Abstract
Metabolite lactic acid has always been regarded as a metabolic by-product rather than a bioactive molecule. Recently, this view has changed since it was discovered that lactic acid can be used as a signal molecule and has novel signal transduction functions both intracellular and extracellular, which can regulate key functions in the immune system. In recent years, more and more evidence has shown that lactic acid is closely related to the metabolism and polarization of macrophages. During inflammation, lactic acid is a regulator of macrophage metabolism, and it can prevent excessive inflammatory responses; In malignant tumors, lactic acid produced by tumor tissues promotes the polarization of tumor-associated macrophages, which in turn promotes tumor progression. In this review, we examined the relationship between lactic acid and macrophage metabolism. We further discussed how lactic acid plays a role in maintaining the homeostasis of macrophages, as well as the biology of macrophage polarization and the M1/M2 imbalance in human diseases. Potential methods to target lactic acid in the treatment of inflammation and cancer will also be discussed so as to provide new strategies for the treatment of diseases.
1. Introduction
Lactic acid is the end product of glycolysis, and its presence in mammals was first observed in muscle tissue in the early 19th century [Citation1]. Since its discovery, lactic acid has been widely studied and has been shown to play a role in many metabolic functions [Citation2,Citation3]. However, more and more studies have found that lactic acid plays different physiological roles as active signals in various biological processes, rather than just as a by-product of metabolism [Citation4]. Such processes include gene transcriptional regulation, signal transduction, functional phenotypic transformation of immune cells [Citation5], and even promoting the activity and proliferation of stem cells (such as hair follicle stem cells [Citation6], intestinal stem cells [Citation7,Citation8], cancer stem cells [Citation9], etc.). Lactic acid can also control muscle regeneration [Citation10] and promote wound healing [Citation11,Citation12], and plays a powerful role in regeneration. In inflammatory diseases, lactic acid plays an anti-inflammatory role through the lactic acid signaling pathway [Citation13]. In malignant tumors, continuous local release of lactic acid is associated with cancer progression [Citation13].
Macrophages are long-lived innate immune cells that can be found in almost all tissues. Macrophages have strong heterogeneity and plasticity [Citation14]. In many human diseases, macrophages are triggered into different phenotypes in response to infection, tumors, metabolism and immunity [Citation14]. Under physiological conditions, M1/M2 macrophages maintains an intermediate state, while a shift from M1 to M2 or vice versa leads to disease progression. This will be discussed in detail in a later section. Therefore, the phenotypic transformation of macrophages is a potential way to treat diseases [Citation15]. At present, studies have found that lactic acid played an important role in regulating the functional polarization of macrophages [Citation13]. Therefore, in this review, we focused on lactic acid in macrophage metabolic reprogramming and studied the biology of macrophage polarization, the M1/M2 imbalance in human diseases, and the role of lactic acid in macrophage homeostasis. Finally, we discussed how lactic acid drives macrophage polarization and potential treatment opportunities of lactic acid.
2. Lactic acid: a signaling molecule
Lactic acid, both as a nutrient and a hormone-like signaling molecule, has the following characteristics: (i)energy-rich substrate, (ii)molecules capable of affecting epigenetics, and (iii)soluble hormones "sensed" by membrane receptors [Citation16]. Signaling transduction induced by lactic acid is an important pathway in the human body [Citation17]. These processes are mediated by receptors or transporters. The production of lactic acid mainly occurs in the cytoplasm and then it is secreted through the plasma membrane. This transport is mediated by lactate-dependent transmembrane protein (MCT) or sodium-dependent co-transport (SLC5A8, SlC5A12) [Citation1,Citation16]. There are four members of the MCT family, all of which share conserved sequence motifs, but their substrate specificity, transport rate and expression pattern are different. All MCTs can promote the import or export of lactate according to differences in concentration. However, several studies have determined that MCT4 is responsible for exporting lactate to extracellular space [Citation18], and MCT1 regulates its transport across the plasma membrane in most lactate-producing tissues [Citation1].
Extracellular lactic acid has a concentration of 1.5 mM in physiological conditions and 20–40mM in pathological conditions. These levels have a significant impact on cell functions because it leads to the activation of different signaling pathways [Citation1,Citation19]. For example, lactic acid coordinates adaptive metabolic responses in muscle cells during endurance training, which induces an increase in MCT1 expression in reactive oxygen species(ROS)-dependent/NRF2-mediated signaling pathway [Citation2]. In neurons, lactic acid derived from astrocytes alters the intracellular redox state through the imbalance of NADH/NAD+, thereby stimulating the expression of N-methyl-D-aspartic acid(NMDA) receptor and ERK1/2-mediated genes associated with neuronal plasticity [Citation3,Citation20]. At the intracellular level, lactate can stabilize HIF-1α by inhibiting prolyl hydroxylase2(PHD2), thus ensuring cell adaptation under hypoxia [Citation21]. Lactic acid promotes the expression of VEGF mediated by HIF-1α in cancer cells and the expression of bFGF and VEGFR-2 in adjacent endothelial cells. Lactic acid also induces the stabilization of HIF-2α and enhances the decomposition of glutamine in cancer cells. A recent study reported that lactic acid regulated the expression of homeostasis genes in macrophages through a histone post-translational modification function called histone lysine lactation [Citation22].
The signaling transduction function of lactic acid in extracellular space is mediated by the activation of G-protein-coupled receptor GPR [Citation5,Citation23–26]. In physiological environment, lactic acid binds to GPR81, thus inhibiting lipolysis in adipocytes [Citation27]. In cancers, GPR81 is highly expressed in different cancer cell lines, including colon cancer, breast cancer, lung cancer, liver cancer, cervical cancer, and pancreatic cancer [Citation28,Citation29]. In vitro, the expression of GPR81 is associated with the survival, proliferation, migration, invasion and chemotherapy resistance of cancer cells, and participates in the inhibition of anti-tumor immunity by promoting the overexpression of PD-L1 in lung cancer cell lines [Citation28]. During inflammation, lactic acid activated the GPR81-mediated pathway, inhibitted inflammation and reduced organ damage [Citation30]. Another study revealed that high levels of lactic acid produced during childbirth acted on uterine GPR81, and down-regulated the key pro-inflammatory genes [Citation31]. In addition, GPR4, GPR65, GPR68 and GPR132, acted as lactic acid sensors, are activated in acidic tumor microenvironment (TME) due to the low pH value of lactic acid [Citation32]. It remains to be clarified whether the regulation of the lactic acid signaling pathway occurs directly through GPR-lactic acid interaction or through conformational modification of the receptor induced by lactic acidosis.
3. Biology of macrophage polarization
Macrophages can differentiate into different phenotypes and perform different functions based on different microenvironmental signals [Citation33,Citation34]. The researchers divided macrophages into M1-phenotypes (classically activated macrophages, CAM) and M2-phenotypes (alternatively activated macrophages, AAM) [Citation18,Citation35]. Under normal physiological conditions, a dynamic balance is maintained between M1 and M2 macrophages. Before discussing further, we must first understand the function of M1 and M2 macrophages. The features of the different phenotypes of activated macrophages are shown in .
Table 1. Features in different phenotypes of activated macrophages.
3.1. M1: classically activated macrophages
Classical M1-type macrophages are induced by interferon-γ (IFN-γ) and T-helper1 cells (Th-1) or pathogen-derived lipopolysaccharide (LPS) [Citation36]. They can promote the production of reactive oxygen species (ROS) and nitrogen intermediates, as well as inflammatory factors such as IL-1β, TNF-α and IL-6. They also upregulate the expression of CD86 and CD80, induce nitric oxide synthase (iNOS), CXC chemokine ligand 9 (CXCL9), CXCL10 and monocyte chemoattractant protein-1, and regulates the immune responses through Th1 and Th17 cells, which are beneficial to pro-inflammatory, bactericidal and anti-tumor effects [Citation37]. Recent studies have established the metabolic characteristics of M1/M2 macrophages, indicating that macrophage metabolism also dominated the functional phenotypic changes of macrophages [Citation38].
3.2. M2: alternatively activated macrophages
M2-type macrophages are induced by IL-4, IL-13, IL-10 or immune complexes. They up-regulate the expression of arginase-1 (Arg-1), CD206 and CD163, and regulate Th2 cells. M2 macrophages also produce VEGFA, TGF-β and IL-8, which are responsible for angiogenesis and lymphangiogenesis, which also are essential for tissue and wound healing [Citation39]. M2 macrophages can be further divided into three subtypes: M2a, M2b and M2c, and each subtype performs different functions [Citation40–42]. M2a-type macrophages are induced by IL-4 or IL-13, promote cell growth and tissue repair [Citation41]. M2b-type macrophages are induced by immune complex or LPS/IL-1 stimulation, suppress acute inflammatory response. M2c-type macrophages are induced by glucocorticoids, IL-10 or TGF-β, play a role in regulating immunity and suppressing inflammation [Citation42]. Cell metabolism [Citation43–47], such as lipid metabolism and iron metabolism, also play an important role in activating the M2-type macrophages.
4. M1/M2 imbalance in human diseases: inflammation and cancer
Under pathological conditions, the transition of the macrophage phenotype to either the M1 or M2 polar extreme may lead to the progression of a variety of diseases [Citation39,Citation48]. Molecules associated with M1/M2 polarization include members of the PPAR, KLF, IRF, STAT, NF-κB and HIF families [Citation25,Citation49]. In addition, the miRNA and epigenetic modification have also become regulators of macrophage transformation [Citation24,Citation25]. A better understanding of the role of macrophage phenotypes and the mechanisms during the disease progression may provide new treatment strategies [Citation42,Citation50].
4.1. Macrophage polarization and inflammation
Recent studies have found that macrophage polarization is closely related to the occurrence and development of inflammation-related diseases, such as acute injuries, sepsis, cardiovascular diseases, autoimmune inflammation, obesity, diabetes and neurodegenerative diseases [Citation25,Citation49]. In atherosclerosis (AS), M1-type macrophages mainly exist in the early plaques and promote the formation of atherosclerotic plaque, while M2-type macrophages is mainly related to the progression of plaque, promoting the formation of fibrous caps and enhancing the stability of plaques [Citation51]. Another study found that [Citation23], in different stages of acute myocardial infarction, M1-subtypes macrophages played a dominant role in the early stage of inflammation and secreted damaging pro-inflammatory cytokines. M2-macrophages expressed high levels of IL-10 and promoted repair in the late stage of scar formation. Short-term aminooxyacetic acid (AOAA) treatment can balance macrophage polarization by regulating macrophage metabolism and inhibiting the NLRP3-Caspase1/IL-1β pathway, thus significantly improving the cardiac function of MI mice. Pioglitazone, a peroxisome proliferator-activated receptor-γ (PPAR-γ) agonist, had a unique anti-inflammatory effect on acute inflammation caused by macrophages and promoted heart healing after AMI, thereby protecting the heart from IR damage [Citation52]. In the pathogenesis of rheumatoid arthritis (RA), M1-type macrophages promoted synovial inflammation, while the polarization of M2-type macrophages improved the severity of arthritis [Citation53]. In the mouse model of systemic lupus erythematosus (SLE), there were a large number of macrophages infiltrating in the kidney, and the polarization of M2b-type macrophages alleviated lupus symptoms in mice [Citation54,Citation55]. Further studies have shown that activated NF-κB could up-regulate the transcription of HIF-1α [Citation56], promote glycolysis and the polarization of M1-type macrophages [Citation57], and enhance its bactericidal properties [Citation58]. Activated AMPK can regulate the balance of M1/M2 macrophages by inhibiting the NF-κB signaling pathway [Citation59] and reducing the inflammatory response [Citation60]. Therefore, promoting the polarization of M2 macrophages provides a new method of treatment for inflammation-related diseases [Citation61], such as local inflammation [Citation62], pulmonary inflammation [Citation63], hepatitis [Citation64,Citation65], acute renal injury (AKI) [Citation66], ischemic disease [Citation67,Citation68], insulin resistance [Citation69] and metabolic diseases [Citation47].
4.2. Macrophage polarization and cancer
Macrophages, identified as tumor-associated macrophages (TAMs), abound in the tumor stroma [Citation70]. TAMs are a major component of tumor infiltrating leukocytes in cancers [Citation14,Citation71]. In tumor microenvironment, the macrophages acquire M2-phenotypes that have tumor promoting functions [Citation49]. They promote tumor growth and spread, maintain angiogenesis, promote matrix degradation, and inhibit adaptive immune responses against tumors [Citation70,Citation72]. TAMs are preferentially located in the hypoxic region of the tumor, where they express HIF-1α, which induces the transcription of VEGF, bFGF, PDGF and prostaglandin E2, and promotes angiogenesis and metastasis [Citation71]. Macrophages produce enzymes and proteases that regulate extracellular matrix (ECM) [Citation73]. The destruction of ECM promotes the migration and diffusion of tumor cells. TAMs also contribute to the immunosuppressive environment to block T cell proliferation and inhibit cytotoxic T cell response [Citation74]. Based on the tumor-promoting function of TAMs, TAMs infiltration is usually associated with reduced survival in patients with lung cancer [Citation75], breast cancer and classic Hodgkin’s lymphoma [Citation76]. Therefore, inhibiting the aggregation of M2 macrophages in tumor tissue and intervening in its polarization can provide new hope for the treatment of tumors [Citation76].
A recently published study [Citation77] developed a novel adoptive cellular immunotherapy that built a "backpack" of IFN-γ for macrophages to keep them in a tumor-killing state, known as M1-type. The immunotherapy was effective in slowing tumor growth and reducing metastasis in a mouse model of invasive breast cancer. Another exciting treatment was found to enhance chemotherapy or immunotherapy by eliminating the immunosuppressive activity of macrophages [Citation74].
5. Lactic acid and metabolic reprogramming of macrophages
The activated macrophages also undergo profound metabolic changes, called “metabolic reprogramming”, which are similar to the changes in metabolic pathway experienced by rapidly-proliferating tumor cells [Citation78]. Studies have confirmed that the metabolic patterns of M1 and M2-type macrophages are significantly different. It is currently believed that M1-type macrophages rely on the aerobic glycolysis pathway and the pentose phosphate pathway to provide energy, while M2-type macrophages obtain energy mainly through fatty acid oxidation and oxidative phosphorylation [Citation79].
Studies have shown that activated M1-type macrophages were still able to produce ATP rapidly through glycolysis, even when oxygen was sufficient [Citation80]. LPS can induce the transformation of macrophage metabolic pathways from OXPHOS to aerobic glycolysis and promote M1-type polarization, and finally promoted the synthesis of lactic acid, secretion of inflammatory mediators and ROS production, which contributed to the inflammatory response and pathogen clearance [Citation80–83].
Compared with M1-type macrophages, M2-type macrophages have different metabolic profiles and inflammatory phenotypes. An increase in OXPHOS flux in M2-type macrophages led to an increase in the expression of anti-inflammatory cytokines such as IL-10 and a decrease in the expression of NO and TNF-α. In addition, M2-type macrophages mainly expressed PFKFB1, the homotype of PFK2. It was easier to decompose F-2,6-BP to fructose-6-phosphate, thus reducing the glycolysis activity [Citation79]. The uptake and oxidation of fatty acids in mitochondria were the driving forces of M2 macrophages, and metabolic programs of mitochondria were controlled by AMPK and its downstream targets (including PPAR) [Citation84]. IL-4 and IL-13 can increased the expression of PPAR and its coactivator PGC-1β, which led to an increase in the expression of mitochondrial respiratory chain protein [Citation85]. Therefore, the main metabolic differences seemed to be due to the different functions of M1-type and M2-type macrophages.
In addition, locally high concentrations of lactic acid created a negative feedback mechanism to regulate macrophage metabolism. It has been shown that high concentrations of lactic acid inhibited the glycolysis of immune cells, immediately reduced the extracellular acidification rate (ECAR) and increased the oxygen consumption rate (OCR) [Citation86]. When lactic acid was added under inflammatory conditions (4 hours after LPS stimulation), ECAR decreased and OCR increased, resulting in a sharp increase in the ratio of OCR/ECAR, which responded to the metabolic state with an anti-inflammatory response to inhibit inflammation [Citation87]. The acute effects of extracellular lactic acid on monocyte metabolism were eliminated if the cells were pretreated with oxalate, an LDH inhibitor. It was found that lactic acid inhibited PFK-1, a key glycolytic enzyme, and promoted the decomposition of active PFK-1 tetramers into less active dimers, thereby reducing the glycolysis flux in monocytes [Citation88]. Lactic acid was injected into diabetic patients with normal blood glucose levels (5.1 ± 0.2 mmol/L). 20 minutes after injection, the plasma lactic acid level increased from 1.3 ± 0.6 mM to 3.7 ± 0.5 mM (paired 0.002). It was found that in vitro lactic acid therapy could significantly increase the OCR/ECAR in monocytes of diabetic patients, which indicated that lactic acid had significant anti-inflammatory effects on diabetic patients [Citation87]. These metabolic characteristics provide us with many potential drug targets for the treatment of inflammatory diseases, metabolic diseases and cancer.
6. Effect of lactic acid on macrophage polarization
The concentration of physiological lactic acid is approximately 1.5–3mM, inncreasing to 10 mM in blood and healthy tissues, and reaching as high as 25 mM after strenuous exercise. In inflammatory diseases, such as atherosclerotic plaques or rheumatic synovial fluid, the concentration of lactic acid can be around 10 mM, and in cancer tissues it can even reach to 20–40mM [Citation19,Citation89]. The accumulation of lactic acid had a great impact on the macrophages that had infiltrated and were staying in the tissue, which further influenced disease progression.
A recent study found that lactic acid was the driving force for the proliferation of TAMs during epithelial-mesenchymal transformation [Citation90]. Toshimitsu Ohashi reported [Citation91] that the lactic acid secreted by a tumor was related to the macrophages in the tumor. Lactic acid was also found to promote the polarization of M2-like macrophages in human head and neck squamous cell carcinoma(HNSCC). Another study found that in the muscle microenvironment, endothelial cells were activated and released lactic acid, which promoted the MCT1-dependent oxidative transition in macrophages. It appeared that the macrophages were polarized toward M2-like phenotypes, and they promoted muscle regeneration by stimulating the proliferation and differentiation of MPC [Citation10]. In addition, macrophages polarized by lactic acid upregulated the expression of vascular endothelial growth factor (VEGF), which formed a positive feedback loop and further stimulated angiogenesis. These results were consistent with previous observations in tumors, where lactic acid induced macrophage polarization by relying on MCT uptake or acted as a signal molecule to initiate GPR132 signal transduction and promote angiogenesis [Citation32]. In conclusion, the results of these studies demonstrated that lactic acid can induce monocytes to differentiate into M2 macrophages in a dose-dependent manner through metabolic reprogramming, so as to promote tumor growth in the microenvironment. Similarly, in the late stage of inflammation, macrophages were often observed to have an M2-like immunophenotype which played a key role in the pathogenesis of immune system dysfunctions [Citation92]. The studies have shown that lactic acid controlled the polarization of M2-like macrophages, but the exact molecular mechanism is still unknown. Recent studies have shown that lactic acid may polarize macrophages into M2-like phenotypes through epigenetic mechanisms (histone lysine lactic acid, acetylation, etc.) [Citation13].
7. Effects of lactic acid signaling on inflammatory response
Previous studies had found that lactic acid signaling inhibited inflammation [Citation87,Citation93,Citation94]. In LPS-stimulated human monocytes, low concentrations of lactic acid (<or = 10mM) significantly inhibited the secretion of TNF during inflammation. The underlying mechanism may be due to lactate interfering with glycolytic flux [Citation87,Citation94]. In vitro studies, it was found that lactic acid inhibited the activation of mononuclear macrophages mediated by TLR and inhibited the secretion of cytokines TNF-a, IL-23 and chemokines CCL2 and CCL7. Further analysis showed that lactic acid delayed the phosphorylation of AKT and the degradation of IκBα [Citation94]. Another in vitro and in vivo study [Citation19] demonstrated that extracellular lactic acid induced metabolic reprogramming in innate immune cells. Short-term infusion of lactic acid in the human body increased glucose consumption of PBMC in vitro, and significantly regulated the production of cytokines, resulting in anti-inflammatory effects. Moreover, extracellular lactic acid was found to be time-and stimulus-dependent on cytokines production, which further emphasized the complex interaction between metabolism and function in immune cells. Carolina Iraporda [Citation95] investigated the effect of lactic acid on inflammation in vivo and found that an increase in lactic acid in the colon enhanced the protective effect of TNBS-induced colitis, thus preventing tissue damage, bacterial translocation and reducing the level of serum IL-6. In summary, these studies revealed that lactic acid regulated the metabolism of immune cells and reduced inflammation and may eventually serve as a negative feedback signal to prevent excessive inflammation.
Lactic acid can activate the G-protein-coupled receptor GPR81 (also known as HCA1). The activation of GPR81 in leukocytes induced by lactic acid specifically inhibited both the activation of NF-κB and inflammatory bodies induced by TLR4 and the downstream production of pro-IL-1β and pro-IL-18 [Citation30]. It is well known that the TLR4-mediated activation of the NF-κB pathway induces an inflammatory state by producing a series of inflammatory cytokines or mediators [Citation93]. In another study, beneficial active molecules in lactic acid bacteria inhibited inflammatory cytokines and chemokines by down-regulating the expression of TLR and the NF-κB pathway [Citation93].
We considered that the active molecule may be the lactic acid probably. Lactic acid produced locally by intestinal probiotics down-regulated the inflammatory response of intestinal epithelial cells and medullary cells by preventing activation of TLR and IL-1β, which was helpful to the protection of the intestinal tract during inflammation [Citation96]. It was found that lactic acid bacteria (LAB) promoted intestinal stem cell (ISC) mediated epithelial development and induced ISC proliferation. The mechanism was mainly occurred through lactic acid stimulation of Gpr81/Wnt/β-catenin signals in Paneth cells and intestinal stromal cells [Citation7]. These results suggested that lactic acid may contribute to the health of the intestinal mucosa through its anti-inflammatory and reparative effects [Citation96–98]. Another study revealed a new link between lactic acid and the control of uterine inflammation where high levels of lactic acid produced during childbirth acted on uterine GPR81 and down-regulated the pro-inflammatory genes. This was the first study on the pharmacological effects of lactic acid in the uterus during pregnancy [Citation31]. In atherosclerosis, lactic acid-mediated activation of GPR81 can significantly reduce oxidative stress and down-regulate the expression of inflammatory cytokines such as IL-8 and monocyte chemoattractant protein (MCP). It was also found that activation of GPR81 reduced the expression of Kruppel-like factor-2 (KLF2), a key atherosclerotic protective transcription factor induced by OSS. These findings suggested that GPR81 had a potential protective effect on atherosclerosis and targeting GPR81 may inhibit OSS-induced endothelial inflammation and dysfunction [Citation99]. In 2020, KunYang discovered a new mechanism, found that lactic acid inhibited the activation of YAP and NF-κB during inflammation through GPR81-mediated signal transduction, and inhibited the pro-inflammatory response of macrophages stimulated by LPS [Citation89].
Lactic acid can promote the steady-state polarization of macrophages by inhibiting interferon-mediated pathways. The direct binding of lactic acid molecules to mitochondrial antiviral signaling protein (MAVS) of the RLR connector inhibited the induction of IFN-β [Citation100]. This study established the key role of lactic acid in limiting RLR signal transduction, and identified MAVS as a direct sensor of lactic acid, whose function is to connect energy metabolism and innate immunity.
In 2019, convincing evidence was found regarding the homeostatic function of lactic acid. It was shown that covalent coupling between lactic acid and histone lysine residues of macrophages promoted the transition of macrophages to a steady-state phenotype through the epigenetic process of histone lactic acidification, and expressed genes with M2-like function, which transformed macrophages from the inflammatory phenotype to the anti-inflammatory phenotype [Citation13,Citation101].
Possible mechanisms for the anti-inflammatory effects of lactic acid are summarized as follows: (i) lactic acid may play an anti-inflammatory effect through the lactic acid receptor GPR81; (ii) lactic acid may drive a negative feedback loop to inhibit glycolysis, thus inhibiting the release of pro-inflammatory factors from macrophages. (iii) lactic acid can promote the steady-state macrophages polarization by inhibiting RLR signal transduction. (iv) The epigenetic process of lactic acid acidification through macrophages histones. These findings provide further support that lactic acid can be a new treatment strategy to regulate inflammation. The mechanisms of the anti-inflammatory effects of lactic acid are illustrated in .
Figure 1. The signaling transduction function of lactic acid in macrophage in inflammation. Lactic acid has two main ways to transmit signals to cells: mediated by transporters(McT) or sodium-dependent co-transports (SLC5A8,SlC5A12); And receptors(GPR). i. Lactic acid negative feedback inhibits glycolysis; ii. Lactic acid activates Wnt/?-catenin and Yap/Taz signaling pathway, inhibits NF/?B signaling pathway through GPR on the cell membrane; iii. Lactic acid binds to MAVS and inhibits the interaction of RIG-1; iv. Lactic acid enters the nucleus, binds to histone lysine residues, and directly activates the expression of M2 gene through histone lactylation.
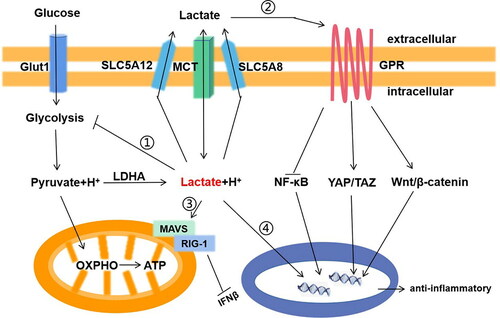
8. Effects of lactic acid signaling on malignant tumors
Studies have shown that tumor cells can increase glycolysis flux as a metabolic strategy, and increased glycolysis produced lactic acid [Citation102], which led to the acidification of the cell microenvironment [Citation103]. The lactate biosynthesis of cancer cells was mainly attributed to an imbalance in three transcription factors: HIF-1a, C-MYC and p53 [Citation102]. Clinically, the expression of HIF-1a was related to glycolysis and the high invasiveness and short survival in patients with malignant tumors [Citation104–108]. In addition, it was recently discovered that lactic acid promoted the stem characteristic and epithelial-mesenchymal transformation of cancer stem cells and promoted tumor progression. Zhenhe Suo reported that the increase of lactic acid enhanced the malignant characteristics of esophageal squamous cell carcinoma [Citation109]. In addition, the team also reported that lactic acid enhanced the stem cell phenotype and survival of prostate cancer cells [Citation9].
Lactic acid stimulated resident macrophages to polarize into M2-type, which played an important role in immunosuppression and cancer progression [Citation110]. Oscar R. Colegio et al. [Citation111] proved that HIF-1α mediated the effect of lactic acid promoting M2-like polarization of TAMs [Citation111]. The lactic acid released by tumor cells upregulated HIF-1α in macrophages, thus forming a positive feedback loop between TAMs and tumor cells [Citation111]. Another research found that lactic acid secreted by breast cancer cells increased level of ROS in macrophages through Nrf2-mediation and induced M2-type macrophages and VEGF expression, thus promoting epithelial-mesenchymal transformation (EMT) of cancer cells. This study provided a new understanding of the role of Nrf2 in the interaction between cancer cells and TAMs, and put forward potential therapeutic targets [Citation90].
The activation of ERK/STAT3, the main signal molecule in lactic acid signaling pathway, deepened our understanding of how lactic acid induced TAMs on a molecular level [Citation112]. A recent study showed that lactic acid activated PI3Kδ/γ played an important role in signal transduction to induce TAMs polarization and subsequently promote tumor growth in Hodgkin’s lymphoma [Citation113]. In addition, the inhibition of ERK/STAT3 signal reduced tumor growth and angiogenesis by eliminating the polarization of M2 macrophages. Although GPR81 was previously reported to be expressed only in adipocytes, it was found to be expressed in macrophages as well. Lactic acid derived from tumor cells up-regulated TAZ-dependent PD-L1 expression in human lung cancer cells through GPR81, which enhanced immune escape [Citation28,Citation114].
Na Liu et al. [Citation115] showed that high concentrations of lactic acid in tumors promoted the M2-like TAM phenotype of tumors. Lactic acid activated the signal transduction mediated by mammalian rapamycin target complex 1 (mTORC1) in macrophages, resulting in a decrease of TFEB expression and its downstream target genes, including the D2 subunit of Atp6v0d2. A decrease in ATP6V0D2 expression led to a decrease in HIF-2α, thus inducing the high expression of Vegf and M2 homeostasis genes Mrc1, Arg1 and Retnla [Citation21]. These results indicated that the lactic acid/ATP6V0d2/HIF-2α axis in macrophages played a key role in maintaining tumor growth [Citation115].
In addition, lactic acid was proven to be a weak HDAC inhibitor [Citation24]. Lactic acid can regulate the level of histone acetylation in macrophages and change their activation state, thus promoting the polarization of TAMs to M2-like phenotypes, and inducing the expression of M2-macrophage markers such as E-Cadherin, MMR, Ym and Fizzl to promote tumor growth. A recent study further confirmed that lactic acid led to the production of TAM phenotypes in tumors, thereby promoting tumor growth [Citation116]. The mechanism was attributed to the activation of plasma membrane G-protein-coupled-receptor and the downstream cAMP-dependent signal transduction and M2-like gene expression mediated by lactic acid, as well as the transcriptional repressor cAMP response element modulator (CREM). The mechanism of lactic acid on TAMs is illustrated in .
Figure 2. The signaling transduction function of lactic acid in macrophage in cancer. i. Lactic acid activates cAMP and CREM through GPR on the cell membrane. ii. Lactic acid activates mTORC1/ATP6V0D2 pathway and expression of HIF2a; iii. lactic acid activates the expression of transcription factor Nrf2; iv. Lactic acid activates PI3K/ Akt pathway; v. Lactic acid activates the ERK/STAT3 pathway; vi. lactic acid enters the nucleus, binds to histone lysine residues, and directly activates the expression of M2-like genes.
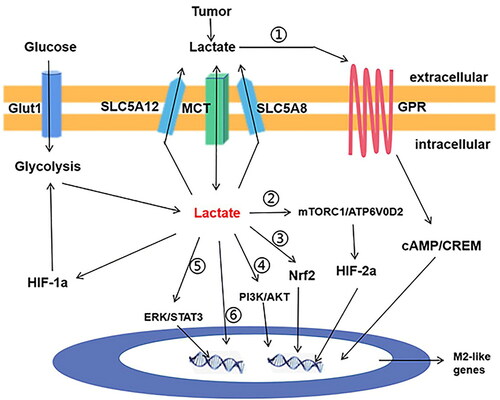
In addition to TAMs, lactic acid also promoted tumor progression through other mechanisms in the tumor microenvironment. An increase in the level of lactic acid promoted the emergence of the immune escape microenvironment by weakening the activation of dendritic cells and T cells and decreasing monocyte migration [Citation117]. Lactic acid also stimulated fibroblast cells to produce hyaluronic acid, which contributed to tumor invasiveness. Extracellular acidification can stimulated the activities of matrix metalloproteinases (MMPs) and cathepsin, promoted the degradation of extracellular matrix components and enhanced tumor invasiveness. Lactic acid damaged the immune surveillance of effector T cells and NK cells by blocking glycolysis flux and IFN-γ secretion [Citation117]. To summarize, the interaction between cancer cells and their microenvironment further affected the metabolism of cancer cells and made them more aggressive, forming a positive feedback of malignant proliferation.
9. Targeting the lactic acid-controlled macrophage polarization
9.1. Potential drug targets for lactic acid-controlled macrophage polarization in inflammatory diseases
Inflammation is a favorable defense response of the body against microbial invasion and external stimulation. Inflammatory cells, such as macrophages and neutrophils, are rapidly activated to produce pro-inflammatory mediators and reactive oxygen species to eliminate microbial infection [Citation118]. However, excessive inflammation often causes tissue damage. As mentioned previously in this paper, lactic acid and macrophage polarization plays an important role in the occurrence and development of inflammatory diseases [Citation118]. This observation can be used as a basis for the treatment of inflammatory diseases [Citation119]. PHD1 targeting deletion or hydroxylase inhibition could protect mice from colitis induced by sodium dextran sulfate (DSS) and reduce epithelial erosion, immune cell infiltration and colonic microvascular dysfunction [Citation120,Citation121]. Such a result might be partially dependent on the enhancement of glycolysis to produce lactic acid. Hoque et al. [Citation30] proved that exposure to high concentrations of lactic acid could inhibit inflammation of the liver and pancreas, and reduce injury to organs.
In a study of cardiac inflammation caused by sepsis, it was found that hypertonic sodium lactate could prevent cardiac dysfunction, alleviate mesenteric microcirculation and capillary leakage during sepsis, while reducing cardiac inflammation [Citation122]. Furthermore, in addition to immune regulation, lactic acid stimulated the proliferation of intestinal cells and maintained an intestinal barrier in mice during starvation experiments. Lactic acid reduced the TLR expression and IL-1β-dependent NF-kappaB activation of intestinal epithelial cells [Citation123]. Therefore, targeting lactic acid, its signaling pathways and macrophages polarization may be potential new therapeutic targets for the treatment of inflammation. More experimental studies and clinical trials should be conducted to prove this hypothesis.
9.2. Potential drug targets for lactic acid-controlled macrophage polarization in the progression of malignant tumors
Studies have shown that lactic acid at the tumor site promoted the polarization of M2 macrophages, increased tumor angiogenesis and promoted tumor progression. In recent years, the targeting of lactic acid, its signaling pathways and tumor-associated macrophages were investigated as potential new therapeutic targets for the treatment of malignant tumors. In particular, LDH and MCT inhibitors, such as AR-C155858, SR13800 and AZ3965, have anticancer effects [Citation16].
Preclinical studies have found that AZ3965 achieved promising results [Citation124]. AZ3965 was effective in the treatment of Burkett’s lymphoma, breast cancer, gastric cancer, and small cell lung cancer (SCLC) tumors [Citation124]. A phase I/II clinical trial (NCT01791595) is currently ongoing for patients with solid cancer (especially prostate and gastric cancer) and diffuse large B-cell lymphoma.
MCT4 inhibitor AZ93 is highly selective and is also being used in preclinical studies [Citation125]. Another potentially effective treatment is to target the LDH enzyme which can bi-directionally convert pyruvate to lactic acid. Tae-Wook Chung et al. [Citation126] reported that machilinA (MA), the strongest inhibitor of LDHA, significantly reduced the production of lactic acid in cancer cells, regulated the polarization of alternately activated macrophages (M2), decreased neovascularization and prevented tumor growth.
Lactic acid participates in the polarization signaling of M2 macrophages, such as ERK1/2, STAT3, HIF-1a and STAT6. The inhibition of these signaling pathways may play an anti-tumor effect. Xianmin Mu et al. [Citation112] found that the combination of STAT3 and ERK inhibitors (selumetinib and statistic) could reverse M2-phenotype and inhibit the migration and proliferation of tumor cells. In addition, it was found that WithaD can also effectively prevent M2 polarization of macrophages by reducing ERK/STAT3, thus inhibiting breast cancer progression and tumor angiogenesis in vitro and in vivo.
G-protein-coupled-receptor 132 (Gpr132) acted as a key macrophage sensor for lactic acid in the acidic tumor environment and Gpr132 deletion reduced M2 macrophages and hindered lung metastasis of breast cancer in mice [Citation32]. Targeting lactic acid at tumor sites directly inhibited tumor growth. Qi-Wen Chen et al [Citation127] used Shewanella oneidensis MR-1 to form a bacterial respiratory pathway at the tumor site, which led to continuous catabolism of lactic acid. In addition, decorated MnO2 nanoflowers prevented lactic acid production by down-regulating the expression of hypoxia-inducible factor-1α (HIF-1α), and finally inhibited tumor progression by combining bacterial respiration with tumor metabolism. Of course, modulating the metabolic reprogramming of macrophages can play an anti-tumor effect. It was found that 6-gingerol acted as an arginase inhibitor to reverse macrophage polarization, which produced an anti-tumor effect [Citation128]. The above studies showed that reducing the production of lactic acid and blocking the lactic acid signaling pathway can be used as a treatment for cancer.
Finally, another underestimated problem facing targeted metabolism is the role of the immune system in the anti-cancer response. Recently, it was reported that reducing the production of lactic acid in cancer cells may have a synergistic effect with immunotherapy. The expression of programmed cell death protein 1 (PD-1) and its ligand (PD-L1) was regulated by high levels of lactic acid in the tumor microenvironment (TME) [Citation114]. The efficacy of anti-PD-L1 therapy can be improved by blocking lactate dehydrogenase-A (LDH-A) [Citation129]. Fan Gao et al. [Citation130] used hollow MnO2 camouflaged by erythrocyte membrane with a catalytic nano-system with lactate oxidase (LOX) and glycolysis inhibitors for intracellular/extracellular lactic acid absorption and tumor synergistic metabolic therapy and immunotherapy. It was also proven that this TME regulation strategy can effectively improved the anti-tumor effect of anti-PD-L1 therapy. Tariq M. Rana et al. [Citation131] also found that small molecular Alkbh5 inhibitors regulated the lactic acid in the tumor microenvironment and enhanced the efficacy of cancer immunotherapy. To summarize, lactic acid in malignant tumors can control the polarization of macrophages. This technique can be a promising therapeutic target for malignant tumors.
10. Conclusion
Lactic acid is not only a by-product of metabolism, but it also participates in regulating macrophages function and phenotype. The two different phenotypes of macrophages have different functions in tumors and inflammation-related diseases. This implies that targeting lactic acid can be an effective treatment for life-threatening diseases. The metabolic pathways associated with this phenomenon present several novel therapeutic drug targets, such as LDHA, MCT1 and MCT4 for cancer. Small molecule inhibitors have been identified for these targets, some of which are currently being investigated in clinical trials for cancer treatment. This would be an ideal cancer treatment strategy with no unwanted side effects. In addition, lactic acid is involved in the regulation of macrophage functions and phenotypes, and it plays an important role in inflammation-related diseases. This implies that the metabolism of lactic acid can be used effectively to treat inflammation-related diseases. Recent studies have shown that lactic acid acted as an agonist for the GI/O protein-coupled receptor GPR81 on the cell surface, revealing another potential drug target in this metabolic pathway. Further clinical trials should be conducted and more scientific evidence should be gathered to prove the relationship between lactic acid and macrophages and its prospects for the treatment of diseases. Finally, improvements in metabonomics and other immunometabolism-based techniques will contribute significantly to enhancing the existing knowledge of immunometabolism. Much more knowledge remains to be explored in this area, including epigenetic regulation of lactic acid metabolism and inflammation in immune cells, as well as as-yet-undiscovered immune metabolic signaling pathways.
Author’s contributions
Hai-Cun Zhou, Xin-Yan Yan and Wen-Wen Yu drafted the paper. Guang-Hui Zhao and Hong-Bin Liu conceived and designed the paper. Hai-Cun zhou, Zhi-chang Liu, Xiao-Qin Liang and Xiao-yan Du revised and finalized the paper. Jian-ping Long provided the financial support. All authors read and confirmed the final paper.
Consent for publication
All authors have agreed to the publication of the paper.
Declaration of interest
We confirm that there are no conflicts of interest pertaining to this paper.
Additional information
Funding
References
- Certo M, Marone G, de Paulis A, Mauro C, Pucino V. Lactate: fueling the fire starter. Wiley Interdiscip Rev Syst Biol Med. 2020;12(3):e1474. doi:https://doi.org/10.1002/wsbm.1474.
- Brooks GA. The science and translation of lactate shuttle theory. Cell Metab. 2018;27(4):757–785. doi:https://doi.org/10.1016/j.cmet.2018.03.008.
- Magistretti PJ, Allaman I. Lactate in the brain: from metabolic end-product to signalling molecule. Nat Rev Neurosci. 2018;19(4):235–249. doi:https://doi.org/10.1038/nrn.2018.19.
- Sun S, Li H, Chen J, Qian Q. Lactic acid: no longer an inert and end-product of glycolysis. Physiology (Bethesda). 2017;32(6):453–463. doi:https://doi.org/10.1152/physiol.00016.2017.
- Ivashkiv LB. The hypoxia-lactate axis tempers inflammation. Nat Rev Immunol. 2020;20(2):85–86. doi:https://doi.org/10.1038/s41577-019-0259-8.
- Flores A, Schell J, Krall AS, et al. Lactate dehydrogenase activity drives hair follicle stem cell activation. Nat Cell Biol. 2017;19(9):1017–1026. doi:https://doi.org/10.1038/ncb3575.
- Lee YS, Kim TY, Kim Y, et al. Microbiota-derived lactate accelerates intestinal stem-cell-mediated epithelial development. Cell Host Microbe. 2018;24(6):833–846 e6. doi:https://doi.org/10.1016/j.chom.2018.11.002.
- Schell JC, Wisidagama DR, Bensard C, et al. Control of intestinal stem cell function and proliferation by mitochondrial pyruvate metabolism. Nat Cell Biol. 2017;19(9):1027–1036. doi:https://doi.org/10.1038/ncb3593.
- Zhong Y, Li X, Yu D, et al. Application of mitochondrial pyruvate carrier blocker UK5099 creates metabolic reprogram and greater stem-like properties in LnCap prostate cancer cells in vitro. Oncotarget. 2015;6(35):37758–37769. doi:https://doi.org/10.18632/oncotarget.5386.
- Zhang J, Muri J, Fitzgerald G, et al. Endothelial lactate controls muscle regeneration from ischemia by inducing M2-like macrophage polarization. Cell Metab. 2020;31(6):1136–1153 e7. doi:https://doi.org/10.1016/j.cmet.2020.05.004.
- Ko SH, Nauta AC, Morrison SD, et al. PHD-2 suppression in mesenchymal stromal cells enhances wound healing. Plast Reconstr Surg. 2018;141:55e–67e.
- Vinaik R, Barayan D, Auger C, Abdullahi A, Jeschke MG. Regulation of glycolysis and the Warburg effect in wound healing. JCI Insight. 2020;5(17):e138949. doi:https://doi.org/10.1172/jci.insight.138949.
- Zhang D, Tang Z, Huang H, et al. Metabolic regulation of gene expression by histone lactylation. Nature. 2019;574(7779):575–580. doi:https://doi.org/10.1038/s41586-019-1678-1.
- Biswas SK, Chittezhath M, Shalova IN, Lim JY. Macrophage polarization and plasticity in health and disease. Immunol Res. 2012;53(1–3):11–24. doi:https://doi.org/10.1007/s12026-012-8291-9.
- Chen X, Tang J, Shuai W, Meng J, Feng J, Han Z. Macrophage polarization and its role in the pathogenesis of acute lung injury/acute respiratory distress syndrome. Inflamm Res. 2020;69(9):883–895. doi:https://doi.org/10.1007/s00011-020-01378-2.
- Ippolito L, Morandi A, Giannoni E, Chiarugi P. Lactate: a metabolic driver in the tumour landscape. Trends Biochem Sci. 2019;44(2):153–166. doi:https://doi.org/10.1016/j.tibs.2018.10.011.
- Haas R, Cucchi D, Smith J, Pucino V, Macdougall CE, Mauro C. Intermediates of metabolism: from bystanders to signalling molecules. Trends Biochem Sci. 2016;41(5):460–471. doi:https://doi.org/10.1016/j.tibs.2016.02.003.
- Tan Z, Xie N, Banerjee S, et al. The monocarboxylate transporter 4 is required for glycolytic reprogramming and inflammatory response in macrophages. J Biol Chem. 2015;290(1):46–55. doi:https://doi.org/10.1074/jbc.M114.603589.
- Ratter JM, Rooijackers HMM, Hooiveld GJ, et al. In vitro and in vivo effects of lactate on metabolism and cytokine production of human primary PBMCs and monocytes. Front Immunol. 2018;9:2564. doi:https://doi.org/10.3389/fimmu.2018.02564.
- Machler P, Wyss MT, Elsayed M, et al. In vivo evidence for a lactate gradient from astrocytes to neurons. Cell Metab. 2016;23(1):94–102. doi:https://doi.org/10.1016/j.cmet.2015.10.010.
- Zhao Y, Zhao B, Wang X, et al. Macrophage transcriptome modification induced by hypoxia and lactate. Exp Ther Med. 2019;18(6):4811–4819. doi:https://doi.org/10.3892/etm.2019.8164.
- Liberti MV, Locasale JW. Histone lactylation: a new role for glucose metabolism. Trends Biochem Sci. 2020;45(3):179–182. doi:https://doi.org/10.1016/j.tibs.2019.12.004.
- Zhao P, Zhou W, Zhang Y, et al. Aminooxyacetic acid attenuates post-infarct cardiac dysfunction by balancing macrophage polarization through modulating macrophage metabolism in mice. J Cell Mol Med. 2020;24(4):2593–2609. doi:https://doi.org/10.1111/jcmm.14972.
- Latham T, Mackay L, Sproul D, et al. Lactate, a product of glycolytic metabolism, inhibits histone deacetylase activity and promotes changes in gene expression. Nucleic Acids Res. 2012;40(11):4794–4803. doi:https://doi.org/10.1093/nar/gks066.
- Motwani MP, Gilroy DW. Macrophage development and polarization in chronic inflammation. Semin Immunol. 2015;27(4):257–266. doi:https://doi.org/10.1016/j.smim.2015.07.002.
- Khatib-Massalha E, Bhattacharya S, Massalha H, et al. Lactate released by inflammatory bone marrow neutrophils induces their mobilization via endothelial GPR81 signaling. Nat Commun. 2020;11(1):3547. doi:https://doi.org/10.1038/s41467-020-17402-2.
- Liu C, Wu J, Zhu J, et al. Lactate inhibits lipolysis in fat cells through activation of an orphan G-protein-coupled receptor, GPR81. J Biol Chem. 2009;284(5):2811–2822. doi:https://doi.org/10.1074/jbc.M806409200.
- Brown TP, Bhattacharjee P, Ramachandran S, et al. The lactate receptor GPR81 promotes breast cancer growth via a paracrine mechanism involving antigen-presenting cells in the tumor microenvironment. Oncogene. 2020;39(16):3292–3304. doi:https://doi.org/10.1038/s41388-020-1216-5.
- Brown TP, Ganapathy V. Lactate/GPR81 signaling and proton motive force in cancer: role in angiogenesis, immune escape, nutrition, and Warburg phenomenon. Pharmacol Ther. 2020;206:107451. doi:https://doi.org/10.1016/j.pharmthera.2019.107451.
- Hoque R, Farooq A, Ghani A, Gorelick F, Mehal WZ. Lactate reduces liver and pancreatic injury in Toll-like receptor- and inflammasome-mediated inflammation via GPR81-mediated suppression of innate immunity. Gastroenterology. 2014;146(7):1763–1774. doi:https://doi.org/10.1053/j.gastro.2014.03.014.
- Madaan A, Nadeau-Vallee M, Rivera JC, et al. Lactate produced during labor modulates uterine inflammation via GPR81 (HCA1). Am J Obstet Gynecol. 2017;216:60 e1–e17.
- Chen P, Zuo H, Xiong H, et al. Gpr132 sensing of lactate mediates tumor-macrophage interplay to promote breast cancer metastasis. Proc Natl Acad Sci USA. 2017;114(3):580–585. doi:https://doi.org/10.1073/pnas.1614035114.
- Kim SY, Nair MG. Macrophages in wound healing: activation and plasticity. Immunol Cell Biol. 2019;97(3):258–267. doi:https://doi.org/10.1111/imcb.12236.
- Murray PJ. On macrophage diversity and inflammatory metabolic timers. Nat Rev Immunol. 2020;20(2):89–90. doi:https://doi.org/10.1038/s41577-019-0260-2.
- Locati M, Curtale G, Mantovani A. Diversity, mechanisms, and significance of macrophage plasticity. Annu Rev Pathol. 2020;15:123–147. doi:https://doi.org/10.1146/annurev-pathmechdis-012418-012718.
- Wang F, Zhang S, Jeon R, et al. Interferon gamma induces reversible metabolic reprogramming of M1 macrophages to sustain cell viability and pro-inflammatory activity. EBioMedicine. 2018;30:303–316. doi:https://doi.org/10.1016/j.ebiom.2018.02.009.
- Huang X, Li Y, Fu M, Xin HB. Polarizing macrophages in vitro. Methods Mol Biol. 2018;1784:119–126. doi:https://doi.org/10.1007/978-1-4939-7837-3_12.
- Galvan-Pena S, O’Neill LA. Metabolic reprograming in macrophage polarization. Front Immunol. 2014;5:420.
- Zhou D, Huang C, Lin Z, et al. Macrophage polarization and function with emphasis on the evolving roles of coordinated regulation of cellular signaling pathways. Cell Signal. 2014;26(2):192–197. doi:https://doi.org/10.1016/j.cellsig.2013.11.004.
- Tang PM, Nikolic-Paterson DJ, Lan HY. Macrophages: versatile players in renal inflammation and fibrosis. Nat Rev Nephrol. 2019;15(3):144–158. doi:https://doi.org/10.1038/s41581-019-0110-2.
- Ziemba AM, D’Amato AR, MacEwen TM, et al. Stabilized interleukin-4-loaded poly(lactic-co-glycolic) acid films shift proinflammatory macrophages toward a regenerative phenotype in vitro. ACS Appl Bio Mater. 2019;2(4):1498–1508. doi:https://doi.org/10.1021/acsabm.8b00769.
- Shapouri-Moghaddam A, Mohammadian S, Vazini H, et al. Macrophage plasticity, polarization, and function in health and disease. J Cell Physiol. 2018;233(9):6425–6440. doi:https://doi.org/10.1002/jcp.26429.
- Corna G, Campana L, Pignatti E, et al. Polarization dictates iron handling by inflammatory and alternatively activated macrophages. Haematologica. 2010;95(11):1814–1822. doi:https://doi.org/10.3324/haematol.2010.023879.
- Recalcati S, Locati M, Marini A, et al. Differential regulation of iron homeostasis during human macrophage polarized activation. Eur J Immunol. 2010;40(3):824–835. doi:https://doi.org/10.1002/eji.200939889.
- Prieur X, Roszer T, Ricote M. Lipotoxicity in macrophages: evidence from diseases associated with the metabolic syndrome. Biochim Biophys Acta. 2010;1801(3):327–337. doi:https://doi.org/10.1016/j.bbalip.2009.09.017.
- Engin AB. Adipocyte-macrophage cross-talk in obesity. Adv Exp Med Biol. 2017;960:327–343. doi:https://doi.org/10.1007/978-3-319-48382-5_14.
- Kawanishi N, Yano H, Yokogawa Y, Suzuki K. Exercise training inhibits inflammation in adipose tissue via both suppression of macrophage infiltration and acceleration of phenotypic switching from M1 to M2 macrophages in high-fat-diet-induced obese mice. exercise immunology review.
- Sica A, Erreni M, Allavena P, Porta C. Macrophage polarization in pathology. Cell Mol Life Sci. 2015;72(21):4111–4126. doi:https://doi.org/10.1007/s00018-015-1995-y.
- Schultze JL, Schmieder A, Goerdt S. Macrophage activation in human diseases. Semin Immunol. 2015;27(4):249–256. doi:https://doi.org/10.1016/j.smim.2015.07.003.
- Park J, Zhang Y, Saito E, et al. Intravascular innate immune cells reprogrammed via intravenous nanoparticles to promote functional recovery after spinal cord injury. Proc Natl Acad Sci USA. 2019;116(30):14947–14954. doi:https://doi.org/10.1073/pnas.1820276116.
- Xu H, Jiang J, Chen W, Li W, Chen Z. Vascular macrophages in atherosclerosis. J Immunol Res. 2019;2019:4354786. doi:https://doi.org/10.1155/2019/4354786.
- Tokutome M, Matoba T, Nakano Y, et al. Peroxisome proliferator-activated receptor-gamma targeting nanomedicine promotes cardiac healing after acute myocardial infarction by skewing monocyte/macrophage polarization in preclinical animal models. Cardiovasc Res. 2019;115(2):419–431. doi:https://doi.org/10.1093/cvr/cvy200.
- Chen X, Zhu X, Ma L, et al. A core-shell structure QRu-PLGA-RES-DS NP nanocomposite with photothermal response-induced M2 macrophage polarization for rheumatoid arthritis therapy. Nanoscale. 2019;11(39):18209–18223. doi:https://doi.org/10.1039/c9nr05922a.
- Ma C, Xia Y, Yang Q, Zhao Y. The contribution of macrophages to systemic lupus erythematosus. Clin Immunol. 2019;207:1–9. doi:https://doi.org/10.1016/j.clim.2019.06.009.
- Zhang W, Xu W, Xiong S. Blockade of Notch1 signaling alleviates murine lupus via blunting macrophage activation and M2b polarization. J Immunol. 2010;184(11):6465–6478. doi:https://doi.org/10.4049/jimmunol.0904016.
- Taylor CT, Doherty G, Fallon PG, Cummins EP. Hypoxia-dependent regulation of inflammatory pathways in immune cells. J Clin Invest. 2016;126(10):3716–3724. doi:https://doi.org/10.1172/JCI84433.
- Maedera S, Mizuno T, Ishiguro H, Ito T, Soga T, Kusuhara H. GLUT6 is a lysosomal transporter that is regulated by inflammatory stimuli and modulates glycolysis in macrophages. FEBS Lett. 2019;593(2):195–208. doi:https://doi.org/10.1002/1873-3468.13298.
- Braverman J, Stanley SA. Nitric oxide modulates macrophage responses to mycobacterium tuberculosis infection through activation of HIF-1α and repression of NF-κB. J Immunol. 2017;199(5):1805–1816. doi:https://doi.org/10.4049/jimmunol.1700515.
- Chiang C-F, Chao T-T, Su Y-F, et al. Metformin-treated cancer cells modulate macrophage polarization through AMPK-NF-κB signaling. Oncotarget 2017;8(13):20706–20718. doi:https://doi.org/10.18632/oncotarget.14982.
- Kanno Y, Ishisaki A, Kawashita E, Kuretake H, Ikeda K, Matsuo O. uPA Attenuated LPS-induced inflammatory osteoclastogenesis through the plasmin/PAR-1/Ca(2+)/CaMKK/AMPK Axis. Int J Biol Sci. 2016;12(1):63–71. doi:https://doi.org/10.7150/ijbs.12690.
- Dou G, Tian R, Liu X, et al. Chimeric apoptotic bodies functionalized with natural membrane and modular delivery system for inflammation modulation. Sci Adv. 2020;6(30):eaba2987. doi:https://doi.org/10.1126/sciadv.aba2987.
- Kim H, Kim BH, Huh BK, et al. Surgical suture releasing macrophage-targeted drug-loaded nanoparticles for an enhanced anti-inflammatory effect. Biomater Sci. 2017;5(8):1670–1677. doi:https://doi.org/10.1039/c7bm00345e.
- McMahan RS, Birkland TP, Smigiel KS, et al. Stromelysin-2 (MMP10) moderates inflammation by controlling macrophage activation. J Immunol. 2016;197(3):899–909. doi:https://doi.org/10.4049/jimmunol.1600502.
- Minutti CM, Jackson-Jones LH, Garcia-Fojeda B, et al. Local amplifiers of IL-4Rα-mediated macrophage activation promote repair in lung and liver. Science. 2017;356(6342):1076–1080. doi:https://doi.org/10.1126/science.aaj2067.
- Heung LJ, Hohl TM. Inflammatory monocytes are detrimental to the host immune response during acute infection with Cryptococcus neoformans. PLoS Pathog. 2019;15(3):e1007627. doi:https://doi.org/10.1371/journal.ppat.1007627.
- Tang T-T, Wang B, Wu M, et al. Extracellular vesicle-encapsulated IL-10 as novel nanotherapeutics against ischemic AKI. Sci Adv. 2020;6(33):eaaz0748. doi:https://doi.org/10.1126/sciadv.aaz0748.
- Zhu D, Johnson TK, Wang Y, et al. Macrophage M2 polarization induced by exosomes from adipose-derived stem cells contributes to the exosomal proangiogenic effect on mouse ischemic hindlimb. Stem Cell Res Ther. 2020;11(1):162. doi:https://doi.org/10.1186/s13287-020-01669-9.
- Zhao J, Li X, Hu J, et al. Mesenchymal stromal cell-derived exosomes attenuate myocardial ischaemia-reperfusion injury through miR-182-regulated macrophage polarization. Cardiovasc Res. 2019;115(7):1205–1216. doi:https://doi.org/10.1093/cvr/cvz040.
- Kratz M, Coats BR, Hisert KB, et al. Metabolic dysfunction drives a mechanistically distinct proinflammatory phenotype in adipose tissue macrophages. Cell Metab. 2014;20(4):614–625. doi:https://doi.org/10.1016/j.cmet.2014.08.010.
- Kim J, Bae JS. Tumor-associated macrophages and neutrophils in tumor microenvironment. Mediators Inflamm. 2016;2016:6058147. doi:https://doi.org/10.1155/2016/6058147.
- Chen Y, Song Y, Du W, Gong L, Chang H, Zou Z. Tumor-associated macrophages: an accomplice in solid tumor progression. J Biomed Sci. 2019;26(1):78. doi:https://doi.org/10.1186/s12929-019-0568-z.
- Riabov V, Gudima A, Wang N, Mickley A, Orekhov A, Kzhyshkowska J. Role of tumor associated macrophages in tumor angiogenesis and lymphangiogenesis. Front Physiol. 2014;5:75. doi:https://doi.org/10.3389/fphys.2014.00075.
- Alonso-Nocelo M, Raimondo TM, Vining KH, López-López R, de la Fuente M, Mooney DJ. Matrix stiffness and tumor-associated macrophages modulate epithelial to mesenchymal transition of human adenocarcinoma cells. Biofabrication. 2018;10(3):035004. doi:https://doi.org/10.1088/1758-5090/aaafbc.
- Noy R, Pollard JW. Tumor-associated macrophages: from mechanisms to therapy. Immunity. 2014;41(1):49–61. doi:https://doi.org/10.1016/j.immuni.2014.06.010.
- Sumitomo R, Hirai T, Fujita M, Murakami H, Otake Y, Huang CL. M2 tumor-associated macrophages promote tumor progression in non-small-cell lung cancer. Exp Ther Med. 2019;18:4490–4498. doi:https://doi.org/10.3892/etm.2019.8068.
- Steidl C, Lee T, Shah SP, et al. Tumor-associated macrophages and survival in classic Hodgkin’s lymphoma. N Engl J Med. 2010;362(10):875–885. doi:https://doi.org/10.1056/NEJMoa0905680.
- Shields CWt, Evans MA, Wang LL, et al. Cellular backpacks for macrophage immunotherapy. Sci Adv. 2020;6(18):eaaz6579. doi:https://doi.org/10.1126/sciadv.aaz6579.
- Kelly B, O’Neill LA. Metabolic reprogramming in macrophages and dendritic cells in innate immunity. Cell Res. 2015;25(7):771–784. doi:https://doi.org/10.1038/cr.2015.68.
- Kim J. Regulation of immune cell functions by metabolic reprogramming. J Immunol Res. 2018;2018:8605471. doi:https://doi.org/10.1155/2018/8605471.
- Palsson‐McDermott EM, O’Neill L. The Warburg effect then and now: from cancer to inflammatory diseases. BioEssays. 2013;35(11):965–973. doi:https://doi.org/10.1002/bies.201300084.
- Bailey JD, Diotallevi M, Nicol T, et al. Nitric oxide modulates metabolic remodeling in inflammatory macrophages through TCA cycle regulation and itaconate accumulation. Cell Rep. 2019;28(1):218–230 e7. doi:https://doi.org/10.1016/j.celrep.2019.06.018.
- Cheng SC, Quintin J, Cramer RA, et al. mTOR- and HIF-1α-mediated aerobic glycolysis as metabolic basis for trained immunity. Science 2014;345(6204):1250684. doi:https://doi.org/10.1126/science.1250684.
- Na YR, Je S, Seok SH. Metabolic features of macrophages in inflammatory diseases and cancer. Cancer Lett. 2018;413:46–58. doi:https://doi.org/10.1016/j.canlet.2017.10.044.
- O’Neill LA, Hardie DG. Metabolism of inflammation limited by AMPK and pseudo-starvation. Nature. 2013;493(7432):346–355. doi:https://doi.org/10.1038/nature11862.
- Griffiths HR, Gao D, Pararasa C. Redox regulation in metabolic programming and inflammation. Redox Biol. 2017;12:50–57. doi:https://doi.org/10.1016/j.redox.2017.01.023.
- Caslin HL, Abebayehu D, Abdul Qayum A, et al. Lactic acid inhibits lipopolysaccharide-induced mast cell function by limiting glycolysis and ATP availability. J Immunol. 2019;203(2):453–464. doi:https://doi.org/10.4049/jimmunol.1801005.
- Dietl K, Renner K, Dettmer K, et al. Lactic acid and acidification inhibit TNF secretion and glycolysis of human monocytes. J Immunol. 2010;184(3):1200–1209. doi:https://doi.org/10.4049/jimmunol.0902584.
- Costa Leite T, Da Silva D, Guimaraes Coelho R, Zancan P, Sola-Penna M. Lactate favours the dissociation of skeletal muscle 6-phosphofructo-1-kinase tetramers down-regulating the enzyme and muscle glycolysis. Biochem J. 2007;408(1):123–130. doi:https://doi.org/10.1042/BJ20070687.
- Yang K, Xu J, Fan M, et al. Lactate suppresses macrophage pro-inflammatory response to LPS stimulation by inhibition of YAP and NF-kappaB activation via GPR81-mediated signaling. Front Immunol. 2020;11:587913.
- Feng R, Morine Y, Ikemoto T, et al. Nrf2 activation drive macrophages polarization and cancer cell epithelial-mesenchymal transition during interaction. Cell Commun Signal. 2018;16(1):54. doi:https://doi.org/10.1186/s12964-018-0262-x.
- Ohashi T, Aoki M, Tomita H, et al. M2-like macrophage polarization in high lactic acid-producing head and neck cancer. Cancer Sci. 2017;108(6):1128–1134. doi:https://doi.org/10.1111/cas.13244.
- Kumar V. Targeting macrophage immunometabolism: dawn in the darkness of sepsis. Int Immunopharmacol. 2018;58:173–185. doi:https://doi.org/10.1016/j.intimp.2018.03.005.
- Kanmani P, Kim H. Protective effects of lactic acid bacteria against TLR4 induced inflammatory response in hepatoma HepG2 cells through modulation of toll-like receptor negative regulators of mitogen-activated protein kinase and NF-κB signaling. Front Immunol. 2018;9:1537. doi:https://doi.org/10.3389/fimmu.2018.01537.
- Peter K, Rehli M, Singer K, Renner-Sattler K, Kreutz M. Lactic acid delays the inflammatory response of human monocytes. Biochem Biophys Res Commun. 2015;457(3):412–418. doi:https://doi.org/10.1016/j.bbrc.2015.01.005.
- Iraporda C, Romanin DE, Bengoa AA, et al. Local treatment with lactate prevents intestinal inflammation in the TNBS-induced colitis model. Front Immunol. 2016;7:651.
- Iraporda C, Errea A, Romanin DE, et al. Lactate and short chain fatty acids produced by microbial fermentation downregulate proinflammatory responses in intestinal epithelial cells and myeloid cells. Immunobiology. 2015;220(10):1161–1169. doi:https://doi.org/10.1016/j.imbio.2015.06.004.
- Hou Q, Ye L, Liu H, et al. Lactobacillus accelerates ISCs regeneration to protect the integrity of intestinal mucosa through activation of STAT3 signaling pathway induced by LPLs secretion of IL-22. Cell Death Differ. 2018;25(9):1657–1670. doi:https://doi.org/10.1038/s41418-018-0070-2.
- Lemme-Dumit JM, Polti MA, Perdigon G, Galdeano CM. Probiotic bacteria cell walls stimulate the activity of the intestinal epithelial cells and macrophage functionality. Benef Microbes. 2018;9(1):153–164. doi:https://doi.org/10.3920/BM2016.0220.
- Sun Z, Han Y, Song S, Chen T, Han Y, Liu Y. Activation of GPR81 by lactate inhibits oscillatory shear stress-induced endothelial inflammation by activating the expression of KLF2. IUBMB Life. 2019;71(12):2010–2019. doi:https://doi.org/10.1002/iub.2151.
- Zhang W, Wang G, Xu ZG, et al. Lactate is a natural suppressor of RLR signaling by targeting MAVS. Cell 2019;178(1):176–189 e15. doi:https://doi.org/10.1016/j.cell.2019.05.003.
- Notarangelo G, Haigis MC. Sweet temptation: from sugar metabolism to gene regulation. Immunity. 2019;51(6):980–981. doi:https://doi.org/10.1016/j.immuni.2019.11.008.
- Pavlova NN, Thompson CB. The emerging hallmarks of cancer metabolism. Cell Metab. 2016;23(1):27–47. doi:https://doi.org/10.1016/j.cmet.2015.12.006.
- Pertega-Gomes N, Felisbino S, Massie CE, et al. A glycolytic phenotype is associated with prostate cancer progression and aggressiveness: a role for monocarboxylate transporters as metabolic targets for therapy. J Pathol. 2015;236(4):517–530. doi:https://doi.org/10.1002/path.4547.
- Chen F, Chen J, Yang L, et al. Extracellular vesicle-packaged HIF-1α-stabilizing lncRNA from tumour-associated macrophages regulates aerobic glycolysis of breast cancer cells. Nat Cell Biol. 2019;21(4):498–510. doi:https://doi.org/10.1038/s41556-019-0299-0.
- Huang M, Chen Q, Xiao J, et al. Overexpression of hypoxia-inducible factor-1α is a predictor of poor prognosis in cervical cancer: a clinicopathologic study and a meta-analysis. Int J Gynecol Cancer. 2014;24(6):1054–1064. doi:https://doi.org/10.1097/IGC.0000000000000162.
- Zhu CL, Huang Q, Liu CH, Lin XS, Xie F. Prognostic value of HIF-1α expression in patients with gastric cancer. Mol Biol Rep. 2013;40(11):6055–6062. doi:https://doi.org/10.1007/s11033-013-2715-z.
- Zhang J, Wu Y, Lin YH, et al. Prognostic value of hypoxia-inducible factor-1 alpha and prolyl 4-hydroxylase beta polypeptide overexpression in gastric cancer. WJG. 2018;24(22):2381–2391. doi:https://doi.org/10.3748/wjg.v24.i22.2381.
- Cai FF, Xu C, Pan X, et al. Prognostic value of plasma levels of HIF-1a and PGC-1a in breast cancer. Oncotarget. 2016;7(47):77793–77806. doi:https://doi.org/10.18632/oncotarget.12796.
- Li Y, Li X, Kan Q, et al. Mitochondrial pyruvate carrier function is negatively linked to Warburg phenotype in vitro and malignant features in esophageal squamous cell carcinomas. Oncotarget. 2017;8(1):1058–1073. doi:https://doi.org/10.18632/oncotarget.13717.
- Wang JX, Choi SYC, Niu X, et al. Lactic acid and an acidic tumor microenvironment suppress anticancer immunity. Int J Mol Sci. 2020;21:8363.
- Colegio OR, Chu NQ, Szabo AL, et al. Functional polarization of tumour-associated macrophages by tumour-derived lactic acid. Nature 2014;513(7519):559–563. doi:https://doi.org/10.1038/nature13490.
- Mu X, Shi W, Xu Y, et al. Tumor-derived lactate induces M2 macrophage polarization via the activation of the ERK/STAT3 signaling pathway in breast cancer. Cell Cycle. 2018;17(4):428–438. doi:https://doi.org/10.1080/15384101.2018.1444305.
- Locatelli SL, Careddu G, Serio S, et al. Targeting cancer cells and tumor microenvironment in preclinical and clinical models of hodgkin lymphoma using the dual PI3Kδ/γ inhibitor RP6530. Clin Cancer Res. 2019;25(3):1098–1112. doi:https://doi.org/10.1158/1078-0432.CCR-18-1133.
- Feng J, Yang H, Zhang Y, et al. Tumor cell-derived lactate induces TAZ-dependent upregulation of PD-L1 through GPR81 in human lung cancer cells. Oncogene 2017;36(42):5829–5839. doi:https://doi.org/10.1038/onc.2017.188.
- Liu N, Luo J, Kuang D, et al. Lactate inhibits ATP6V0d2 expression in tumor-associated macrophages to promote HIF-2α-mediated tumor progression . J Clin Invest. 2019;129(2):631–646. doi:https://doi.org/10.1172/JCI123027.
- El-Kenawi A, Gatenbee C, Robertson-Tessi M, et al. Acidity promotes tumour progression by altering macrophage phenotype in prostate cancer. Br J Cancer. 2019;121(7):556–566. doi:https://doi.org/10.1038/s41416-019-0542-2.
- Mendler AN, Hu B, Prinz PU, Kreutz M, Gottfried E, Noessner E. Tumor lactic acidosis suppresses CTL function by inhibition of p38 and JNK/c-Jun activation. Int J Cancer. 2012;131(3):633–640. doi:https://doi.org/10.1002/ijc.26410.
- Fullerton JN, Gilroy DW. Resolution of inflammation: a new therapeutic frontier. Nat Rev Drug Discov. 2016;15(8):551–567. doi:https://doi.org/10.1038/nrd.2016.39.
- Pucino V, Bombardieri M, Pitzalis C, Mauro C. Lactate at the crossroads of metabolism, inflammation, and autoimmunity. Eur J Immunol. 2017;47(1):14–21. doi:https://doi.org/10.1002/eji.201646477.
- Van Welden S, De Vos M, Wielockx B, et al. Haematopoietic prolyl hydroxylase-1 deficiency promotes M2 macrophage polarization and is both necessary and sufficient to protect against experimental colitis. J Pathol. 2017;241(4):547–558. doi:https://doi.org/10.1002/path.4861.
- Cummins EP, Seeballuck F, Keely SJ, et al. The hydroxylase inhibitor dimethyloxalylglycine is protective in a murine model of colitis. Gastroenterology 2008;134(1):156–165. e1. doi:https://doi.org/10.1053/j.gastro.2007.10.012.
- Besnier E, Coquerel D, Kouadri G, et al. Hypertonic sodium lactate improves microcirculation, cardiac function, and inflammation in a rat model of sepsis. Crit Care. 2020;24(1):354. doi:https://doi.org/10.1186/s13054-020-03083-2.
- Okada T, Fukuda S, Hase K, et al. Microbiota-derived lactate accelerates colon epithelial cell turnover in starvation-refed mice. Nat Commun. 2013;4:1654. doi:https://doi.org/10.1038/ncomms2668.
- Polański R, Hodgkinson CL, Fusi A, et al. Activity of the monocarboxylate transporter 1 inhibitor AZD3965 in small cell lung cancer. Clin Cancer Res. 2014;20(4):926–937. doi:https://doi.org/10.1158/1078-0432.CCR-13-2270.
- Ždralević M, Vučetić M, Daher B, Marchiq I, Parks SK, Pouysségur J. Disrupting the ‘Warburg effect’ re-routes cancer cells to OXPHOS offering a vulnerability point via ‘ferroptosis’-induced cell death. Adv Biol Regul. 2018;68:55–63. doi:https://doi.org/10.1016/j.jbior.2017.12.002.
- Chung T-W, Kim E-Y, Han CW, et al. Machilin A inhibits tumor growth and macrophage M2 polarization through the reduction of lactic acid. Cancers. 2019;11(7):963. doi:https://doi.org/10.3390/cancers11070963.
- Chen QW, Wang JW, Wang XN, et al. Inhibition of tumor progression through the coupling of bacterial respiration with tumor metabolism. Angew Chem Int Ed Engl. 2020;59(48):21562–21570. doi:https://doi.org/10.1002/anie.202002649.
- Yao J, Du Z, Li Z, et al. 6-Gingerol as an arginase inhibitor prevents urethane-induced lung carcinogenesis by reprogramming tumor supporting M2 macrophages to M1 phenotype. Food Funct. 2018;9(9):4611–4620. doi:https://doi.org/10.1039/c8fo01147h.
- Daneshmandi S, Wegiel B, Seth P. Blockade of lactate dehydrogenase-A (LDH-A) improves efficacy of anti-programmed cell death-1 (PD-1) therapy in melanoma. Cancers (Basel) 2019;11(4):450. doi:https://doi.org/10.3390/cancers11040450.
- Gao F, Tang Y, Liu WL, et al. Intra/extracellular lactic acid exhaustion for synergistic metabolic therapy and immunotherapy of tumors. Adv Mater. 2019;31(51):e1904639. doi:https://doi.org/10.1002/adma.201904639.
- Li N, Kang Y, Wang L, et al. ALKBH5 regulates anti-PD-1 therapy response by modulating lactate and suppressive immune cell accumulation in tumor microenvironment. Proc Natl Acad Sci USA. 2020;117(33):20159–20170. doi:https://doi.org/10.1073/pnas.1918986117.