Abstract
LA-ICPMS U–Pb data from metamorphic monazite in upper amphibolite and granulite-grade metasedimentary rocks indicate that the Nawa Domain of the northern Gawler Craton in southern Australia underwent multiple high-grade metamorphic events in the Late Paleoproterozoic and Early Mesoproterozoic. Five of the six samples investigated here record metamorphic monazite growth during the period 1730–1690 Ma, coincident with the Kimban Orogeny, which shaped the crustal architecture of the southeastern Gawler Craton. Combined with existing detrital zircon U–Pb data, the metamorphic monazite ages constrain deposition of the northern Gawler metasedimentary protoliths to the interval ca 1750–1720 Ma. The new age data highlight the craton-wide nature of the 1730–1690 Ma Kimban Orogeny in the Gawler Craton. In the Mabel Creek Ridge region of the Nawa Domain, rocks metamorphosed during the Kimban Orogeny were reworked during the Kararan Orogeny (1570–1555 Ma). The obtained Kararan Orogeny monazite ages are within uncertainty of ca 1590–1575 Ma zircon U–Pb metamorphic ages from the Mt Woods Domain in the central-eastern Gawler Craton, which indicate that high-grade metamorphism and associated deformation were coeval with the craton-scale Hiltaba magmatic event. The timing of this deformation, and the implied compressional vector, is similar to the latter stages of the Olarian Orogeny in the adjacent Curnamona Province and appears to be part of a westward migration in the timing of deformation and metamorphism in the southern Australian Proterozoic over the interval 1600–1545 Ma. This pattern of westward-shifting tectonism is defined by the Olarian Orogeny (1600–1585 Ma, Curnamona Province), Mt Woods deformation (1590–1575 Ma), Mabel Creek Ridge deformation (1570–1555 Ma, Kararan Orogeny) and Fowler Domain deformation (1555–1545 Ma, Kararan Orogeny). This westward migration of deformation suggests the existence of a large evolving tectonic system that encompassed the emplacement of the voluminous Hiltaba Suite and associated volcanic and mineral systems.
Introduction
Footnote∗Reconstructions of continental terrains attempt to integrate all available geological and geophysical datasets into a coherent best-fit model. Reconstruction models for Proterozoic Australia commonly focus on the interaction of the Gawler Craton with the North Australian Craton (as defined by Myers et al. Citation1996) and the Musgrave Province (Myers et al. Citation1996; Karlstrom et al. Citation2001; Betts et al. Citation2002; Dawson et al. Citation2002; Giles et al. Citation2002, Citation2004; Fitzsimons Citation2003; Betts & Giles Citation2006; Schmidt et al. Citation2006; Wade et al. Citation2006) (). However, while these models have progressed understanding of the processes that led to the formation of Proterozoic Australia, such reconstructions are hampered by the lack of geological constraints in key regions. One such region is the northern Gawler Craton (), which comprises the link between the Late Archean–Early Paleoproterozoic core of the Gawler Craton and the Mesoproterozoic Musgrave Province. This geographical position makes the northern Gawler Craton of paramount importance for understanding the interaction of the Gawler Craton, North Australian Craton and Musgrave Province during the Proterozoic. However, despite the apparent importance of the northern Gawler Craton crust for understanding the building of Proterozoic Australia, very little is known about the geology of the region and timing of the events that have shaped that crust. This is primarily due to the almost complete lack of outcropping basement rocks (Daly et al. Citation1998; Payne et al. Citation2006a), and thickness of cover sequences (up to 2 km) in a greenfields region which has resulted in a relative lack of explorative drilling to date.
Figure 1 Current distribution of tectonic elements of Proterozoic Australia that formed prior to ca 1070 Ma. Constructed from GIS datasets of Northern Territory Geological Survey, Geological Survey of Western Australia, Primary Industries and Resources South Australia, Geological Survey of Queensland. Australian coast outline adapted from topographic dataset of Geoscience Australia. Gawler Craton outline and detail interpreted from geophysical datasets (Fairclough et al. Citation2003).
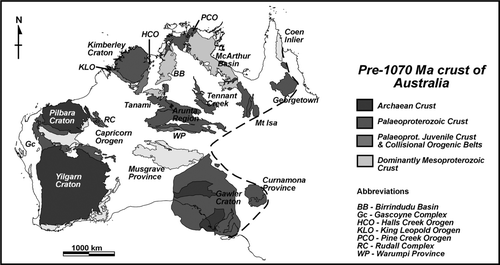
Figure 2 Simplified solid geology of the Gawler Craton (after Fairclough et al. Citation2003). Inset, Total Magnetic Intensity image of the Gawler Craton overlain by interpreted domains with selected domain names: P & D, Peake and Denison Domain; CP, Coober Pedy Domain; MW, Mt Woods Domain.
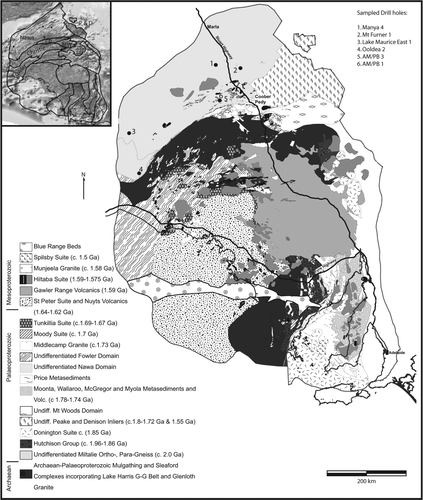
This study utilises U–Pb LA-ICPMS (Laser Ablation Inductively Coupled Plasma Mass Spectrometry) monazite geochronology on samples obtained from existing drillcore to constrain the timing of metamorphism in the northern Gawler Craton. These new geochronological data are linked to petrological observations and the pre-existing framework for metamorphism and tectonism on the Gawler Craton. The results allow for discussion on the nature and areal extent of orogenic events on the Gawler Craton and the implications for the evolution of the Australian crust during the Proterozoic.
Geological Setting
The Gawler Craton () is composed of Late Archean–Early Paleoproterozoic supracrustal and magmatic lithologies which are surrounded, overlain and intruded by Paleoproterozoic (1850–1610 Ma) and Mesoproterozoic (1590–1510 Ma) rocks (Daly et al. Citation1998; Ferris et al. Citation2002; Swain et al. Citation2005a; Fanning et al. Citation2007). Neoproterozoic and Phanerozoic sedimentary rocks and sediments overlie much of the craton. Tectonic domains () have been delineated for the Gawler Craton based on the interpretation of total magnetic intensity (TMI) and gravity datasets and limited geological evidence. These domains are primarily based on structural trends evident in the geophysical data and do not necessarily represent fundamental terrane boundaries. The dominant structure evident in the TMI data () is the Karari Fault Zone, which bounds the Coober Pedy Domain (, ), and separates the Paleoproterozoic rocks of the Nawa and Peake and Denison Domains (, ) (Payne et al. Citation2006a) from the Archean rocks of the Christie Domain and Paleoproterozoic to Mesoproterozoic Mt Woods Domain ().
Figure 3 Total Magnetic Intensity image of the Gawler Craton overlain with distribution of Kimban Orogeny metamorphic and magmatic ages on the Gawler Craton. Dashed lines represent interpreted domains with the Coober Pedy Domain (CP), Mabel Creek Ridge (MC), Peake and Denison Domain (P & D) and Mt Woods Domain (MW) and trace of the Karari Fault Zone (KFZ, bold line) marked. Metamorphic ages (black dots) are: 1, 1731 ± 8 Ma (U–Pb SHRIMP zircon: Vassallo 2001); 2, 1699 ± 15 Ma (MSWD 7.3, U–Pb SHRIMP zircon: Vassallo 2001); 3, 1693 ± 9 (MSWD 8.5, U–Pb SHRIMP zircon: Vassallo 2001); 4, 1701 ± 2.8 Ma (U–Pb zircon: Vassallo 2001); 5, 1712 ± 10 Ma (U–Pb SHRIMP monazite: Teasdale 1997); 6, 1736 ± 14 Ma (U–Pb SHRIMP zircon Daly et al. 1998); 7, 1696 ± 12 Ma (U–Pb SHRIMP zircon, 4 analyses: Daly et al. 1998); 8, 1728 ± 30 Ma (Pb–Pb garnet: Hopper 2001); 9–13, Kimban Orogeny metamorphic ages from this study; 14, 1728 ± 13 Ma (U–Pb SHRIMP monazite: Dutch et al. 2006); 15, 1720 ± 6 Ma (U–Pb SHRIMP monazite: Dutch et al. 2006). White dots represent the location of dated syn-orogenic Kimban granites; white squares represent the location of dated post-orogenic Tunkillia Suite granites: granite data from Teasdale (1997), Daly et al. (1998) and Ferris (2001).
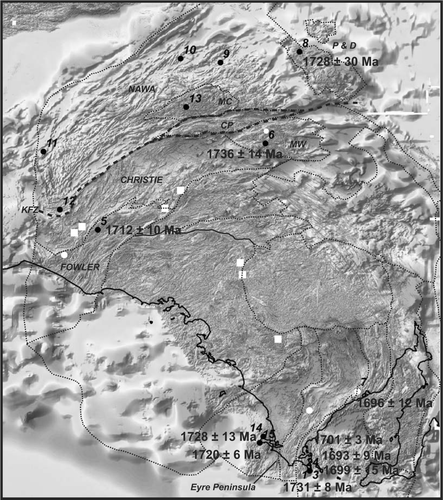
Current understanding of the evolution of the Gawler Craton is largely based on the geological record preserved in the southeastern and central-southern parts of the craton. The Late Archean stratigraphy in the central Gawler Craton consists of metasedimentary, volcanic and granite–greenstone lithologies (ca 2560–2500 Ma: Swain et al. 2005a) that were deformed during the Sleafordian Orogeny (2460–2430 Ma: Daly et al. Citation1998; McFarlane Citation2006). This was followed by a period of tectonic quiescence until approximately 2020–2000 Ma, at which time the protoliths of the Miltalie Gneiss were intruded (Daly et al. Citation1998; Howard Citation2006; Fanning et al. Citation2007). A period of sediment deposition (Hutchison Group) in the eastern Gawler Craton (Daly et al. Citation1998; Fanning et al. Citation2007) was followed by the Cornian Orogeny and Donington Suite intrusion (ca 1850 Ma: Reid et al. Citation2006). This event marked the onset of a period of extensive sediment deposition across the eastern and northern Gawler Craton (Daly et al. Citation1998; Payne et al. Citation2006a), before onset of magmatism, deformation and metamorphism of the Kimban Orogeny during ca 1730–1690 Ma (Daly et al. Citation1998; Vassallo & Wilson Citation2001, Citation2002; Ferris et al. Citation2002; Swain et al. Citation2005b). The Kimban Orogeny was followed by the late to post-tectonic magmatism of the Tunkillia Suite (1690–1670 Ma: Ferris Citation2001; Payne et al. Citation2006b) and graben development at ca 1650 Ma (Tarcoola Formation: Daly et al. Citation1998). This was followed shortly thereafter by the development of arc-like magmatism of the St Peter Suite between 1630 and 1615 Ma, which dominates the southwest part of the craton (Swain et al. in press) and extensive I- and A-type magmatism of the Gawler Range Volcanics (ca 1592 Ma) and Hiltaba Suite (1595–1575 Ma: Creaser Citation1995; Morrow & McPhie Citation2000; Allen et al. Citation2003).
Existing geochronology in the northern and western Gawler Craton is mostly restricted to the vicinity of the Karari Fault Zone and the shear zones of the Fowler Domain (, ), and appears to define at least three high-grade metamorphic/thermal events. Metamorphic ages consistent with the Kimban Orogeny are reported from the Fowler Domain (, ) (U–Pb SHRIMP zircon, 1712 ± 10 Ma: Teasdale 1997) and Peake and Denison Domain (, ) (Hopper Citation2001). In addition to these age constraints, a Pb–Pb TIMS zircon age (Kober method) of 1690 ± 9 Ma and a SHRIMP U-Pb zircon age of 1659 ± 6 Ma were obtained from ultra-high-temperature (UHT) metamorphic rocks in the Ooldea 2 drillhole in the southwestern Nawa Domain () (Teasdale 1997; Fanning et al. Citation2007). Teasdale (Citation1997) assigned partial melting and granulite-grade metamorphism of the Ooldea 2 rocks to the ca 1690 Ma zircon-forming event (producing granoblastic textures), and assigned the overprinting sapphirine–sillimanite–orthopyroxene–quartz-bearing UHT assemblages to the ca 1660 Ma age. The ca 1690 Ma age correlates with the age range described for the Kimban Orogeny (Ferris et al. Citation2002). The ca 1660 Ma age is similar to EPMA monazite ages obtained by Swain et al. (Citation2005b) from drillholes close to Ooldea 2.
Metamorphic zircon U–Pb SHRIMP ages constrain the next major phase of metamorphism in the northern-central and western Gawler Craton to ca 1550 Ma. This is constrained by an age of 1547 ± 9 Ma in the Fowler Domain (Daly et al. Citation1998; Fanning et al. Citation2007) and was formerly constrained by ages of ca 1550 Ma in the Mabel Creek Ridge area (Nawa Domain: Daly et al. Citation1998) and 1565 ± 8 Ma in the Coober Pedy Domain (Daly et al. Citation1998). However, publication and revision of data for the latter two ages by Fanning et al. (Citation2007) have demonstrated that these ages were based upon four or fewer analyses for each sample and do not form coherent age clusters (Fanning et al. Citation2007).
Swain et al. (Citation2005b) reported EPMA monazite ages from shear zones in the Fowler and Christie Domains that are younger than ages reported elsewhere for tectonism in the Gawler Craton. These range between ca 1516 and 1468 Ma. A recent study by Fraser & Lyons (2006) has provided a range of 40Ar/39Ar ages from across the northern and western Gawler Craton that highlight regional cooling at ca 1450 Ma. Fraser & Lyons (Citation2006) suggested that significant movement occurred along shear zones (notably Karari, Tallacootra and Coorabie: ) at this time, leading to the current crustal exposure levels and domain geometries.
Relative to the existing geochronological database, the present study significantly expands the areal extent of samples that constrain the timing of tectonism in the northern Gawler Craton ().
Sampling and Methods
Due to the paucity of outcrop in the northern and northwestern Gawler Craton, all samples used in this study were taken from diamond drillholes. Despite the areal extent of the northern Gawler Craton, there are only 17 diamond drillholes with basement intersections, and samples obtained from these holes form the basis of this study. Samples used in this study are listed in , and the location of the drillholes is shown in . All drillholes intersect basement lithologies of the Nawa Domain (), with AM/PB 1 and AM/PB 3 intersecting basement in the Mabel Creek Ridge subdivision of the Nawa Domain (). The majority of diamond drillholes sampled are either diamond tails on reverse-circulation drillholes or small (<10 m) intersections of basement in stratigraphic drillholes through the overlying basin sequences.
Table 1 Sample descriptions and drillhole locations.
Single-grain U–Pb monazite dating was undertaken using LA-ICPMS at the University of Adelaide similar to the method used for zircon in Payne et al. (2006a). Monazite was separated from the samples through routine crushing, sieving, panning, Frantz Isodynamic separation, and heavy-liquid separation. Monazite grains were imaged using BSE on a Phillips XL-20 and XL-30 SEM. U–Pb isotopic analyses were obtained using a New Wave 213 nm Nd-YAG laser in a He ablation atmosphere, coupled to an Agilent 7500cs ICP-MS. A 60 s gas blank was analysed followed by 60 s of measurement during monazite ablation. Prior to each ablation, the laser was fired for 10 s with the shutter closed to allow beam stabilisation. The beam diameter was ∼15 μm at the sample surface. Isotopes measured were 204Pb, 206Pb, 207Pb and 238U for 10, 15, 30 and 15 ms, respectively. Common-Pb was not corrected for in the age calculations due to unresolvable, varying levels of 204Hg interference on the 204Pb isotope peak. Instead, monitoring of the 204Pb isotope allowed for analyses to be omitted if appreciable common-Pb was observed during analysis.
Monazite age calculations utilised the real-time correction program ‘Glitter’ developed at Macquarie University, Sydney (Jackson et al. Citation2004). U–Pb fractionation was corrected using the MAdel monazite standard (TIMS normalisation data: 207Pb/206Pb = 490.7 Ma, 206Pb/238U = 514.8 Ma and 207Pb/235U = 510.4 Ma). Corrected age accuracy was confirmed prior to unknown analysis and throughout analysis runs by regular analyses of an in-house monazite standard (94-222/Bruna-NW, ca 450 Ma: Maidment Citation2005). The MAdel standard contains two ages separated by approximately 4 million years (Appendix 1). The dominant age domain analysed in this study is the younger domain with a 207Pb/235U age of 510 Ma. This age variation (4 million years) is well within the uncertainty of the methodology and so is not considered to affect age data. To further ensure against inaccurate age reporting, an overestimate of 1% uncertainty is assigned to the age of the standard for sample age error calculations, and samples are analysed over multiple analytical sessions. Over the duration of this study, and in the month prior to analysis, the reported average normalised ages for MAdel are 513.9 ± 1.6 and 511.0 ± 1.3 Ma for the 206Pb/238U and 207Pb/235U ages, respectively (n = 57). This provides an indicator of the expected minor/insignificant age variation obtained using the MAdel standard and typical accuracy of the LA-ICPMS technique.
Sample Description
Mt Furner 1: central-northern Nawa Domain
The Mt Furner 1 well bore is 555.04 m in depth, primarily intersecting Cambrian sequences of the Officer Basin. Basement intersected at a depth of 548.64 m consists of layered metasedimentary lithologies that define a total intersection of 6.6 m. Detrital zircon ages indicate that the precursors to the metasedimentary rocks have a maximum depositional age of ca 1740 Ma (Payne et al. 2006a).
The sampled interval consists of a garnet-bearing metapelite with a steeply dipping foliation. Garnet porphyroblasts are up to 3–4 mm in diameter and contain inclusions of sillimanite, biotite and quartz. The garnets are enclosed by a gneissic fabric defined by biotite which is interspersed in a matrix of plagioclase and quartz. The primary gneissic fabric has undergone within-plane reworking resulting in recrystallisation of biotite, quartz and plagioclase. Late-stage muscovite (up to 1.5 mm) post-dates both structural fabrics.
Manya 4: central-northern Nawa Domain
The Manya 4 drillhole depth is 806.71 m, most of which penetrates Cambrian to Neoproterozoic sequences of the eastern Officer Basin. The bottom 10.6 m intersects the gneissic basement to the Officer Basin. Detrital zircon ages indicate that the precursors to the metasedimentary rocks have a maximum depositional age of ca 1750 Ma (J. L. Payne unpubl. data).
The sampled interval is a partly weathered garnet–biotite-bearing quartzo-feldspathic gneiss. Garnet ranges between 0.5 and 2 mm, and contains inclusions of biotite and quartz. The gneissic fabric is defined by coarse biotite interspersed with quartz and sericitised plagioclase.
Lake Maurice East 1: far western Nawa Domain
The Lake Maurice East drillhole is 738.94 m in depth and penetrates a thick package of Lower to Middle Cambrian sandstone of the Officer Basin, with metapelitic basement intersected at a depth of 691 m. Samples from Lake Maurice East 1 exhibit significant mineral breakdown with feldspars and interpreted cordierite largely replaced by sericite. However, despite the extent of the replacement, the pseudomorphic nature of the alteration has left the rock texture intact. The primary mineral assemblage comprised coarse-grained garnet, sillimanite, cordierite, biotite, plagioclase and quartz. Very little cordierite is preserved, but its former abundance is indicated by pseudomorphs composed of fine-grained sericite and chlorite (). These pseudomorphs are distinct from largely replaced plagioclase, which displays feldspar-cleavage patterns despite the extent of alteration. Sillimanite has also undergone extensive weathering and is preserved as coarse-grained crystals up to 5 mm in length. Garnet occurs as relatively inclusion-free blasts up to 5 mm in diameter, and also as symplectitic intergrowth with magnetite (). However, both textural types appear to have formed part of the primary assemblage. The primary coarse-grained mineral assemblage is overprinted by a strong mylonitic fabric that encloses garnet and sillimanite porphyroclasts. Tails on asymmetric cordierite porphyroclasts define the shear fabric along with quartz and plagioclase.
Figure 4 Metamorphic mineral assemblages. (a) Mt Furner 1, showing sillimanite inclusions in garnet and late coarse-grained muscovite growth. (b) Manya 4, garnet, biotite and quartz with weathering products. (c) Lake Maurice East 1, garnet, magnetite, sillimanite with quartz ribbons. Alteration products are pseudomorphing plagioclase and cordierite. (d) Lake Maurice East 1, garnet and magnetite symplectite textures. (e) Ooldea 2, garnet and magnetite symplectite. (f) Enhanced photograph of Ooldea 2 drillcore demonstrating UHT fine-grained mylonitic fabric (taken from Teasdale 1997). (g) AM/PB 3, breakdown texture of garnet to orthopyroxene. (h) AM/PB 3, fine-grained shear fabric overprinting primary coarse-grained fabric. (i) AM/PB 1, coarse-grained garnet showing sillimanite inclusions paralleling the curved foliation enclosing the garnet in the matrix. (j) AM/PB 1, coarse-grained cordierite enclosed by quartz and sillimanite. bi, biotite; gt, garnet; mag, magnetite; mus, muscovite; opx, orthopyroxene; plg, plagioclase; qtz, quartz; sill, sillimanite; cd, cordierite; (cd), clay minerals after cordierite; (plg), clay minerals after plagioclase; wth, indeterminate weathering products.
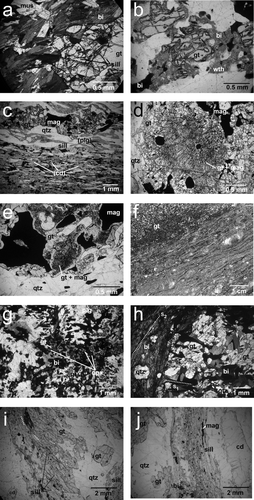
Ooldea 2: southwestern Nawa Domain
The Ooldea 2 drillhole penetrates magnetite-bearing metasedimentary rocks, including aluminous pelitic granulites, to a depth of 169 m. High-grade metamorphism has reset detrital zircons (Teasdale Citation1997) such that a maximum deposition age cannot be obtained for these rocks. Despite this, Sm–Nd isotopic data suggest the lithologies have a similar provenance and potentially deposition age to lithologies found in other drillholes in the northern Gawler Craton (Payne et al. Citation2006a).
The following brief description summarises the work of Teasdale (1997) that incorporates a detailed petrographical study of the Ooldea 2 granulites. Quartz-bearing granulites in Ooldea 2 contain three stages of mineral assemblage development. The first stage is defined by preserved coarse-grained intergrowths of prismatic sillimanite and magnetite, hypersthene, garnet coronas on magnetite and garnet–magnetite symplectites (). This coarse-grained assemblage is overprinted by a fine-grained, high-strain fabric () associated with the development of: (i) hypersthene, sillimanite and quartz intergrowths interpreted to have replaced primary cordierite; and (ii) hypersthene, sapphirine, K-feldspar, plagioclase and quartz intergrowths interpreted to have replaced after osumilite. The final stage of mineral growth includes the development of retrograde biotite and cordierite after anhydrous phases (sapphirine and hypersthene). Teasdale (1997) interpreted the P–T–t evolution to follow an anticlockwise path with the second mineral assemblage defining UHT metamorphism with conditions in excess of ∼900°C and 950 MPa.
AM/PB 3: Mabel Creek Ridge area of Nawa Domain
The AM/PB 3 drillhole intersects basement at a depth of 187.25 m and has a total depth of 287 m. The basement intersection contains significant intervals of iron-rich metasedimentary units that are characterised by high percentages of magnetite. Detrital zircon ages indicate that the precursors to the metasedimentary rocks have a maximum depositional age of ca 1740 Ma (Payne et al. Citation2006a).
The sampled interval targeted comparatively magnetite-poor rocks and contained evidence for two metamorphic assemblages. The primary mineral assemblage is characterised by interlayered, steeply dipping coarse-grained garnet–biotite–plagioclase–quartz and garnet–hornblende–biotite–plagioclase–quartz assemblages. In both bulk compositions, garnet has been partially replaced by orthopyroxene and plagioclase (). The primary assemblage and garnet replacement textures have been overprinted by narrow shear zones that formed at a high angle to the primary fabric. The shear fabric assemblage is dominated by biotite (), which encloses recrystallised plagioclase and quartz.
AM/PB 1: Mabel Creek Ridge area of Nawa Domain
The AM/PB 1 drillhole intersects magnetite-rich metasedimentary rocks at 152 m before passing into pelitic metasedimentary rocks ∼30 m above the maximum depth of 356.2 m. Samples for this study are taken from the pelitic interval and contain a single metamorphic mineral assemblage characterised by coarse-grained garnet (up to 5 cm), sillimanite (2–4 mm), biotite, cordierite (3–8 mm), quartz, magnetite and minor plagioclase. Garnet contains inclusions of quartz, K-feldspar and sillimanite. Garnet is enclosed by a gneissic foliation but also overprints the foliation as evidenced by sillimanite and biotite inclusions that parallel the matrix foliation (). Cordierite is generally enclosed by quartz and sillimanite, and is rarely found in contact with garnet, biotite or magnetite (). No retrogression of this mineral assemblage is evident.
Monazite U–Pb Geochronology
Data for all monazite analyses are presented in Appendix 2∗. Backscattered electron (BSE) images of the analysed monazite grains are displayed in , and weighted average and concordia ages are shown with quoted errors at 95% confidence levels in .
Figure 5 BSE images of representative monazites from each sample analysed. (a) Mt Furner 1. (b) Manya 4. (c) Lake Maurice East 1: gr 1, resorption textures; gr 2, concentric zoning. (d) Lake Maurice East 1, resorption textures (e) Ooldea 2. (f) AM/PB 3, monazite with ca 1555 Ma rim. (g) AM/PB 3, monazite with inconsistent BSE zoning and ages. (h) AM/PB 1, monazite from garnet separate with two growth zones evident, highlighted by dashed white line.
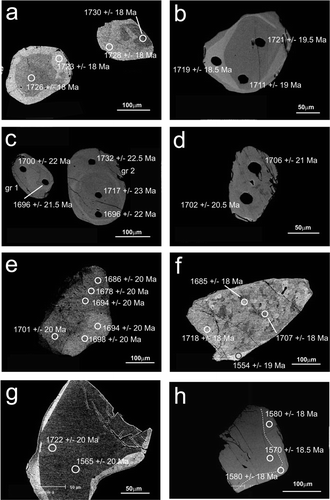
Mt Furner 1: sample 650698
Nine monazites were analysed from sample 650698 for a total of 12 analyses. Two compositional zones () are evident in BSE images with the inner (darker BSE response) growth zone being overgrown by the later zone (lighter BSE response), or in some instances resorption is evident. No correlation is evident between U–Pb age and compositional zoning for this sample (, ). U–Pb and 207Pb/206Pb age data demonstrate a unimodal age distribution (). Weighted average analyses of 207Pb/206Pb age data yield an age of 1728 ± 10 Ma (MSWD = 0.19). The calculated concordia age is 1727.3 ± 6.6 Ma (MSWDconc&equiv = 1.3).
Figure 6 Concordia, weighted average and probability density plots for all data from analysed samples. (a, b) Mt Furner: (a) concordia; (b) 207Pb/206Pb weighted average. (c, d) Manya 4: (c) concordia; (d) 207Pb/206Pb weighted average. (e, f) Lake Maurice East: (e) concordia; (f) 207Pb/206Pb weighted average. (g, h) Ooldea 2: (g) concordia; (h) 207Pb/206Pb weighted average. (i–k) AM/PB3: (i) concordia, all data; (j) 207Pb/206Pb weighted average, old group; (k) 207Pb/206Pb weighted average, young group. (l–n) AM/PB1: (l) concordia bulk-rock monazites and garnet-separate monazites (grey ellipses); (m) 207Pb/206Pb weighted average, garnet-separate monazites; (n) 207Pb/206Pb weighted average, bulk-rock monazites excluding monazite grain displaying two simple growth zones in BSE imaging; 207Pb/206Pb weighted average for all analyses is 1569 ± 8 Ma.
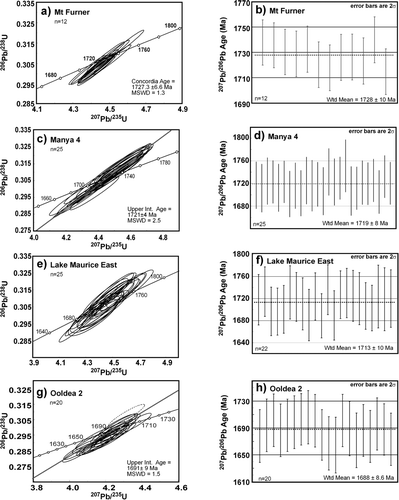
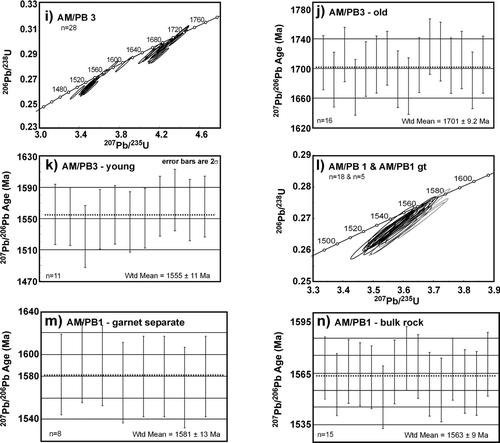
Manya 4: sample 650703
Nine monazites were analysed from sample 650703 for a total of 25 analyses. Only simple compositional zoning is evident in BSE images consistent with monazite growth (). The age data obtained for the sample are unimodal, and hence no distinguishable age variation is seen relating to compositional zoning. A weighted average 207Pb/206Pb age of 1719.3 ± 7.9 Ma (MSWD = 0.3) is obtained. The U–Pb concordia upper intercept age is 1721 ± 4 Ma (MSWD = 2.5) (Figure ).
Lake Maurice East 1: sample 1052049
Ten monazites were analysed from sample 1052049 for a total of 23 spot analyses. Analysis LME10.b contained minor common-Pb, evidenced by a small elevation of 204Pb counts per second during analysis, and hence is excluded from age calculations. Monazites are characterised by concentric compositional zoning () or zoning consistent with resorption (, ). Monazite grains with concentric zoning display a range of ages (1733–1689 Ma), while monazite grains displaying resorption characteristics yield ages in the range of 1706–1696 Ma. Ages obtained from grains with concentric zoning do not display consistent age and zoning correlations. All 207Pb/206Pb ages are within error, and a weighted average of all valid 207Pb/206Pb data (n = 22, excluding LME10.b) produces an age of 1713 ± 9.5 (MSWD = 0.47) (). The limited range of younger ages obtained from grains showing resorption characteristics in BSE imaging and the non-equivalence of U–Pb data in concordia age calculations, despite all data plotting on or within error of concordia (), suggest a sequence of monazite growth and resetting events. However, the current age data do not allow for resolution of any such events.
Ooldea 2: sample 660856
Seven monazite grains were analysed from the Ooldea 2 sample for a total of 21 analyses (Appendix 2∗). Monazites analysed show variable BSE responses that include grains with no visible zoning to grains that show up to three well-defined compositional zones (). No consistent relationship is apparent between obtained ages and BSE zoning. A weighted average of all 207Pb/206Pb data provides a mean age of 1686 ± 8.4 Ma (MSWD = 0.56). A weighted average of 207Pb/206Pb data, excluding analysis Ooldm-3a () on the basis of reverse discordance due to potential excess 206Pb (see below), provides a mean age of 1688 ± 8.6 Ma (MSWD = 0.41) (). A Model 2 upper intercept age of 1688.6 ± 8.8 Ma (MSWD = 1.6) is obtained for all U–Pb data. An intercept age of 1691 ± 9.5 Ma (MSWD = 1.5) is obtained for U–Pb data when excluding the outlying data point above the concordia (denoted in ). The outlying analysis falls outside the main cluster on a U–Pb concordia diagram and has a 207Pb/206Pb age of 1651 ± 19 Ma. Analysis Ooldm-3a has a similar 207Pb/235U age to concordant data of the dataset but has a significantly higher 206Pb/238U age/ratio. This is reflected in the statistical rejection of the 206Pb/238U age from a weighted average of all 206Pb/238U age data but no rejection of the 207Pb/235U age from equivalent calculations (using Isoplot 3.0: Ludwig 2003). Due to the potential for excess 206Pb due to incorporation of 230Th in monazite, this analysis is not considered reliable despite being statistically inseparable based upon 207Pb/206Pb ages. Although little difference is observed in final calculated ages, the ages calculated excluding analysis Ooldm-3a are preferred.
AM/PB 3: sample 650723
Twelve monazites from sample 650723 were analysed for a total of 28 spot analyses. BSE images demonstrate the highly variable nature of the monazite grains (). demonstrates evidence for two monazite populations in this sample. Individual monazite grains contain one or two ages with variable relationships between age and composition zones in BSE images (, g). A simple weighted average of all 207Pb/206Pb ages in the older grouping yields an age of 1701 ± 9.2 Ma (n = 16, MSWD = 0.76). demonstrates that analyses incorporated in this age group display an apparent bimodal age distribution in U–Pb data. No systematic correlation is evident between age and monazite compositional zoning for the older event. This variation in data may reflect the effects of Pb-loss related to the younger event that has drawn data down along the concordia, and hence the age of 1701 ± 9.2 Ma can be interpreted as a minimum age. The second event is represented by a weighted average 207Pb/206Pb age of 1555 ± 11 Ma (n = 11, MSWD = 0.4). This age is obtained from single-age grains and outer rims on monazite grains containing ca 1730–1690 Ma ages (). The single analysis with a near-concordant age between the two major groupings ) is interpreted to represent either a mixed age analysis or possible draw-down of U–Pb data along a discord due to partial Pb-loss at ca 1555 Ma and hence is not included in the age calculations for the younger group.
AM/PB 1: sample 967234
Monazites for sample 967234 were separated from a bulk-rock sample split and also from within three large garnet grains (3–5 cm across). Three monazites were analysed from the garnet separate for a total of five analyses. BSE images of these grains display two compositional zones in each, consistent with monazite growth. A weighted average of these five analyses yields an age of 1584 ± 16 Ma (MSWD = 0.18).
Six grains were analysed from the bulk-rock separate for a total of 18 analyses. Five grains display various growth and resorption characteristics and one grain displays two simple compositional zones consistent with monazite growth (). A weighted average of all 207Pb/206Pb age data for the bulk-rock monazite sample yields an age of 1565.6 ± 8.5 Ma (n = 18, MSWD = 0.19). The monazite grain with two growth zones from the bulk-rock sample displays similar growth zoning to the monazite grains separated from within the garnets. A weighted average of the data from this grain provides a 207Pb/206Pb age of 1577 ± 21 Ma (n = 3, MSWD = 0.11). A weighted average of all data from sample 967234 provides a mean 207Pb/206Pb age of 1569 ± 8 Ma (n = 23, MSWD = 0.35). Petrologically constrained geochronology is required to confirm the possibility of two monazite growth events as suggested by the different, but within uncertainty, ages obtained from the garnet and bulk-rock monazite separates. The weighted mean age of 1569 ± 8 Ma is considered the age of monazite growth for this sample.
Discussion
The following discussion focuses on the implications of the results for the timing and nature of metamorphism in the northern Gawler Craton and the evolution of the Gawler Craton as a whole. These new constraints are then discussed with respect to the various reconstruction models of Proterozoic Australia that incorporate the Gawler Craton.
Interpretation of U–Pb monazite ages
The samples used in this study contain metamorphic mineral assemblages ranging in grade from upper amphibolite to UHT granulite. Under the P–T conditions experienced by these samples, monazite generally records metamorphic ages (Parrish Citation1990; Rubatto et al. Citation2001; Kelsey et al. Citation2006). Traditionally, monazite U–Pb ages in high-T rocks have been interpreted to reflect cooling through closure temperatures of around 700°C (Dahl Citation1997). However, Pb-diffusion data (Foster et al. Citation2002; Seydoux-Guillaume et al. Citation2002) suggest that monazite has closure systematics similar to zircon, and therefore U–Pb ages from monazite should record monazite growth under high-T conditions. That monazite ages are commonly younger than zircon ages in high-T metamorphic rocks (Rubatto et al. Citation2001; Williams Citation2001) is therefore likely to reflect growth systematics rather than lower-T Pb diffusion (Fitzsimons et al. Citation2005; Kelsey et al. Citation2006). Therefore, in the following discussion, the monazite ages obtained in this study are considered to be indicative of growth during high-grade metamorphism.
The new geochronological data can be summarised as defining events at ca 1725–1690 Ma and ca 1570–1555 Ma (AM/PB 1 and AM/PB 3). The earlier event correlates with previous geochronological constraints on the Kimban Orogeny (Teasdale Citation1997; Daly et al. Citation1998; Ferris et al. Citation2002), which have been obtained predominantly from the southeastern Gawler Craton. The metamorphic ages also provide tight constraints on the depositional interval for the sedimentary precursors. Detrital zircon ages obtained from the same drillholes give maximum depositional ages of around 1740 Ma (Payne et al. Citation2006a), and in combination with the monazite ages presented here indicate that deposition occurred in the interval ca 1740–1720 Ma.
The ages obtained from Ooldea 2, Lake Maurice East 1 and AM/PB 3 are late in the interval typically attributed to the Kimban Orogeny (Daly et al. Citation1998). However, there is insufficient coverage of drillholes and existing geochronological data to interpret whether this represents spatial variations in the timing of the Kimban event across the craton, or reflects the paucity of comparative monazite data.
The U–Pb data obtained from Lake Maurice East 1 show a spread of ages ranging from ca 1735 to ca 1690 Ma. Rocks in Lake Maurice East 1 show two distinct stages of metamorphic development suggesting the possibility of two monazite-forming events during the 1735–1690 Ma interval. An early coarse-grained garnet–cordierite–sillimanite–biotite-bearing assemblage has been overprinted by a fine-grained granulite-grade fabric. It is beyond the capabilities of the current geochronology to assign an age to each of these metamorphic mineral assemblages, but presumably they occurred within age uncertainty of each other during the Kimban Orogeny.
The results from sample 660856 (Ooldea 2) hold particular significance for understanding the timing of high-T metamorphic events in the northern and western Gawler Craton. Rocks from Ooldea 2 contain petrological evidence for two prograde rock-forming metamorphic assemblages, an interpreted early coarse-grained garnet–cordierite–quartz–osumilite–magnetite-bearing association, and a secondary orthopyroxene–sillimanite–sapphirine–quartz mylonite fabric (Teasdale 1997). Previous zircon geochronology has yielded two interpreted ages of metamorphism from this drillhole: 1690 ± 9 Ma and 1659 ± 6 Ma (Teasdale Citation1997; Daly et al. Citation1998; Fanning et al. Citation2007). The older of these results is essentially identical to the age of 1686 ± 8 Ma obtained from this study. The absence of monazite ages correlating with the 1659 Ma zircon age is curious, as monazite will typically grow at lower P–T conditions than zircon (Rubatto et al. Citation2001; Williams Citation2001; Kelsey et al. Citation2006). Therefore, if the rocks in Ooldea 2 have undergone two high-grade recrystallisation events, it would be likely that monazite would either record the younger event or show a range of partially reset ages between ca 1690 and 1650 Ma.
Despite the high-T mineral assemblages associated with the secondary assemblages in Ooldea 2, there is little evidence for migmatisation, suggesting that the bulk composition was effectively anhydrous during the metamorphic reworking. Kelsey et al. (Citation2006) showed that monazite in anhydrous rocks (with REE-rich bulk compositions) is stable at temperatures in excess of 850°C. This is consistent with the presence of inherited monazite ages in dry UHT metasedimentary granulites (Kelsey Citation2008). In interpreting the significance of the ca 1690 and 1650 Ma zircon ages from Ooldea 2, it should be noted that geochemical data are not available for the interval that yielded the 1659 ± 6 Ma age, and therefore it may be from a reactive bulk composition in which zircon was able to recrystallise. Therefore, a reasonable interpretation is that the age of the early granulite assemblage in Ooldea 2 is ca 1690 Ma. This event effectively dehydrated the rock mass and limited the extent of partial melting during the second event, assisting in the preservation of monazite and zircon in most bulk compositions. In this case the younger high-T reworking event may be ca 1660 Ma in age and be recorded by zircon recrystallisation in a reactive bulk composition.
The younger age population obtained from AM/PB 1 and AM/PB 3 defines an age of 1570–1555 Ma. This age is within error of existing zircon U–Pb geochronology obtained from granulite-grade metasediments in the Nundroo area of the Fowler Domain in the southwestern Gawler Craton (Daly et al. Citation1998). The nomenclature for this orogenic event is currently not agreed upon (Daly et al. Citation1998; Ferris et al. Citation2002; Direen et al. Citation2005). The initial nomenclature of Daly et al. (Citation1998) referred to this event as the Late Kararan Orogeny, with the Early Kararan referring to tectonism at around 1650 Ma. The 1650 Ma event has been renamed the Ooldean Event (Direen et al. Citation2005) in recognition that metamorphism around 1660 Ma in age is recorded only in the Ooldea 2 drillhole (Daly et al. Citation1998). Direen et al. (Citation2005) named an event the Coorabie Orogeny based upon the apparent timing of movement on the Coorabie Shear Zone and other shear zones in the western Gawler Craton. Direen et al. (Citation2005) assigned a duration of ∼100 million years (1550–1450 Ma) for this tectonism. There is currently no evidence to support a single continuous orogenic event of this duration, and furthermore the nature of events during this period are poorly constrained. However, rather than introduce yet another event name, we suggest the terminology Kararan Event be used for tectonothermal activity in the period ca 1570–1545 Ma in the Gawler Craton.
Tectonothermal events in the Gawler Craton
The Gawler Craton contains evidence for multiple major deformational events that span the interval ca 2500–1450 Ma, as well as several craton-scale magmatic-dominated events () (Daly et al. 1998; Swain et al. Citation2005b; Fraser & Lyons Citation2006). Of these events, the 1730–1690 Ma Kimban Orogeny appears to be the most areally extensive with high-T metamorphism, deformation and magmatism affecting much of the craton.
Table 2 Summary of orogenic events in the Gawler Craton.
Figures and display U–Pb geochronological constraints that provide evidence for the extent of Kimban-aged tectonism in the Gawler Craton. In the eastern and southeastern Gawler Craton, including the Mt Woods, and Peake and Dension Domains (), Kimban-aged metamorphism ranges from lower amphibolite to medium-pressure granulite in grade (Vassallo & Wilson Citation2001; Tong et al. Citation2004; Dutch et al. Citation2006). In the samples analysed in this study from the northern and western parts of the craton, the mineral assemblages are consistent with upper amphibolite to granulite grade metamorphism. This demonstrates the Kimban Orogeny to be a high-grade event present in all Paleoproterozoic regions of the Gawler Craton ().
Figure 7 (a) TMI data of the Gawler Craton overlain with locations of Kimban Orogeny metamorphic age data. Black dots represent metamorphic ages; white dots and squares represent syn- and post-Kimban magmatism. Dashed trendlines represent structural trends interpreted to have formed during the Kimban Orogeny. (b) TMI data of Gawler Craton overlain with location and ages of metamorphic mineral geochronology in the ca 1585–1545 Ma interval. Black dots represent U–Pb metamorphic ages in the interval 1590–1570 Ma; White dots represent U–Pb metamorphic ages in the 1570–1550 Ma interval; the white square represents metamorphic age in the 1550–1540 Ma interval. See text for data sources.
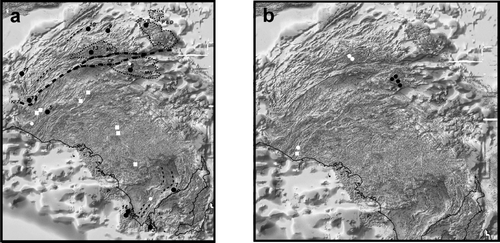
In the southeastern part of the craton, Kimban-aged structures formed during dextral transpression (Vassallo & Wilson Citation2001, Citation2002; Dutch et al. Citation2006) and show a pronounced curvature from northeast to north trends moving northward along the orogen (), until they become obscured by the ca 1595 Ma Gawler Range Volcanics. This north–south trend is continued in the Peake and Denison Domain in which the earliest fabric and structure development, attributed to the Kimban Orogeny, has a north–south to southwest–northeast trend (Hopper 2001). Elsewhere in the northern Gawler Craton, there is no outcrop that allows direct dating of the northeast-trending structures that dominate the regional TMI data. However, the monazite ages obtained from the Manya 4, Mt Furner 1 and Lake Maurice East 1 drillholes provide evidence for Kimban Orogeny-aged (1730–1690 Ma) mineral growth and, at face value, suggest that the regional northeast-trending structures in the northern and northwestern Gawler Craton are Kimban in age. The Mabel Creek Ridge () preserves geochronological evidence for granulite-grade metamorphism at ca 1570–1550 Ma (Kararan Orogeny: Daly et al. Citation1998; this study). The Mabel Creek Ridge is typified by east to east-northeast structural trends in TMI datasets, and, together with the Coober Pedy Domain, is bounded by terrain-scale shear zones that form part of the Karari Shear system. The east and northeast structural trends that are evident in regional TMI datasets from the Coober Pedy Domain and Mabel Creek Ridge appear to continue eastward to the Peake and Denison Domain where large-scale east–west-trending open folds are visible in the TMI data. Hopper (Citation2001) demonstrated that these east–west folds were accompanied by lower-amphibolite-facies metamorphism in the Peake and Denison Domain and suggested they formed at around 1530 Ma based on unpublished Pb–Pb zircon data. We suggest that the Kararan Orogeny was responsible for the development of the east–west- to east-northeast-trending structures in the northern Gawler Craton. The age data from drillhole AM/PB 3 suggests that the Kararan Orogeny reworked rocks that had been previously affected by the Kimban Orogeny.
The significance of the ca 1660 Ma Ooldean Event is unclear. Metamorphism of this age is only seen in the Ooldea region in the far-western part of the craton. However, metamorphism reached UHT grade with the development of sapphirine–orthopyroxene–sillimanite–quartz-bearing granulites (Teasdale Citation1997), suggesting that the Ooldean Event was a significant phase of tectonism. Until a greater areal extent can be established, the context of this event in the evolution of the Gawler Craton is unknown. The age of the Ooldean Event is essentially identical to the timing of minor felsic volcanism and graben formation in the central Gawler Craton (Daly et al. 1998), which may be indicative of a widespread high-T regime.
The 1570–1555 Ma ages obtained from the Mabel Creek Ridge are partly within error of the timing of high-grade metamorphism and deformation in the Mt Woods Domain in the central-northeastern Gawler Craton. In the Mt Woods Domain, upper high-grade metasedimentary rocks yield metamorphic zircon ages in the range 1587 ± 2 to 1576 ± 5 Ma (Skirrow et al. Citation2006). At least some of these metasedimentary packages have maximum depositional ages around 1670–1650 (Skirrow et al. Citation2006), indicating that they post-date the Kimban Orogeny. The depositional and metamorphic ages are reminiscent of the upper parts of the Willyama Supergroup and its subsequent metamorphism in the adjacent Curnamona Province, hinting at correlation between these regions. The mineral assemblages in Mt Woods metasedimentary rocks include garnet–sillimanite–cordierite–biotite±spinel-bearing metapelites and garnet– orthopyroxene-bearing felsic gneiss (Flint & Benbow Citation1977), suggesting P–T conditions around 500–600 MPa and 750–800°C (Boger & White Citation2003; Kelsey et al. Citation2003). In the case of Mt Woods, the P–T regime is likely to have been augmented by the presence of mafic Hiltaba-aged rocks which form a significant component of the Mt Woods Domain. Although not specifically dated, fabrics which can now be attributed to the ca 1585 Ma granulite event trend approximately northeast reflecting compressional deformation (Betts et al. Citation2003). The timing of this deformation and the implied compressional vector is similar to the latter stages of the Olarian Orogeny in the adjacent Curnamona Province.
The generally slightly younger ages of 1570–1555 Ma obtained from the Mabel Creek Ridge and the 1545 Ma granulites in the Fowler Domain in the western Gawler Craton suggest that Kararan-aged deformation and metamorphism migrated southwestwards or westwards with time. If the Olarian Orogeny in the Curnamona Province is also considered in this context, the southern Australian Proterozoic appears to record a systematic westward migration in the locus of deformation from ca 1600 to ca 1545 Ma, with individual regions experiencing around a 10–15 million year interval of deformation (Olarian 1600–1585 Ma); Mt Woods (1590–1575 Ma); Mabel Creek Ridge (1570–1555 Ma); Fowler Domain (1555–1545 Ma) () (Teasdale Citation1997; Daly et al. Citation1998; Skirrow et al. Citation2006; Rutherford et al. Citation2007; this study). This apparent westward migration of deformation is at odds with the interpreted main compression direction of the Kararan Orogeny (north–south to northwest–southeast; see above). Further work is required to assess the apparent migration of deformation. A preliminary assessment may suggest there is a rotation of the bulk compressional stress field with time from north–south to east–west, as is suggested by late overprinting north–south-trending structures in the Olarian Orogeny (Rutherford et al. Citation2007).
The craton-wide extent of the Kimban-aged tectonism () supports the suggestion of Fraser & Lyons (Citation2006) that the Mesoproterozoic events on the Gawler Craton such as the Kararan Orogeny do not represent terrane juxtaposition, but rather are intracratonic reworking events. This is in contrast to the proposed Late Paleoproterozoic to Early Mesoproterozoic accretionary models of Daly et al. (Citation1998), Direen et al. (Citation2005) and Betts & Giles (Citation2006). The craton-wide nature of the Kimban Orogeny suggests that any terrane accretion in the Gawler Craton occurred prior to or during the Kimban Orogeny.
Implications for continental reconstruction models of Proterozoic Australia
The northern and western Gawler Craton is located in an important geographical position for models involving the reconstruction of Australia during the Proterozoic. The new geochronological data presented here, when combined with the 40Ar/39Ar data of Fraser & Lyons (Citation2006), place considerable constraints on models for the evolution of the Gawler Craton and Proterozoic Australia. As outlined above, models which call for the assembly of the Gawler Craton from distinct terranes (Daly et al. Citation1998; Dawson et al. Citation2002; Direen et al. Citation2005; Betts & Giles Citation2006) are not consistent with geochronological evidence, unless amalgamation occurred prior to, or during, the Kimban Orogeny.
It is apparent from the areal extent of structural, metamorphic and geochronological constraints on the Gawler Craton that any collision events leading to the assembly of the Gawler Craton must have occurred either during the Sleafordian Orogeny (2450–2430 Ma: Daly et al. 1998; McFarlane Citation2006) or Kimban Orogeny (1730–1690 Ma: Teasdale Citation1997; Vassallo & Wilson Citation2001, Citation2002; Ferris et al. Citation2002). The newly defined temporal and areal extent of the Kimban Orogeny further highlights the temporal correlations proposed between the Kimban Orogeny and Strangways Orogeny in the Arunta Region of the North Australian Craton (Karlstrom et al. Citation2001; Betts et al. Citation2002; Giles et al. Citation2002, Citation2004; Betts & Giles Citation2006). These links are based on temporal correlations, and further work is required to assess the structural and metamorphic characteristics (P–T–t paths) of the orogenies and determine if the proposed links are appropriate.
The significance of the Kararan Orogeny is currently poorly understood. The timing of Kararan deformation in part coincides with interpreted arc magmatism in the Musgrave Province (Wade et al. Citation2006) that lies to the north of Gawler Craton (). It also immediately post-dates proposed continental-scale, intracratonic orogeny associated with north–south shortening in the North Australian Craton (1590–1570 Ma: Hand Citation2006). Wade et al. (Citation2006) suggested that the Gawler Craton (as the leading edge of the Mawson Continent) collided with the North Australian Craton between ca 1590 and 1550 Ma. Wade et al. (Citation2006) also postulated that the suture is located at the northern margin of the Musgrave Province. If the 1565–1550 Ma Kararan-aged tectonism in the Gawler Craton is considered in the same context as the 1590–1570 Ma intracratonic deformation in the North Australian Craton and ca 1590–1550 Ma arc magmatism in the Musgrave Province (Hand 2006), essentially the entirety of the eastern Australian Proterozoic underwent approximately north–south shortening during the interval 1590–1570 Ma.
There is currently little evidence in the Gawler Craton for deformation after ca 1450 Ma (Fraser & Lyons Citation2006). This contradicts the recently proposed model of Schmidt et al. (2006), which proposes that the Gawler, North Australian and Yilgarn Cratons collided sometime between 1070 and 830 Ma in the region now comprising the Musgrave Block. The lack of deformation of this age in the northern Gawler Craton and the Musgrave Province, where there is extensive outcrop, also makes this model improbable.
Conclusions
New monazite LA-ICPMS U–Pb geochronology from the northern Gawler Craton has identified two regional high-grade metamorphic events. The earliest of these events occurred in the interval 1730–1690 Ma and affected metasedimentary rocks deposited between ca 1750 and 1730 Ma. The timing of this event is identical to the Kimban Orogeny, which dominates the crustal architecture of the southeastern Gawler Craton, and suggests that the Kimban Orogeny affected essentially the entire Gawler Craton. The continuity of Kimban-aged tectonism across the Gawler Craton suggests that any amalgamation events leading to the creation of the craton occurred either during or before 1730–1690 Ma.
Rocks affected by the Kimban Orogeny in the northern Gawler Craton underwent high-T reworking during the 1570–1555 Ma Kararan Orogeny. The effects of this event are best seen in the Coober Pedy Domain and Mabel Creek Ridge in the central-northern Gawler Craton where granulite-grade rocks have been exhumed along the craton-scale Karari Fault Zone and transported southward across Early Paleoproterozoic to Mesoproterozoic rocks comprising the central Gawler Craton. The timing of the Kararan Orogeny coincides with the final stages of arc magmatism in the Musgrave Province, which separates the Gawler Craton from the North Australian Craton. The Kararan Orogeny also overlaps with the waning stages of continental-scale intracratonic orogeny in the North Australian Craton. This suggests that it forms part of a continental-scale tectonic system that may have been linked to collision between the North Australian Craton and lithosphere that may have incorporated the Gawler Craton.
Acknowledgements
This research was funded by ARC Linkage Project LP0454301. Angus Netting is thanked for technical assistance. Anthony Reid is thanked for the many conversations regarding the 5% exposed Gawler Craton and together with Lachlan Rutherford is thanked for earlier reviews of the manuscript. Jim Crowley (MIT, now at Boise State University) is thanked for TIMS analyses on the MAdel monazite standard. Geoff Fraser and Ian Scrimgeour are thanked for thorough and detailed reviews which significantly improved the manuscript.
Notes
∗Appendix 2 [indicated by an asterisk (∗) in the text and listed at the end of the paper] is a Supplementary paper; copies may be obtaiked from the Geological Society of Australia's website (<http://www.gsa.org.au>) or from the National Library of Australia's Pandora archive (<http://nla.gov.au/nla.arc-25194>).
References
- Allen , S. R. , Simpson , C. J. , McPhie , J. and Daly , S. J. 2003 . Stratigraphy, distribution and geochemistry of widespread felsic volcanic units in the Mesoproterozoic Gawler Range Volcanics, South Australia . Australian Journal of Earth Sciences , 50 : 97 – 112 .
- Betts , P. G. and Giles , D. 2006 . The 1800–1100 Ma tectonic evolution of Australia . Precambrian Research , 144 : 92 – 125 .
- Betts , P. G. , Giles , D. , Lister , G. S. and Frick , L. R. 2002 . Evolution of the Australian lithosphere . Australian Journal of Earth Sciences , 49 : 661 – 695 .
- Betts , P. G. , Valenta , R. K. and Finlay , J. 2003 . Evolution of the Mount Woods Inlier, northern Gawler Craton, Southern Australia: an integrated structural and aeromagnetic analysis . Tectonophysics , 366 : 83 – 111 .
- Boger , S. D. and White , R. W. 2003 . The metamorphic evolution of metapelitic granulites from Radok Lake, northern Prince Charles Mountains, east Antarctica: evidence for an anticlockwise P–T path . Journal of Metamorphic Geology , 21 : 285 – 298 .
- Creaser , R. A. 1995 . Neodymium isotopic constraints for the origin of Mesoproterozoic felsic magmatism, Gawler Craton, South Australia . Canadian Journal of Earth Sciences , 32 : 460 – 471 .
- Dahl , P. S. 1997 . A crystal-chemical basis for Pb retention and fission-track annealing systematics in U-bearing minerals, with implications for geochronology . Earth and Planetary Science Letters , 150 : 277 – 290 .
- Daly , S. J. , Fanning , C. M. and Fairclough , M. C. 1998 . Tectonic evolution and exploration potential of the Gawler Craton, South Australia , Geology and Mineral Potential of Major Australian Mineral Provinces Edited by: Hodgson , I. and Hince , B. 145 – 168 . AGSO Journal of Australian Geology & Geophysics 17(3)
- Dawson , G. C. , Krapez , B. , Fletcher , I. R. , McNaughton , N. J. and Rasmussen , B. 2002 . Did late Palaeoproterozoic assembly of proto-Australia involve collision between the Pilbara, Yilgarn and Gawler cratons? Geochronological evidence from the Mount Barren Group in the Albany–Fraser Orogen of Western Australia . Precambrian Research , 118 : 195 – 220 .
- Direen , N. G. , Cadd , A. G. , Lyons , P. and Teasdale , J. 2005 . Architecture of Proterozoic shear zones in the Christie Domain, western Gawler Craton, Australia: geophysical appraisal of a poorly exposed orogenic terrane . Precambrian Research , 142 : 28 – 44 .
- Dutch , R. , Hand , M. and Kinny , P. D. 2006 . On the Kimban overprint: new geochronological constraints on high-grade metamorphism from Eyre Peninsula, southern Gawler Craton . Geological Society of Australia Abstracts , 82 (CD-ROM)
- Elburg , M. A. , Bons , P. D. , Foden , J. and Brugger , J. 2003 . A newly defined Late Ordovician magmatic–thermal event in the Mt Painter Province, northern Flinders Ranges, South Australia . Australian Journal of Earth Sciences , 50 : 611 – 631 .
- Fairclough , M. C. , Schwarz , M. and Ferris , G. M. 2003 . Interpreted Crystalline Basement Geology of the Gawler Craton, South Australia , (Special Map 1:1 000 000 scale) Primary Industries and Resources South Australia, Adelaide
- Fanning , C. M. , Reid , A. and Teale , G. S. 2007 . A geochronological framework for the Gawler Craton, South Australia . Geological Survey of South Australia Bulletin , 55
- Ferris , G. M. , Schwarz , M. and Heithersay , P. 2002 . “ The geological framework, distribution and controls of Fe-oxide and related alteration, and Cu–Au mineralisation in the Gawler Craton, South Australia. Part 1: Geological and tectonic framework ” . In Hydrothermal Iron Oxide Copper–Gold and Related Deposits: a Global Perspective , Edited by: Porter , T. Vol. 1 , 21 – 23 . Adelaide : PGC Publishing .
- Ferris , G. M. 2001 . The geology and geochemistry of granitoids in the Childara region, western Gawler Craton, South Australia: implications for the Proterozoic tectonic history of the western Gawler Craton and the development of the lode-style gold mineralisation at Tunkillia. MSc thesis, University of Tasmania, Hobart (unpubl.)
- Fitzsimons , I. C. W. 2003 . “ Proterozoic basement provinces of southern and southwestern Australia, and their correlation with Antarctica ” . In Proterozoic East Gondwana: Supercontinent Assembly and Breakup Edited by: Yoshida , M. , Windley , B. F. and Dasgupta , S . 93 – 130 . Geological Society of London Special Publication 206
- Fitzsimons , I. C. W. , Kinny , P. D. , Wetherley , S. and Hollingsworth , D. A. 2005 . Bulk chemical control on metamorphic monazite growth in pelitic schists and implications for U–Pb age data . Journal of Metamorphic Geology , 23 : 261 – 277 .
- Flint , R. B. and Benbow , M. C. 1977 . Geology of the Mt Woods Inlier . South Australian Department of Mines Report Book , : 77 – 134 .
- Foster , G. , Gibson , H. D. , Parrish , R. , Horstwood , M. , Fraser , J. and Tindle , A. 2002 . Textural, chemical and isotopic insights into the nature and behaviour of metamorphic monazite . Chemical Geology , 191 : 183 – 207 .
- Fraser , G. L. and Lyons , P. 2006 . Timing of Mesoproterozoic tectonic activity in the northwestern Gawler Craton constrained by 40Ar/39Ar geochronology . Precambrian Research , 151 : 160 – 184 .
- Giles , D. , Betts , P. and Lister , G. 2002 . Far-field continental backarc setting for the 1.80–1.67 Ga basins of northeastern Australia . Geology , 30 : 823 – 826 .
- Giles , D. , Betts , P. G. and Lister , G. S. 2004 . 1.8–1.5-Ga links between the North and South Australian Cratons and the Early–Middle Proterozoic configuration of Australia . Tectonophysics , 380 : 27 – 41 .
- Hand , M. 2006 . “ Intracratonic orogeny in Mesoproterozoic Australia ” . In Evolution and Metallogenesis of the North Australian Craton Edited by: Lyons , P. and Huston , D. L. Geoscience Australia Record 2006/16 (pdf)
- Hopper , D. J. 2001 . Crustal evolution of Paleo- to Mesoproterozoic rocks in the Peake and Denison Ranges, South Australia. PhD thesis, University of Queensland, Brisbane (unpubl.)
- Howard , K. E. 2006 . Provenance of Palaeoproterozoic metasedimentary rocks in the eastern Gawler Craton, Southern Australia: implications for reconstruction models of Proterozoic Australia. BSc (Hons) thesis, University of Adelaide, Adelaide (unpubl.)
- Jackson , S. E. , Pearson , N. J. , Griffin , W. L. and Belousova , E. A. 2004 . The application of laser ablation-inductively coupled plasma-mass spectrometry to in situ U–Pb zircon geochronology . Chemical Geology , 211 : 47 – 69 .
- Karlstrom , K. E. , Åhall , K-I. , Harlan , S. S. , Williams , M. L. , McLelland , J. and Geissman , J. W. 2001 . Long-lived (1.8–1.0 Ga) convergent orogen in southern Laurentia, its extensions to Australia and Baltica, and implications for refining Rodinia . Precambrian Research , 111 : 5 – 30 .
- Kelsey , D. E. 2008 . On Ultrahigh Temperature Crustal Metamorphism . Gondwana Research , 13 : 1 – 29 .
- Kelsey , D. , Clark , C. and Hand , M. 2006 . Integrating zircon and monazite saturation with metamorphism in melt-bearing systems . Geochimica et Cosmochimica Acta , 70 ( 18 Supplement 1 ) : A312
- Kelsey , D. E. , White , R. W. and Powell , R. 2003 . Orthopyroxene–sillimanite–quartz assemblages: distribution, petrology, quantitative P–T–X constraints and P–T paths . Journal of Metamorphic Geology , 21 : 439 – 453 .
- Ludwig K. R. 2003. Isoplot 3.00. Berkeley Geochronology Center Special Publication 4
- McFarlane , C. R. M. 2006 . Palaeoproterozoic evolution of the Challenger Au deposit, South Australia, from monazite geochronology . Journal of Metamorphic Geology , 24 : 75 – 87 .
- Maidment , D. 2005 . Palaeozoic high-grade metamorphism within the Centralian Superbasin, Harts Range region, central Australia. PhD thesis, Australian National University, Canberra (unpubl.)
- Morrow , N. and McPhie , J. 2000 . Mingled silicic lavas in the Mesoproterozoic Gawler Range Volcanics, South Australia . Journal of Volcanology and Geothermal Research , 96 : 1 – 13 .
- Myers , J. S. , Shaw , R. D. and Tyler , I. M. 1996 . Tectonic evolution of Proterozoic Australia . Tectonics , 15 : 1431 – 1446 .
- Parrish , R. R. 1990 . U–Pb dating of monazite and its application to geological problems . Canadian Journal of Earth Sciences , 27 : 1431 – 1450 .
- Payne , J. L. , Barovich , K. and Hand , M. 2006a . Provenance of metasedimentary rocks in the northern Gawler Craton, Australia: implications for Palaeoproterozoic reconstructions . Precambrian Research , 148 : 275 – 291 .
- Payne , J. L. , Barovich , K. M. and Hand , M. 2006b . On the tectonic setting of the magmatic suite previously known as the ‘Arc-like Tunkillia/Ifould.’ . Geological Society of Australia Abstracts , 82 (CD-ROM)
- Reid , A. , Hand , M. , Schwarz , M. , Belousova , E. A. and Pearson , N. J. 2006 . Palaeoproterozoic collisional tectonics in the southeastern Gawler Craton, South Australia . Geological Society of Australia Abstracts , 82 (CD-ROM)
- Rubatto , D. , Williams , I. S. and Buick , I. S. 2001 . Zircon and monazite response to prograde metamorphism in the Reynolds Range, central Australia . Contributions to Mineralogy and Petrology , 140 : 458 – 468 .
- Rutherford , L. , Hand , M. and Barovich , K. 2007 . Timing of Proterozoic metamorphism in the southern Curnamona Province: implications for tectonic models and continental reconstructions . Australian Journal of Earth Sciences , 54 : 65 – 81 .
- Schmidt , P. W. , Williams , G. E. , Camacho , A. and Lee , J. K. W. 2006 . Assembly of Proterozoic Australia: implications of a revised pole for the ?1070 Ma Alcurra Dyke Swarm, central Australia . Geophysical Journal International , 167 : 626 – 634 .
- Schoene , B. , Crowley , J. L. , Condon , D. J. , Schmitz , M. D. and Bowring , S. A. 2006 . Reassessing the uranium decay constants for geochronology using ID-TIMS U–Pb data . Geochimica et Cosmochimica Acta , 70 : 426 – 445 .
- Seydoux-Guillaume , A. M. , Paquette , J. L. , Wiedenbeck , M. , Montel , J. M. and Heinrich , W. 2002 . Experimental resetting of the U–Th–Pb systems in monazite . Chemical Geology , 191 : 165 – 181 .
- Skirrow , R. , Fairclough , M. , Budd , A. , Lyons , P. , Raymond , O. , Milligan , P. , Bastrakov , E. , Fraser , G. , Highet , L. , Holm , O. and Williams , N. 2006 . Iron oxide Cu–Au (–U) potential map of the Gawler Craton, South Australia 1:500 000 Scale , 1st edition Geoscience Australia, Canberra
- Swain , G. , Barovich , K. , Hand , M. and Schwarz , M. in press . Petrogenesis of the St Peter Suite, southern Australia: arc magmatism and Proterozoic crustal growth . Precambrian Research ,
- Swain , G. M. , Hand , M. , Teasdale , J. , Rutherford , L. and Clark , C. 2005b . Age constraints on terrane-scale shear zones in the Gawler Craton, southern Australia . Precambrian Research , 139 : 164 – 180 .
- Swain , G. , Woodhouse , A. , Hand , M. , Barovich , K. , Schwarz , M. and Fanning , C. M. 2005a . Provenance and tectonic development of the late Archaean Gawler Craton, Australia: U–Pb zircon, geochemical and Sm–Nd isotopic implications . Precambrian Research , 141 : 106 – 136 .
- Teasdale J. 1997. Methods for understanding poorly exposed terranes: the interpretive geology and tectonothermal evolution of the western Gawler Craton. PhD thesis, University of Adelaide, Adelaide (unpubl.)
- Tong , L. , Wilson , C. J. L. and Vassallo , J. J. 2004 . Metamorphic evolution and reworking of the Sleaford Complex metapelites in the southern Eyre Peninsula, South Australia . Australian Journal of Earth Sciences , 51 : 571 – 589 .
- Vassallo J. J. 2001. Tectonic investigation in the Gawler Craton. PhD thesis, University of Melbourne, Melbourne (unpubl.)
- Vassallo , J. J. and Wilson , C. J. L. 2001 . Structural repetition of the Hutchison Group metasediments, Eyre Peninsula, South Australia . Australian Journal of Earth Sciences , 48 : 331 – 345 .
- Vassallo , J. J. and Wilson , C. J. L. 2002 . Palaeoproterozoic regional-scale non-coaxial deformation; an example from eastern Eyre Peninsula, South Australia . Journal of Structural Geology , 24 : 1 – 24 .
- Wade , B. P. , Barovich , K. M. , Hand , M. , Scrimgeour , I. R. and Close , D. F. 2006 . Evidence for early Mesoproterozoic arc magmatism in the Musgrave Block, central Australia: implications for Proterozoic crustal growth and tectonic reconstructions of Australia . Journal of Geology , 114 : 43 – 63 .
- Williams , I. S. 2001 . Response of detrital zircon and monazite, and their U–Pb isotopic systems, to regional metamorphism and host-rock partial melting, Cooma Complex, southeastern Australia . Australian Journal of Earth Sciences , 48 : 557 – 580 .
APPENDIX 1: ISOTOPIC AND COMPOSITIONAL DATA FOR MAdel MONAZITE STANDARD
Monazite fragments for MAdel come from one large grain (∼3×1 cm) with average Th and U concentrations of 8.98 wt% and 0.43 wt%, respectively.
U–Pb isotope data for MAdel are provided in . TIMS analyses were conducted at Massachusetts Institute of Technology. The method involved is identical to that described in Schoene et al. (2006) for xenotime. In-house TIMS analysis methods follow those described for monazite in Elburg et al. (Citation2003).
Table 3 U – Pb Isotopic composition of MAdel by TIMS.
Representative trace element compositions of MAdel are provided in . Analysis was conducted using LA-ICPMS at the University of Adelaide. Ca concentrations used for internal standardisation were obtained using a Cameca SX-51 Microprobe.
Table 4 Representative compositions of MAdel monazite standard.
Supplementary Paper
APPENDIX 2: U–Pb MONAZITE GEOCHRONOLOGY DATA