Abstract
Background
Astragalus polysaccharide (APS) is a major bioactive component of the Chinese herb astragalus, with well-established protective effects on the kidney. However, the effect of APS on diabetic nephropathy (DN) is unclear.
Methods
Long non-coding RNA (lncRNA) expression profiles in kidney samples from control, db/db, and APS-treated db/db mice were evaluated using RNA high-throughput sequencing techniques. Additionally, rat renal tubular epithelial (NRK-52E) cells were cultured in high glucose (HG) media. We inhibited the expression of Gm41268 and prolactin receptor (PRLR) by transfecting NRK-52E cells with Gm41268-targeting antisense oligonucleotides and PRLR siRNA.
Results
We found that APS treatment reduced 24-h urinary protein levels and fasting blood glucose and improved glucose intolerance and pathological renal damage in db/db mice. Furthermore, APS treatment enhanced autophagy and alleviated fibrosis in the db/db mice. We identified a novel lncRNA, Gm41268, which was differentially expressed in the three groups, and the cis-regulatory target gene PRLR. APS treatment induced autophagy by reducing p62 and p-mammalian target of rapamycin (mTOR) protein levels and increasing the LC3 II/I ratio. Furthermore, APS alleviated fibrosis by downregulating fibronectin (FN), transforming growth factor-β (TGF-β), and collagen IV levels. In addition, APS reversed the HG-induced overexpression of Gm41268 and PRLR. Reduction of Gm41268 decreased PRLR expression, restored autophagy, and ameliorated renal fibrosis in vitro. Inhibition of PRLR could enhance the protective effect of APS.
Conclusions
In summary, we demonstrated that the therapeutic effect of APS on DN is mediated via the Gm41268/PRLR pathway. This information contributes to the exploration of bioactive constituents in Chinese herbs as potential treatments for DN.
1. Introduction
Diabetic nephropathy (DN), a common complication of diabetes and the primary cause of end-stage kidney disease [Citation1], is characterized by pathological changes such as glomerular mesangial expansion and hypertrophy [Citation2]. The global prevalence of diabetes in 2021 is estimated to be approximately 10.5%, suggesting that it affects over 536.6 million people and is predicted to rise to 12.2% by 2045 [Citation3,Citation4]. The increasing prevalence of DN worldwide poses a considerable burden to patients and their families. DN is linked to metabolic changes caused by hyperglycemia that lead to renal fibrosis [Citation5]. Hyperglycemia is associated with renal inflammation, apoptosis, and oxidative stress, all of which contribute to DN progression [Citation6–8]. Several studies have identified the active ingredients and molecular mechanisms of traditional Chinese medicine (TCM) herbs that have hypoglycemic and renoprotective effects [Citation9,Citation10].
There are 2000 species of Astragalus worldwide. Astragalus, a Chinese herb also known as Huang Qi, originates from the root of Astragalus membranaceus (Fisch.) Bge. var. mongholicus (Bge.) Hsiao, Astragalus membranaceus (Fisch.) Bge. It is included in the Chinese Pharmacopoeia [Citation11] and has been widely used in TCM for over 2000 years. According to TCM theory, astragalus can reinforce Qi, facilitate urination, and enhance body repair capacity. Astragalus has been reported to have a therapeutic effect on type 2 diabetes [Citation12]. A Cochrane review suggested that astragalus offers promising effects in reducing proteinuria and increasing hemoglobin and serum albumin levels in chronic kidney disease [Citation13]. Several meta-analyses have demonstrated its efficacy in DN [Citation14,Citation15]. Astragalus contains more than 200 compounds, including flavonoids, saponins, and polysaccharides [Citation16]. Polysaccharides are major components that play an important role in the treatment of various diseases. Recently, the therapeutic effects of Astragalus polysaccharide (APS) have been reported to be beneficial for many diseases, such as cardiovascular disease, diabetes, and kidney and digestive diseases [Citation17,Citation18]. However, few studies have investigated the effects of APS on DN. APS was found to lower blood glucose and protect kidney function by affecting the transforming growth factor-β (TGF-β)/Smad signaling pathway in diabetic rats [Citation19]. In addition, APS has been shown to improve early DN by affecting the mRNA expression levels of NF-κB and IκB [Citation20]. However, these studies have mainly focused on inflammatory and fibrotic signaling pathways. The molecular mechanisms underlying the protective effects of APS against DN remain unclear and require further study.
Long non-coding RNAs (lncRNAs) are RNA transcripts that exceed 200 nucleotides in length. Numerous reports have confirmed that lncRNAs play mechanistic and functional roles in gene expression and RNA metabolism under both physiological and pathological conditions [Citation21]. It has been shown that lncRNAs are associated with DN and, therefore, could be key factors in diabetes and diabetic renal disease. Further knowledge of their activities could provide beneficial insights into the diagnosis, prevention, and treatment of DN [Citation22]. A growing number of studies have demonstrated that herbal extracts can regulate the expression of lncRNAs [Citation23]. For example, the active ingredient of Paris polyphylla Smith showed anticancer properties in nasopharyngeal carcinoma by regulating lncRNA ROR levels [Citation24], whereas β-elemene, a compound found in the herb Curcuma wenyujin Y. H. Chen & C. Ling, acted as an antineoplastic inhibitor of disseminated large B-cell lymphoma via the lncRNA HULC pathway [Citation25].
RNA sequencing (RNA-Seq) is a powerful tool commonly used for the analysis of gene expression and identification of new RNA species [Citation26]. In this study, we used RNA-seq to explore the effects of APS on lncRNA expression in DN. To elucidate the mechanism of APS action in a diabetic mouse model, we evaluated the differential expression of lncRNAs in non-diabetic (db/m), type 2 diabetic (db/db), and APS-treated db/db mice. The moderating effect of APS on novel lncRNAs was confirmed in vitro.
2. Materials and methods
2.1. Animals
The db/db and db/m mice were purchased from the Model Animal Research Center of Nanjing University (Nanjing, China). All the mice were 4–6-weeks old. The mice were randomly divided into three groups: db/m (control group, CR, n = 6), db/db (model group, MD, n = 6), and db/db + APS (APS, n = 5). Mice in the APS group were fed a diet containing 2 g/kg APS, whereas those in the CR and MD groups were fed a regular diet. APS with purity greater than 98% was purchased from Macklin (#A860847, Shanghai, China). All mice were kept in a laboratory with a 12/12-h light/dark cycle. After a 12-week treatment, the weight, 24-h urinary protein levels, fasting blood glucose, and glucose tolerance (using an oral glucose tolerance test (OGTT)) of the mice were measured. Blood glucose levels were measured using a glucometer (Roche Diabetes Care GmbH, Basel, Switzerland). Mouse tissues were frozen in liquid nitrogen for further analysis.
2.2. Cell culture and transfection
NRK-52E cells were obtained from American Tissue Culture Collection (ATCC; Manassas, VA). The cells were cultured in complete Dulbecco’s modified Eagle’s medium (DMEM; HyClone, South Logan, UT) containing 5% fetal bovine serum (FBS; Invitrogen, Carlsbad, CA) at 37 °C in a 5% CO2 incubator. Upon reaching approximately 70–80% confluence, the cells were divided into five groups: normal glucose (NG), high glucose (HG), low concentration of APS (50APS), medium concentration of APS (300APS), and high concentration of APS (500APS). Cells in the HG group were cultured in DMEM containing HG (30 mmol/L glucose) and 5% FBS for 48 h. Cells in the 50APS, 300APS, and 500APS groups were cultured in DMEM containing 5% FBS with HG plus 50, 300, or 500 μg/mL APS for 48 h, respectively. Cell viability was analyzed using the Cell Counting Kit-8 (Dojindo Laboratories, Kyushu, Japan), following the manufacturer’s instructions. The cells were seeded in 96-well culture plates at a density of 5000 cells/well. After 48 h of culture, 100 μL of cell counting kit-8 solution was added to each well and the cells were incubated for 2 h. The absorbance was measured using a microplate reader (BioTek Instruments, Winooski, VT).
The antisense oligonucleotide (ASO) control and Gm41268-targeting ASO were acquired from RiboBio (Guangzhou, China). To inhibit the expression of Gm41268, cells were transfected with Gm41268-targeting ASOs according to the manufacturer’s instructions. The PRLR siRNA and negative control (NC) vectors were synthesized by Guangzhou Ruibo Biological Co., Ltd. (Guangdong, China).
2.3. Urine biochemistry assays and OGTT
Urinary albumin levels were determined using a mouse urinary albumin ELISA kit (Bethyl Laboratories, Montgomery, TX). OGTT was performed at 4, 8, and 12 weeks. All mice were prepped by oral administration of a glucose solution (2.0 g/kg) after 6 h of fasting. Blood glucose levels were evaluated at 0, 30, 60, 90, and 120 min. The area under the curve (AUC) was plotted to enhance visualization of the OGTT data.
2.4. Histological analysis
The renal samples were fixed in 4% paraformaldehyde for 1–2 h. After paraffin embedding, the samples were cut into 5-μm thick slices. The sections were stained with hematoxylin and eosin (H&E), periodic acid-Schiff (PAS), and Masson’s trichrome. High-resolution images were acquired using a microscope (Olympus, Shinjuku City, Japan). Image-Pro Plus software (version 6.0; Media Cybernetics, Bethesda, MD) was used to process images. The mesangial expansion index was defined as the percentage of the positive area of the entire glomerulus. The collagen fibril area ratio was defined as the proportion of blue areas, according to Masson’s trichrome staining.
2.5. RNA-Seq analysis
Total RNA was extracted from the renal cortex of the mice using the TRIzol kit (Invitrogen, Carlsbad, CA). Ribosomal RNA was eliminated using the Ribo-Zero kit (Epicenter, Madison, WI), and the RNA was cleaved into fragments of approximately 200 nt. Using the NEBNext® Ultra™ RNA Library Prep Kit for Illumina (NEB, Ipswich, MA), single- and double-stranded cDNA was synthesized via reverse transcription and purified. Next, the ends were repaired, and primers were added. The libraries were validated using an Illumina HiSeq 3000 platform (RiboBio, Guangzhou, China).
2.6. Differentially expressed lncRNA
The reads were compared with the frame of the reference genome using the HISAT2 program. The location of the genome corresponding to all reads was then counted. The sample expression level was assessed using RPKM. The differential expression levels of RNAs were identified according to fold change (|log2(fold change)| > 1) and significance level (p < .05).
2.7. Kyoto encyclopedia of genes and genomes (KEGG) pathway and gene ontology (GO) analysis
GO analysis was used to determine the central functions of the differentially regulated lncRNAs (p < .05). KEGG pathway analysis was performed to obtain pathway clusters, including differentially expressed lncRNAs, in molecular interaction networks.
2.8. Quantitative reverse transcription PCR (qRT-PCR)
Total RNA was eluted as described above and reverse-transcribed using the PrimeScript™ RT reagent kit (Cat. RR047A; Takara Bio, Kusatsu, Japan). Real-time PCR was performed using SYBR Green Master Mix and a CFX96 PCR System (Bio-Rad, Hercules, CA). Gene expression was normalized to that of GAPDH, an internal control. The primers used are shown in Table S1.
2.9. Western blotting
Total protein was isolated from tissues and cells using RIPA buffer. The protein concentration was determined using a bicinchoninic acid protein assay kit (Pierce, Rockford, IL). The protein concentrations of the different samples were adjusted accordingly. Next, the proteins were separated using 8–10% SDS-PAGE gels and transferred onto polyvinylidene difluoride membranes. The membranes were blocked with 5% skim milk in TBS-T for 1.5 h and incubated overnight at 4 °C with a primary antibody. The following primary antibodies were used: anti-mammalian target of rapamycin (mTOR) (1:1000), anti-FN (1:1000), anti-PRLR (1:1000), anti-LC3 (1:1000), anti-TGF-β (1:1000), anti-collagen IV (1:1000), anti-p62 (1:1000), and anti-GAPDH (1:1500). The membranes were then incubated with secondary antibodies for 2 h. Proteins were detected using an enhanced chemiluminescence chromogenic substrate (Thermo Fisher Scientific, Waltham, MA) and visualized using a chemiluminescent gel imaging system (Bio-Rad, Hercules, CA).
2.10. Immunofluorescence
NRK-52E cells were seeded uniformly into six-well plates lined with slides. The slides were fixed with 4% paraformaldehyde for 20 min, and the slides were washed by dipping with 1 × PBS three times for 3 min each time. Five percent FBS was added to the slide and closed at room temperature for 1 h. Each slide was incubated overnight at 4 °C with primary antibody anti-PRLR (1:200). Secondary antibodies with fluorescence were added and incubated at 37 °C for 1 h. DAPI was dropped and incubated in the dark for 5 min. The slides were sealed with mounting solution containing anti-fluorescence quencher, and images were observed and collected under a fluorescence microscope. Image-Pro Plus software (version 6.0; Media Cybernetics, Bethesda, MD) was used to detect the fluorescence intensity.
2.11. Transmission electron microscopy
NRK-52E cells were collected by centrifugation at 1200 rpm for 3 min. The cells were fixed with 2.5% glutaraldehyde overnight at 4 °C and washed with PBS. Samples were dehydrated using a graded ethanol series (50–100%) and then embedded. Ultrathin 90-nm sections were stained with 2% uranyl and visualized using a 120 kV JEOL electron microscope (Akishima, Japan) equipped with a Gatan camera. Ten fields of view in the electron micrographs of the different groups were used to count autophagosomes.
2.12. Statistical analyses
Experiments were performed three times, and the results are presented as the mean ± SD. All data were evaluated for significant differences using SPSS (IBM, Armonk, NY) and GraphPad Prism 8.0 (GraphPad Software, San Diego, CA) software. Multiple comparisons were assessed using one-way ANOVA, and significance was set at p < .05.
3. Results
3.1. APS improves renal injury in the db/db mouse model
To determine the effect of APS treatment on DN, we evaluated body weight, 24-h urinary protein levels, fasting blood glucose concentrations, and glucose tolerance in each group. The increase in body weight was faster in the MD group than in the CR group; however, no significant differences in body weight were observed between the APS and MD groups (). Compared with those in the CR group, 24-h urinary protein levels, fasting blood glucose, and the AUC of the OGTT were significantly increased in the MD group. Conversely, 24-h urinary protein levels, fasting blood glucose levels, and AUC at week 12 were significantly lower in the APS group than in the MD group (). Next, we evaluated the renal pathology of the three groups using H&E, PAS, and Masson’s trichrome staining. We observed pathological changes in the kidneys of db/db mice including glomerular mesangial enlargement, basement membrane thickening, and increased nephron fibrosis. Interstitial fibrosis was also observed in the renal tubule area (). After APS treatment, pathological changes improved substantially. Our results indicated that APS reduced 24-h urinary protein and fasting blood glucose levels, improved glucose tolerance, and reduced pathological damage to the kidneys.
Figure 1. Astragalus polysaccharide (APS) improves renal lesions in db/db mice. (A) The body weights of all mice measured weekly (n = 5 or 6). (B) Analysis of 24-h urine for the detection of albumin (n = 5). (C–H) AUC results for the OGTTs at weeks 4, 8, and 12 (n = 5 or 6). (I) Pathological changes in the kidneys of mice in different groups evaluated by hematoxylin–eosin (H&E), periodic acid-Schiff (PAS) and Masson’s trichrome staining. (J) Quantifications of PAS and Masson’s trichrome staining. Data are presented as the mean ± SD. *p < .05; **p < .001.
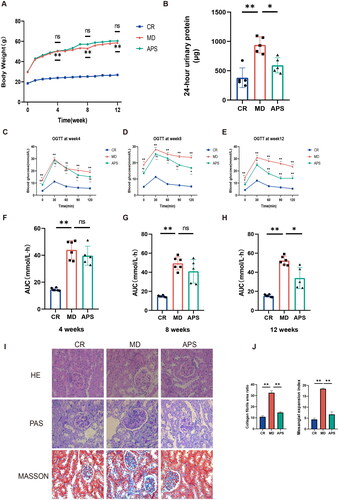
3.2. Differentially expressed lncRNAs in the three groups
Three mice from each group were randomly selected for RNA-seq analysis to further investigate the molecular mechanism of APS in DN. Bioinformatic data from all three groups were compared, and volcano plots demonstrated the differential expression of lncRNAs. We observed 569 lncRNAs that were upregulated and 318 lncRNAs that were downregulated in the CR and MD groups (), whereas 9 lncRNAs were upregulated and 20 lncRNAs were downregulated in the MD vs. APS groups (). Based on the RNA-seq data, a Venn diagram was generated to investigate possible relationships between the CR vs. MD and MD vs. APS groups. The results suggested that only 11 lncRNAs were differentially expressed between the two sets (). To further investigate these findings, heatmaps showed that the expression of the 11 lncRNAs was markedly different in the CR vs. the MD groups and this effect was reversed by the APS treatment (). Among these 11 lncRNAs, Gm41268 expression was the most significantly different. These results indicate that APS affected the expression of 11 lncRNAs, especially Gm41268.
Figure 2. Differential expression of long non-coding RNAs (lncRNAs) in the three experimental groups (n = 3). (A, B) Volcano plots comparing lncRNAs expressed in the CR vs. the MD and the MD vs. the APS groups. (C) A Venn diagram comparing significantly different differentially expressed lncRNAs from the CR vs. the MD groups and the MD vs. the APS groups. (D) A heatmap comparing the expression of 11 lncRNAs between the CR, MD, and APS groups. The results of GO analysis are represented by bubble plots (E) and the results of KEGG analysis are represented by histograms (F); both plots were used for the enrichment analysis of differentially expressed lncRNAs.
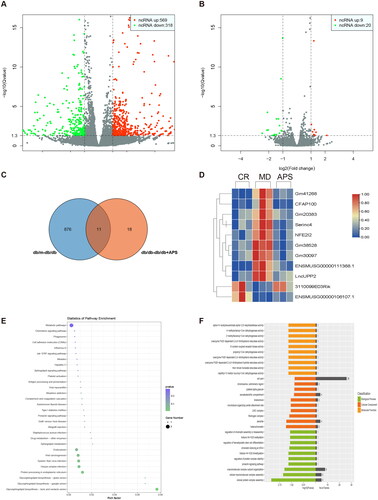
Next, we predicted the potential target genes of the 11 lncRNAs using bioinformatic analysis. GO assays demonstrated that the target genes were involved in regulating chromatin assembly or disassembly, regulation of protein complex stability, the prolactin signaling pathway, and others (). Based on KEGG analysis, these genes were primarily enriched in endocytosis and metabolic pathways ().
3.3. APS regulates Gm41268/PRLR and ameliorates autophagy and renal fibrosis in db/db mice
Based on RNA-seq, GO, and KEGG analyses, we hypothesized that lncRNA Gm41268 expression played a key role in mediating the effects of APS treatment. PCR results showed that Gm41268 was highly expressed in the MD group compared to the CR group, and this effect could be reversed by APS treatment (). To explore the mechanism by which Gm41268 regulates DN, the target genes of Gm41268 were obtained by integrating the differential expression of mRNA data (<10 kb) in RNA-seq (Table S2). The Ensembl database was used to investigate possible targets of Gm41268. Interestingly, the prolactin receptor (PRLR) was identified as a potential target of Gm41268. Several studies have indicated that PRLR is an essential gene that is involved in numerous pathways. For example, PRL/PRLR activates the PI3K/AKT signaling pathway [Citation27], which has been demonstrated to affect DN through its regulatory influence on autophagy [Citation28]. Western blotting experiments showed that PRLR expression was higher in the MR group than in the CR group, whereas APS treatment reduced PRLR expression levels when compared with that in the MR group (). Next, we evaluated the expression levels of autophagy-related proteins such as mTOR, LC3 II/I, and p62 (). While the protein levels of p-mTOR and p62 were upregulated, the LC3 II/I ratio decreased in the MD group. In contrast, p-mTOR and p62 levels were decreased and the LC3 II/I ratio was increased in the APS group compared to those in the MD group. To further study the mechanism of action of APS, protein markers related to renal fibrosis were investigated (). The protein levels of TGF-β, collagen IV, and fibronectin (FN) were substantially higher in the MD group than in the CR group. The expression of these proteins was downregulated in the APS group. These results revealed that APS induces autophagy and alleviates renal fibrosis via the Gm41268/PRLR axis in vivo.
Figure 3. APS regulates Gm41268/prolactin receptor (PRLR) and ameliorates autophagy and renal fibrosis in db/db mice. (A) Validation of Gm41268 expression by qRT-PCR (n = 3). (B, C) PRLR protein levels were detected by western blotting; representative blots and densitometry results are shown (n = 3). (D–G) Protein markers of autophagy were detected by western blotting; representative blots and densitometry results are shown (n = 3). (H–K) Proteins associated with fibrosis were evaluated by western blotting; representative blots and densitometry results are shown (n = 3). Data are presented as the mean ± SD. *p < .05; **p < .001.
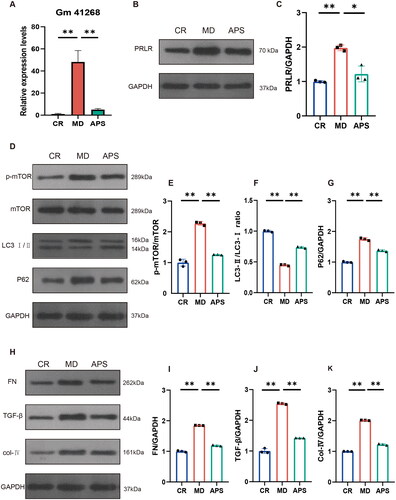
3.4. APS inhibited Gm41268/PRLR and alleviated fibrosis in HG-treated NRK-52E cells
To confirm the protective effects of APS, NRK-52E cells were cultured in HG media. The viability of cells cultured with different APS concentrations was evaluated using the CCK-8 assay (). The results indicated that cell viability was the highest in the 500 APS group. Next, we explored the effects of APS at 50, 300, and 500 μg/mL on the expression of Gm41268 and PRLR. The results demonstrated that Gm41268 and PRLR expression was substantially increased in HG-treated cells compared to NG-cultured cells. APS treatment downregulated the expression of Gm41268 and PRLR (). As APS appeared to alleviate renal fibrosis in vivo, we investigated the effects of HG and APS on fibrosis in vitro. PCR results showed that TGF-β expression was lower in the NG group than in the HG group, whereas APS treatment reduced TGF-β levels (). For further verification, TGF-β, FN, and collagen IV levels were evaluated using western blotting (). The expression of these proteins was substantially upregulated in the HG group and markedly decreased at each APS concentration tested. These results suggest that APS has an antifibrotic effect in vitro.
Figure 4. APS inhibits Gm41268/PRLR and alleviates fibrosis in HG-treated NRK-52E cells. (A) NRK-52E cells were treated with HG or HG plus APS at different concentrations for 48 h. Cell viability was measured using a Cell Counting Kit-8. (B–D) Validation of Gm41268, PRLR, TGF-β expression by qRT-PCR (n = 3). (E–I) Expression of proteins associated with fibrosis was evaluated by western blotting; representative blots and densitometry results are shown (n = 3). Data are presented as the mean ± SD. *p < .05; **p < .001.
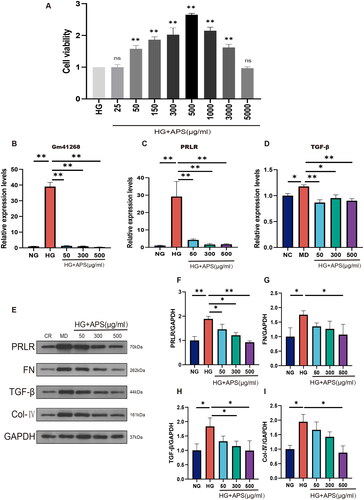
3.5. APS reverses the inhibition of autophagy induced by HG in NRK-52E cells
Compared to the NG group, the protein levels of p-mTOR and p62 were increased in the HG group, whereas APS treatment reduced p-mTOR and p62 levels (,D)). The LC3 II/I ratio, a key autophagy marker, was measured using western blotting. Our results showed that the LC3 II/I ratio was significantly lower in the HG group, whereas treatment with 50, 300, and 500 μg/mL APS increased this ratio (). Furthermore, the number of autophagosomes was reduced in the HG group and increased in the APS group (). These results indicated that APS treatment reversed the HG-induced inhibition of autophagy in NRK-52E cells.
Figure 5. APS reverses the inhibition of autophagy induced by high glucose in NRK-52E cells. (A–E) NRK-52E cells were treated with HG or HG plus APS at different concentrations for 48 h. Expression of protein markers associated with autophagy was evaluated by western blotting; representative blots and densitometry results are shown (n = 3). (F) Autophagosomes/autolysosomes were evaluated by transmission electron microscopy; scale bar = 1 μm. The arrows point to the autophagic vacuoles. Data are presented as the mean ± SD. *p < .05; **p < .001.
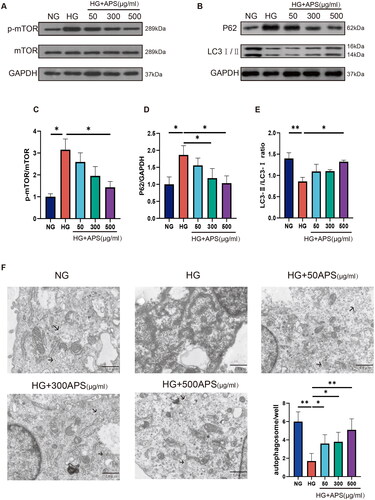
3.6. Inhibition of lncRNA Gm41268 reduces fibrosis and promotes autophagy in NRK-52E cells cultured in HG
To further investigate the role of lncRNA Gm41268 in autophagy and fibrosis in NRK-52E cells, we transfected NRK-52E cells with Gm41268-targeting ASOs. As expected, Gm41268 expression decreased. Furthermore, PRLR expression was down-regulated (). In addition, PCR results showed that TGF-β expression decreased after downregulation of Gm41268 (). Western blotting demonstrated that the protein levels of PRLR, FN, p62, TGF-β, p-mTOR, and collagen IV were also reduced, whereas the LC3 II/I ratio increased in response to Gm41268 downregulation (). These findings suggest that Gm41268 is involved in fibrosis progression and autophagy inhibition in HG-induced NRK-52E cells.
Figure 6. Inhibition of lncRNA Gm41268 expression reduces fibrosis and promotes autophagy. (A–C) Validation of Gm41268, PRLR, and TGF-β expression by qRT-PCR (n = 3). (D–H) The expression of PRLR and the protein associated with fibrosis was evaluated by western blotting; representative blots and densitometry results are shown (n = 3). (I–L) Expression of autophagy-related proteins was evaluated by western blotting; representative blots and their quantification are shown (n = 3). Data are presented as the mean ± SD. *p < .05; **p < .001.
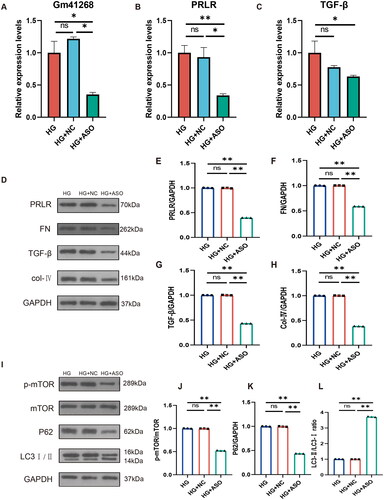
3.7. Knockdown of PRLR ameliorates high glucose-induced fibrosis and enhances autophagy in NRK-52E cells
We used immunofluorescence to observe the expression of PRLR in NRK-52E cells. The results showed that PRLR was highly expressed in NRK-52E cells in HG group compared with the NG group, and treatment with 500 μg/mL APS could reduce PRLR expression levels in NRK-52E cells cultured in HG (). As the role of PRLR in DN remains unclear, we used PRLR siRNA to knock down the expression of PRLR. NRK-52E cells were treated with NG combined with NC (NG + NC group), HG combined with NC (HG + NC group), HG combined with siPRLR (HG + siPRLR group), HG combined with APS at 500 μg/mL and NC (HG + APS + NC group) and HG combined with APS at 500 μg/mL and siPRLR (HG + APS + siPRLR group). Our results revealed that inhibition of PRLR can promote autophagy and ameliorate renal fibrosis in HG-treated NRK-52E cells (). Interestingly, compared with the HG group transfected with APS and NC, the expression of the p62 protein was significantly downregulated, the ratio of LC3II/LC3 was significantly upregulated, and the expression of FN and TGF-β also decreased significantly in the HG group transfected with APS and siPRLR (), which indicated that inhibition of PRLR could enhance the protective effect of APS on HG-induced NRK cells.
Figure 7. Knockdown of PRLR ameliorates high glucose-induced fibrosis and enhances autophagy in NRK-52E cells. (A, B) The expression of PRLR was evaluated by immunofluorescence (n = 3). (C–F) The expression of FN, TGF-β, P62, and LC3 was evaluated by western blotting (n = 3). NRK-52E cells were treated with 500 μg/mL APS. Data are presented as the mean ± SD. *p < .05; **p < .001.
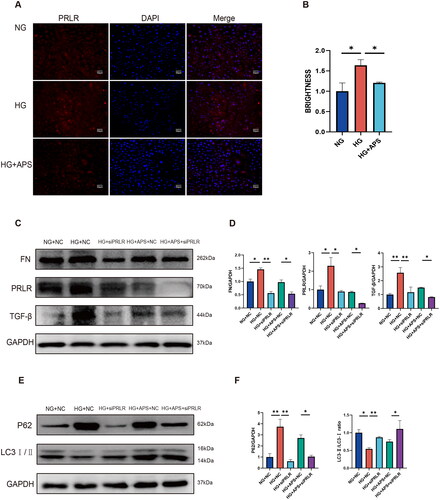
4. Discussion
DN is a chronic disease that poses a great burden on health and quality of life and can easily lead to end-stage renal disease [Citation29]. New innovative technologies and methods are required to further elucidate the molecular mechanisms involved in DN pathogenesis. For example, the regulation of RNA expression by cytoplasmic lncRNAs is an interesting research area because lncRNAs are implicated in the regulation of gene expression by affecting mRNA stability [Citation30]. Several lncRNAs have been shown to play essential roles in DN, such as 1700020I14Rik, which was reported to alleviate cell proliferation and fibrosis in DN [Citation31], and MALAT1, which was shown to mediate epithelial-to-mesenchymal transition in HK-2 cells [Citation32]. Therefore, we selected RNA-Seq, an innovative technique commonly used to study disease mechanisms [Citation33,Citation34], as a tool to identify differentially expressed lncRNAs. In addition, by analyzing RNA-seq data, we could predict the target genes of these RNAs and use this information as a starting point for future research. Here, we identified a novel lncRNA, Gm41268, which has not been previously reported and demonstrated that APS treatment could delay the progression of DN in vivo by downregulating the expression of Gm41268 and its target gene PRLR. We also inhibited Gm41268 expression by transfecting Gm41268-targeting ASOs into NRK-52E cells and observed that inhibition of Gm41268 enhanced autophagy and alleviated fibrosis in HG-treated cells, validating the functions of Gm41268 in DN in vitro.
Recently, emerging evidence has demonstrated the importance of lncRNAs in the regulation of gene transcription. lncRNAs can be divided into two categories: those that influence nearby genes in cis and those that exert their function in trans [Citation35]. Defining cis-regulatory lncRNAs mainly depends on the distance between lncRNAs and regulated mRNAs and whether the coding region of lncRNA contains cis-acting elements of certain mRNAs [Citation36]. We utilized bioinformatics techniques and predicted that Gm41268 may regulate PRLR via a cis-regulating action. PRLR belongs to the cytokine receptor superfamily and has been demonstrated to bind to at least three different ligands, namely prolactin, placental lactogen, and growth hormone, thereby complicating the investigation of its role [Citation37]. Several studies have investigated the function of PRLR in the regulation of glucose homeostasis, β-cell proliferation, and survival and found that STZ-treated diabetic Prlr–/– mice showed increased glucose levels and impaired glucose intolerance [Citation38,Citation39]. However, the role of PRLR in DN remains to be elucidated. A recent study revealed that serum prolactin and renal mRNA expression of PRLR were increased in streptozotocin-induced diabetic rats, indicating a potential role for PRLR in the development of DN [Citation40]. PRLR binds ligands to initiate several intracellular signaling pathways, including the STAT5, PI3K/AKT, and Ras–Raf–Mek–MAPK pathways [Citation41]. The PI3K/AKT pathway is associated with the regulation of autophagy [Citation42]. Thus, we hypothesized that Gm41268 affects autophagy and fibrosis by regulating its target gene PRLR. Our results showed that the mRNA and protein expression of PRLR was increased in the kidneys of DN mice and that APS can downregulate the expression of PRLR, which provides a new target for APS in the treatment of DN.
Autophagy is a highly conserved process in eukaryotic cells that maintains cell stability and strengthens cell tolerance to environmental changes [Citation43]. LC3, p62, and mTOR are commonly used as markers to monitor autophagy activation and flux [Citation44–46]. During autophagy, LC3-I is cleaved and converted to LC3-II, which translocates to the autophagosomal membrane, leading to the formation of autophagosomes [Citation47,Citation48]. P62, an important autophagy receptor, is negatively correlated with the number of autophagic lysosomes [Citation49]. P62 also plays a vital role in the regulation of intracellular signaling, such as the mTOR pathway [Citation50]. As a key molecule in the mTOR signaling pathway, mTOR functions as a hub for maintaining glucose homeostasis and regulating autophagy [Citation51]. Studies have demonstrated that inhibition of autophagy is involved in DN pathogenesis [Citation52,Citation53]. Additionally, recent studies have suggested that the activation of autophagy can help resist fibrosis and delay DN progression [Citation54,Citation55]. Autophagy regulates myofibroblast differentiation, tissue remodeling, and fibrogenesis [Citation56,Citation57]. Our previous study also demonstrated an interaction between autophagy and fibrosis [Citation58]. However, few studies have explored the effects of herbal medicines on fibrosis by regulating autophagy [Citation59]. However, the role of APS in autophagy in DN has not yet been studied. In this study, we demonstrated that APS enhanced hyperglycemia-induced inhibition of autophagy by downregulating the expression of p62 and p-mTOR, increasing the LC3 II/I ratio, improving renal fibrosis, and mitigating DN progression. These results highlight the potential of APS and provide a basis for its efficacy through the discovery of novel therapeutic mechanisms.
Although we confirmed the therapeutic properties of APS both in vivo and in vitro, our study had several limitations. First, we needed to verify whether Gm41268 plays a critical function in vivo, which could be performed by overexpressing and knocking down Gm41268 in mice in our future work. Second, we did not verify the role of lncRNA Gm41268 in the protection of HG-induced NRK-52E cells by APS. In the future study, we will conduct additional experiments to assess whether overexpression of lncRNA Gm41268 affect the protective effect of APS on NRK-52E cells cultured in HG and observe the changes in the expression level of PRLR after overexpression of lncRNA Gm41268 to better clarify the regulatory relationship between lncRNA Gm41268 and target gene PRLR. Third, we can further investigate the function of PRLR by overexpressing it in vitro, which could be helpful in elucidating the mechanism of APS in treating DN. Finally, KEGG results indicated that APS could regulate RNAs involved in other cellular processes, such as metabolism and endocytosis. Therefore, the therapeutic mechanisms of APS should be further investigated in other biological processes.
In conclusion, we used RNA-seq to discover the molecular mechanisms underlying APS action in DN. Using in vivo and in vitro models, we demonstrated that APS exerted a therapeutic effect by promoting autophagy and inhibiting renal fibrosis in DN by targeting the lncGm41268/PRLR pathway (). Our study provides a new perspective on the promising therapeutic potential of the active constituents of Chinese herbs in the treatment of DN.
Author contributions
Ya Xiao and Xiaoshan Zhao conceived the study. Zedong Chen performed experiments and drafted the manuscript. Liang et al. collected data and provided assistance with in vitro experiments. Zhenyu Bai, Ting Xie, and Jiaojiao Dai participated in data analysis. Ya Xiao revised the manuscript accordingly.
Ethical approval
Animal experiments were approved by the Animal Experiment Ethics Committee of Jinan University (approval number: 2019022501).
Supplemental Material
Download PDF (22.5 KB)Supplemental Material
Download PDF (91.1 KB)Disclosure statement
The authors declare no conflict of interest.
Data availability statement
The datasets used to support the findings of this study are available from the corresponding author upon request.
Additional information
Funding
References
- Umanath K, Lewis JB. Update on diabetic nephropathy: core curriculum 2018. Am J Kidney Dis. 2018;71(6):1–15. doi: 10.1053/j.ajkd.2017.10.026.
- DeFronzo RA, Reeves WB, Awad AS. Pathophysiology of diabetic kidney disease: impact of SGLT2 inhibitors. Nat Rev Nephrol. 2021;17(5):319–334. doi: 10.1038/s41581-021-00393-8.
- Sun H, Saeedi P, Karuranga S, et al. IDF diabetes atlas: global regional and country-level diabetes prevalence estimates for 2021 and projections for 2045. Diabetes Res Clin Pract. 2023;204:110945. doi: 10.1016/j.diabres.2021.109119.
- Gupta S, Dominguez M, Golestaneh L. Diabetic kidney disease: an update. Med Clin North Am. 2023;107(4):689–705. doi: 10.1016/j.mcna.2023.03.004.
- Hung PH, Hsu YC, Chen TH, et al. Recent advances in diabetic kidney diseases: from kidney injury to kidney fibrosis. Int J Mol Sci. 2021;22(21):11857. doi: 10.3390/ijms222111857.
- Donate-Correa J, Ferri CM, Sanchez-Quintana F, et al. Inflammatory cytokines in diabetic kidney disease: pathophysiologic and therapeutic implications. Front Med. 2020;7:628289. doi: 10.3389/fmed.2020.628289.
- Ricciardi CA, Gnudi L. Kidney disease in diabetes: from mechanisms to clinical presentation and treatment strategies. Metabolism. 2021;124:154890. doi: 10.1016/j.metabol.2021.154890.
- Tang C, Livingston MJ, Liu Z, et al. Autophagy in kidney homeostasis and disease. Nat Rev Nephrol. 2020;16(9):489–508. doi: 10.1038/s41581-020-0309-2.
- Tang G, Li S, Zhang C, et al. Clinical efficacies underlying mechanisms and molecular targets of Chinese medicines for diabetic nephropathy treatment and management. Acta Pharm Sin B. 2021;11(9):2749–2767. doi: 10.1016/j.apsb.2020.12.020.
- Hu Q, Chen Y, Deng X, et al. Diabetic nephropathy: focusing on pathological signals, clinical treatment, and dietary regulation. Biomed Pharmacother. 2023;159:114252. doi: 10.1016/j.biopha.2023.114252.
- Chinese Pharmacopoeia Commission. The 2020 edition of Pharmacopoeia of the People’s Republic of China. Beijing, China: Chemical Industry Press; 2020.
- Tian H, Lu J, He H, et al. The effect of astragalus as an adjuvant treatment in type 2 diabetes mellitus: a (preliminary) meta-analysis. J Ethnopharmacol. 2016;191:206–215. doi: 10.1016/j.jep.2016.05.062.
- Zhang HW, Lin ZX, Xu C, et al. Astragalus (a traditional Chinese medicine) for treating chronic kidney disease. Cochrane Database Syst Rev. 2014;22:CD008369.
- Li M, Wang W, Xue J, et al. Meta-analysis of the clinical value of astragalus membranaceus in diabetic nephropathy. J Ethnopharmacol. 2011;133(2):412–419. doi: 10.1016/j.jep.2010.10.012.
- Zhang L, Shergis JL, Yang L, et al. Astragalus membranaceus (Huang Qi) as adjunctive therapy for diabetic kidney disease: an updated systematic review and meta-analysis. J Ethnopharmacol. 2019;239:111921. doi: 10.1016/j.jep.2019.111921.
- Du Y, Wan H, Huang P, et al. A critical review of Astragalus polysaccharides: from therapeutic mechanisms to pharmaceutics. Biomed Pharmacother. 2022;147:112654. doi: 10.1016/j.biopha.2022.112654.
- Liu T, Zhang M, Niu H, et al. Astragalus polysaccharide from astragalus melittin ameliorates inflammation via suppressing the activation of TLR-4/NF-kappaB p65 signal pathway and protects mice from CVB3-induced virus myocarditis. Int J Biol Macromol. 2019;126:179–186. doi: 10.1016/j.ijbiomac.2018.12.207.
- Li C, Pan X, Ma M, et al. Astragalus polysacharin inhibits hepatocellular carcinoma-like phenotypes in a murine HCC model through repression of M2 polarization of tumour-associated macrophages. Pharm Biol. 2021;59(1):1533–1539. doi: 10.1080/13880209.2021.1991384.
- Meng X, Wei M, Wang D, et al. Astragalus polysaccharides protect renal function and affect the TGF-β/Smad signaling pathway in streptozotocin-induced diabetic rats. J Int Med Res. 2020;48(5):300060520903612. doi: 10.1177/0300060520903612.
- Zhang Y, Wu C, Cheng J. Merit of Astragalus polysaccharide in the improvement of early diabetic nephropathy with an effect on mRNA expressions of NF-κB and IκB in renal cortex of streptozotocin-induced diabetic rats. J Ethnopharmacol. 2007;114(3):387–392. doi: 10.1016/j.jep.2007.08.024.
- Qian X, Zhao J, Yeung PY, et al. Revealing lncRNA structures and interactions by sequencing-based approaches. Trends Biochem Sci. 2019;44(1):33–52. doi: 10.1016/j.tibs.2018.09.012.
- Guo J, Liu Z, Gong R. Long noncoding RNA: an emerging player in diabetes and diabetic kidney disease. Clin Sci. 2019;133(12):1321–1339. doi: 10.1042/CS20190372.
- Saghafi T, Taheri RA, Parkkila S, et al. Phytochemicals as modulators of long non-coding RNAs and inhibitors of cancer-related carbonic anhydrases. Int J Mol Sci. 2019;20(12):2939. doi: 10.3390/ijms20122939.
- Hong F, Gu W, Jiang J, et al. Anticancer activity of polyphyllin I in nasopharyngeal carcinoma by modulation of lncRNA ROR and P53 signalling. J Drug Target. 2019;27(7):806–811. doi: 10.1080/1061186X.2018.1561887.
- Hu T, Gao Y. β-Elemene suppresses tumor growth of diffuse large B-cell lymphoma through regulating lncRNA HULC-mediated apoptotic pathway. Biosci Rep. 2020;40(2):BSR20190804.
- Hrdlickova R, Toloue M, Tian B. RNA-Seq methods for transcriptome analysis. WIREs RNA. 2017;8(1):1002. doi: 10.1002/wrna.1364.
- Kavarthapu R, Anbazhagan R, Dufau ML. Crosstalk between PRLR and EGFR/HER2 signaling pathways in breast cancer. Cancers. 2021;13(18):4685. doi: 10.3390/cancers13184685.
- Yang F, Qu Q, Zhao C, et al. Paecilomyces cicadae-fermented Radix astragali activates podocyte autophagy by attenuating PI3K/AKT/mTOR pathways to protect against diabetic nephropathy in mice. Biomed Pharmacother. 2020;129:110479. doi: 10.1016/j.biopha.2020.110479.
- Kato M, Natarajan R. Epigenetics and epigenomics in diabetic kidney disease and metabolic memory. Nat Rev Nephrol. 2019;15(6):327–345. doi: 10.1038/s41581-019-0135-6.
- Bridges MC, Daulagala AC, Kourtidis A. LNCcation: lncRNA localization and function. J Cell Biol. 2021;220:e202009045.
- Li A, Peng R, Sun Y, et al. LincRNA 1700020I14Rik alleviates cell proliferation and fibrosis in diabetic nephropathy via miR-34a-5p/Sirt1/HIF-1α signaling. Cell Death Dis. 2018;9(5):461. doi: 10.1038/s41419-018-0527-8.
- Zhang JUN, Jiang T, Liang X, et al. lncRNA MALAT1 mediated high glucose-induced HK-2 cell epithelial-to-mesenchymal transition and injury. J Physiol Biochem. 2019;75(4):443–452. doi: 10.1007/s13105-019-00688-2 31388927.
- Zappia L, Theis FJ. Over 1000 tools reveal trends in the single-cell RNA-Seq analysis landscape. Genome Biol. 2021;22(1):301. doi: 10.1186/s13059-021-02519-4.
- Rao DA, Arazi A, Wofsy D, et al. Design and application of single-cell RNA sequencing to study kidney immune cells in lupus nephritis. Nat Rev Nephrol. 2020;16(4):238–250. doi: 10.1038/s41581-019-0232-6.
- Font-Cunill B, Arnes L, Ferrer J, et al. Long non-coding RNAs as local regulators of pancreatic islet transcription factor genes. Front Genet. 2018;9:524. doi: 10.3389/fgene.2018.00524.
- Kopp F, Mendell JT. Functional classification and experimental dissection of long noncoding RNAs. Cell. 2018;172(3):393–407. doi: 10.1016/j.cell.2018.01.011.
- Bernard V, Young J, Binart N. Prolactin – a pleiotropic factor in health and disease. Nat Rev Endocrinol. 2019;15(6):356–365. doi: 10.1038/s41574-019-0194-6.
- Ramirez-Hernandez G, Adan-Castro E, Diaz-Lezama N, et al. Global deletion of the prolactin receptor aggravates streptozotocin-induced diabetes in mice. Front Endocrinol. 2021;12:619696. doi: 10.3389/fendo.2021.619696.
- Khant AZ, Kokay IC, Grattan DR, et al. Prolactin-Induced adaptation in glucose homeostasis in mouse pregnancy is mediated by the pancreas and not in the forebrain. Front Endocrinol. 2021;12:765976. doi: 10.3389/fendo.2021.765976.
- Al-Trad B. Prolactin receptor mRNA expression in experimental diabetic nephropathy: relationship with urinary albumin excretion. Neuro Endocrinol Lett. 2015;36:552–556.
- Nie H, Huang PQ, Jiang SH, et al. The short isoform of PRLR suppresses the pentose phosphate pathway and nucleotide synthesis through the NEK9-Hippo axis in pancreatic cancer. Theranostics. 2021;11(8):3898–3915. doi: 10.7150/thno.51712.
- Duggan MR, Weaver M, Khalili K. PAM (PIK3/AKT/mTOR) signaling in glia: potential contributions to brain tumors in aging. Aging. 2021;13(1):1510–1527. doi: 10.18632/aging.202459.
- Dong JY, Yin HL, Hao H, et al. Research progress on autophagy regulation by active ingredients of traditional Chinese medicine in the treatment of acute lung injury. J Inflamm Res. 2023;16:1671–1691. doi: 10.2147/JIR.S398203.
- Liu GY, Sabatini DM. mTOR at the nexus of nutrition growth ageing and disease. Nat Rev Mol Cell Biol. 2020;21(4):183–203. doi: 10.1038/s41580-019-0199-y.
- Li X, He S, Ma B. Autophagy and autophagy-related proteins in cancer. Mol Cancer. 2020;19(1):12. doi: 10.1186/s12943-020-1138-4.
- Deng Z, Lim J, Wang Q, et al. ALS-FTLD-linked mutations of SQSTM1/p62 disrupt selective autophagy and NFE2L2/NRF2 anti-oxidative stress pathway. Autophagy. 2020;16(5):917–931. doi: 10.1080/15548627.2019.1644076.
- Weidberg H, Shvets E, Elazar Z. Biogenesis and cargo selectivity of autophagosomes. Annu Rev Biochem. 2011;80(1):125–156. doi: 10.1146/annurev-biochem-052709-094552.
- Schaaf MB, Keulers TG, Vooijs MA, et al. LC3/GABARAP family proteins: autophagy-(un)related functions. FASEB J. 2016;30(12):3961–3978. doi: 10.1096/fj.201600698R.
- Mizushima N, Komatsu M. Autophagy: renovation of cells and tissues. Cell. 2011;147(4):728–741. doi: 10.1016/j.cell.2011.10.026.
- Liu WJ, Ye L, Huang WF, et al. p62 links the autophagy pathway and the ubiquitin–proteasome system upon ubiquitinated protein degradation. Cell Mol Biol Lett. 2016;21(1):29. doi: 10.1186/s11658-016-0031-z.
- Saxton RA, Sabatini DM. mTOR signaling in growth metabolism and disease. Cell. 2017;168(6):960–976. doi: 10.1016/j.cell.2017.02.004.
- Yang C, Chen XC, Li ZH, et al. SMAD3 promotes autophagy dysregulation by triggering lysosome depletion in tubular epithelial cells in diabetic nephropathy. Autophagy. 2021;17(9):2325–2344. doi: 10.1080/15548627.2020.1824694.
- Su P, Liu D, Zhou S, et al. Down-regulation of risa improves podocyte injury by enhancing autophagy in diabetic nephropathy. Mil Med Res. 2022;9:23.
- Tian F, Wang Z, He J, et al. 4-Octyl itaconate protects against renal fibrosis via inhibiting TGF-β/Smad pathway autophagy and reducing generation of reactive oxygen species. Eur J Pharmacol. 2020;15:873.
- Nam SA, Kim WY, Kim JW, et al. Autophagy in FOXD1 stroma-derived cells regulates renal fibrosis through TGF-β and NLRP3 inflammasome pathway. Biochem Biophys Res Commun. 2019;508(3):965–972. doi: 10.1016/j.bbrc.2018.11.090.
- Migneault F, Hebert MJ. Autophagy tissue repair and fibrosis: a delicate balance. Matrix Biol. 2021;100–101:182–196. doi: 10.1016/j.matbio.2021.01.003.
- Liang S, Wu YS, Li DY, et al. Autophagy and renal fibrosis. Aging Dis. 2022;13(3):712–731. doi: 10.14336/AD.2021.1027.
- Liang Q, Liu T, Guo T, et al. ATF4 promotes renal tubulointerstitial fibrosis by suppressing autophagy in diabetic nephropathy. Life Sci. 2021;264:118686. doi: 10.1016/j.lfs.2020.118686.
- Liang Q, Bai Z, Xie T, et al. Deciphering the pharmacological mechanisms of Qidan Dihuang decoction in ameliorating renal fibrosis in diabetic nephropathy through experimental validation in vitro and in vivo. Evid Based Complement Alternat Med. 2022;2022:4137578. doi: 10.1155/2022/4137578.