac biomarkers. J Am Soc Echocardiogr. 2020;33(8):1053–1054. doi:10.1016/j.echo.2020.05.028.
- Inciardi RM, Adamo M, Lupi L, et al. Characteristics and outcomes of patients hospitalized for COVID-19 and cardiac disease in Northern Italy. Eur Heart J. 2020;41(19):1821–1829. doi:10.1093/eurheartj/ehaa388.
- Marzlin N, Hays AG, Peters M, et al. Myocardial work in echocardiography. Circ Cardiovasc Imaging. 2023;16(2):e014419.
- Marwick TH. Ejection fraction pros and cons: JACC state-of-the-Art review. J Am Coll Cardiol. 2018;72(19):2360–2379. doi:10.1016/j.jacc.2018.08.2162.
- Yu C-M, Sanderson JE, Marwick TH, et al. Tissue doppler imaging a new prognosticator for cardiovascular diseases. J Am Coll Cardiol. 2007;49(19):1903–1914. doi:10.1016/j.jacc.2007.01.078.
- Martin WH, Coyle EF, Bloomfield SA, 3rd, et al. Effects of physical deconditioning after intense endurance training on left ventricular dimensions and stroke volume. J Am Coll Cardiol. 1986;7(5):982–989., doi:10.1016/s0735-1097(86)80215-7.
- Petek BJ, Groezinger EY, Pedlar CR, et al. Cardiac effects of detraining in athletes: a narrative review. Ann Phys Rehabil Med. 2022;65(4):101581. doi:10.1016/j.rehab.2021.101581.
- Martinez MW, Kim JH, Shah AB, et al. Exercise-Induced cardiovascular adaptations and approach to exercise and cardiovascular disease: JACC state-of-the-Art review. J Am Coll Cardiol. 2021;78(14):1453–1470. doi:10.1016/j.jacc.2021.08.003.
- Dorfman TA, Levine BD, Tillery T, et al. Cardiac atrophy in women following bed rest. J Appl Physiol. 2007;103(1):8–16. doi:10.1152/japplphysiol.01162.2006.
- Fortney SM, Turner C, Steinmann L, et al. Blood volume responses of men and women to bed rest. J Clin Pharmacol. 1994;34(5):434–439. doi:10.1002/j.1552-4604.1994.tb04984.x.
- Finocchiaro G, Dhutia H, D’Silva A, et al. Effect of sex and sporting discipline on LV adaptation to exercise. JACC Cardiovasc Imaging. 2017;10(9):965–972. doi:10.1016/j.jcmg.2016.08.011.
- Petek BJ, Chung EH, Kim JH, et al. Impact of sex on cardiovascular adaptations to exercise: JACC review topic of the week. J Am Coll Cardiol. 2023;82(10):1030–1038. doi:10.1016/j.jacc.2023.05.070.
- Baggish AL, Wang F, Weiner RB, et al. Training-specific changes in cardiac structure and function: a prospective and longitudinal assessment of competitive athletes. J Appl Physiol (1985). 2008;104(4):1121–1128. doi:10.1152/japplphysiol.01170.2007.
- Spence L, Brown WJ, Pyne DB, et al. Incidence, etiology, and symptomatology of upper respiratory illness in elite athletes. Med Sci Sports Exerc. 2007;39(4):577–586. doi:10.1249/mss.0b013e31802e851a.
- Ade CJ, Broxterman RM, Barstow TJ. VO(2max) and microgravity exposure: convective versus diffusive O(2) transport. Med Sci Sports Exerc. 2015;47(7):1351–1361. doi:10.1249/MSS.0000000000000557.