Abstract
This study aimed to explore the mechanism of Xiaoyu Xiezhuo decoction (XXD) on ischemia–reperfusion-induced acute kidney injury (IRI-AKI) using network pharmacology methods and gut microbiota analysis. A total of 1778 AKI-related targets were obtained, including 140 targets possibly regulated by AKI in XXD, indicating that the core targets were mainly enriched in inflammatory-related pathways, such as the IL-17 signaling pathway and TNF signaling pathway. The unilateral IRI-AKI animal model was established and randomly divided into four groups: the sham group, the AKI group, the sham + XXD group, and the AKI + XXD group. Compared with the rats in the AKI group, XXD improved not only renal function, urinary enzymes, and biomarkers of renal damage such as Kim-1, cystatin C, and serum inflammatory factors such as IL-17, TNF-α, IL-6, and IL 1-β, but also intestinal metabolites including lipopolysaccharides, d-lactic acid, indoxyl sulfate, p-cresyl sulfate, and short-chain fatty acids. XXD ameliorated renal and colonic pathological injury as well as inflammation and chemokine gene abundance, such as IL-17, TNF-α, IL-6, IL-1β, ICAM-1, and MCP-1, in AKI rats via the TLR4/NF-κB/NLRP3 pathway, reducing the AKI score, renal pathological damage, and improving the intestinal mucosa’s inflammatory infiltration. It also repaired markers of the mucosal barrier, including claudin-1, occludin, and ZO-1. Compared with the rats in the AKI group, the α diversity was significantly increased, and the Chao1 index was significantly enhanced after XXD treatment in both the sham group and the AKI group. The treatment group significantly reversed this change in microbiota.
Introduction
Acute kidney injury (AKI) is characterized by a rapid reduction in glomerular filtration rate and/or urine output, as evidenced by elevations in serum creatinine (Scr) or reductions in urine volume [Citation1]. Incidence reports indicate that AKI affects approximately 5–7% of hospitalized patients, according to various single-center studies [Citation2,Citation3]. It stands as the most prevalent acute kidney condition, closely associated with critical complications and an increase in mortality rates [Citation4,Citation5]. Ischemia has been identified as a primary contributor to AKI development [Citation6]. Ischemia–reperfusion injury (IRI), resulting from blood reperfusion, often leads to tissue damage and is a common precipitant of AKI progression [Citation6,Citation7].
Recent research underscores the gut’s pivotal role in mediating complications related to kidney disease [Citation8]. Kidney diseases can disrupt the intestinal barrier, altering the composition and metabolic function of the gut microbiome. This disruption results in the generation of bioactive metabolites and toxins [Citation9]. In healthy individuals, the intestinal barrier effectively prevents the translocation of intestinal bacteria and toxins across the mucosa into the systemic circulation or other tissues. The fermentation of dietary fiber by gut microbiota results in the production of short-chain fatty acids (SCFAs), including acetate, propionate, and butyrate. Colonic bacteria ferment dietary proteins, resulting in the production of uremic toxins such as indoxyl sulfate (IS) and p-cresyl sulfate (PCS) [Citation10]. Tight junction (TJ) proteins such as occludin, ZO-1, and claudin-2 play crucial roles in regulating intestinal epithelial proliferation and survival [Citation11,Citation12]. Approximately a decade ago, gut dysbiosis was first reported in patients with kidney failure [Citation13]. While initial research focused on changes in gut microbial composition and abundance, more recent studies have explored functional capacity [Citation14,Citation15], with quantitative microbial profiling being introduced to complement relative profiling techniques [Citation16].
The development of AKI involves mechanisms related to the immune system, biochemistry, and hemodynamics. Research has commenced to unravel the underlying processes through which imbalances in the microbiome impact AKI. Animal experiments demonstrate the significant influence of gut microbiota on the advancement and consequences of AKI, highlighting the potential use of prebiotics, probiotics, or a combination thereof in treatment. Crucially, gut dysbiosis alters SCFA composition, antagonizing lipopolysaccharides (LPSs) and mitigating intestinal inflammation. Toxic compounds such as trimethylamine N-oxide (TMA/TMAO), in conjunction with uremic toxins and other toxins originating from the gastrointestinal tract, contribute to the development of AKI caused by nephrotoxicity [Citation10].
Evidence suggests that intestinal microbiota can modulate AKI. One proposed mechanism is the reno-protective action of SCFAs against IRI. SCFAs, known for their anti-inflammatory properties and produced by gut microbiota, were found to ameliorate renal dysfunction and reduce inflammation in models [Citation17]. Additionally, gut microbiota exerts broader influences, particularly in immune-mediated kidney diseases, through immunomodulatory effects, notably impacting T-cell subset polarization and natural killer cell functions [Citation18].
Considerable attention has been given to the potential clinical advantages of traditional Chinese medicine (TCM) in the treatment of AKI [Citation19]. For example, the ethanol extraction of Illicium henryi root bark has demonstrated significant dose-dependent protective effects in mice with AKI by suppressing inflammatory reactions and reducing oxidative stress [Citation20]. One of the nationally famous TCM doctors, Li Xueming, who has been a doctor for sixty years, is known for treating kidney disease and internal medical diseases. Xiaoyu Xiezhuo decoction (XXD), comprising Astragalus membranaceus (15 g), processed Radix cyathulae (6 g), Peach Kernel (6 g), Lumbricus (6 g), prepared Rhubarb (5 g), and Plantain (10 g), is his experienced formula, used in the long-range clinical treatment of AKI of qi deficiency with blood stasis and turbidity syndrome, and has been incorporated as a nosocomial preparation. XXD uses rhubarb as the principal herb, together with Peach Kernel and processed Radix cyathulae, to promote blood circulation, remove blood stasis, and clear away turbid toxins, inspired by the ‘Buyang Huanwu’ Decoction recorded in ‘Yilin Gaicuo’ by Wang Qingren, a famous medical practitioner of the Qing Dynasty. Recent studies have indicated the efficacy of XXD as a treatment option for patients with AKI, and animal experiments revealed its capability to reduce pathological harm and diminish the levels of α-smooth muscle actin (α-SMA), TGF-β1, and collagen-1 proteins in renal tissues of mice with unilateral ureteral occlusion (UUO). Additionally, it can also enhance Smad7 levels in these tissues [Citation21,Citation22]. A recent study suggested that this decoction exhibits protective properties for renal function in elderly mice by suppressing the TGF-β1/Smad3 and HIF1 signaling pathways [Citation23].
In conclusion, AKI involves a complex interplay of immunological, biochemical, and hemodynamic mechanisms. Emerging experimental data underscore the significant influence of intestinal microbiota on AKI outcomes [Citation24]. To this end, we examined the active components of XXD and its potential targets for treating AKI. Network pharmacology analysis, functional annotation, and pathway enrichment analysis were conducted in the ischemia–reperfusion-induced acute kidney injury (IRI-AKI) model to further validate the molecular mechanism of XXD against AKI. Furthermore, the impact of XXD on the gut microbiota of AKI rats was assessed through 16S rRNA sequencing analysis.
Materials and methods
Network pharmacological study
Acquisition of targets corresponding to prescription
Using the TCMSP (Traditional Chinese Medicine Systems Pharmacology Database and Analysis Platform, http://tcmspw.com/), keywords such as ‘Huang Qi’ (Hedysarum Multijugum Maxim.), ‘Chuan Niu Xi’ (Cyathulae Radix), ‘Tao Ren’ (Persicae Semen), ‘Da Huang’ (Radix Rhei Et Rhizome), and ‘Che Qian Cao’ (Plantaginis Herba) were used to search for eligible chemical components and their corresponding targets (filtering criteria: OB ≥ 30% and DL ≥ 0.15) [Citation25]. Since TCMSP did not include data related to ‘Di Long’ (Pheretima vulgaris Clen), the relevant literature on its chemical constituents was searched in CNKI (https://www.cnki.net/) to collect the necessary information. The Canonical SMILES of the chemical constituents of ‘Di Long’ (Pheretima vulgaris Clen) were downloaded from the PubChem website (https://pubchem.ncbi.nlm.nih.gov/) and imported into the SwissADME website (http://www.swissadme.ch/). Chemical constituents with ‘High’ GI absorption and ‘Yes’ Lipinski results were selected and imported into the SwissADME website (http://www.swissadme.ch), and the chemical constituents with ‘High’ GI absorption and ‘Yes’ Lipinski results were selected and imported into SwissTargetPrediction website (http://swisstargetprediction.ch). The target information of the above chemical components was collected on the SwissTargetPrediction website with a screening condition of probability >0. All targets were calibrated against the UniProt database (https://www.uniprot.org), selecting ‘Homo sapiens’ in UniProt ID format. The obtained targets were combined and de-weighted to construct the target set of the formula.
Acquisition of disease-corresponding targets
We searched the GeneCards database (https://www.genecards.org/), PharmGKB database (https://www.pharmgkb.org/), DisGeNET database (https://www.disgenet.org/), and TTD database (https://db.idrblab.net/ttd/) for genes related to AKI using the keywords ‘Acute kidney injury, AKI.’ We searched the databases for genes related to AKI including GeneCards database (https://www.genecards.org/), PharmGKB database (https://www.pharmgkb.org), DisGeNET database (https://www.disgenet.org/), and TTD database (http://db.idrblab.net/ttd/). The GeneCards database underwent screening with a relevance score exceeding 10, and the results from the four databases were combined to create the AKI-related target set, eliminating any duplicate entries. The intersection Venn diagram of XXD and AKI-related targets was plotted using the online platform VENNY 2.1 (https://bioinfogp.cnb.csic.es/tools/venny/), followed by extracting the intersection target data.
Protein–protein interaction (PPI) for predicting the core target of XXD in treating AKI
The intersection targets were imported into the STRING database (https://string-db.org/), selecting ‘Homo sapiens’ with an interaction score >0.700 and concealing the free nodes to establish a network of protein interactions for the intervention of AKI by XXD. The network was opened in Cytoscape software [Citation26], and the medians of the topological parameters of the nodes in the network (Degree, DC, Betweenness, BC, Closeness, CC) were calculated using the CytoNCA plug-in, repeated twice to obtain the top five target sites for the intervention of AKI by XXD. The ranking was assigned to the core targets, specifically referring to the top five targets.
Construction of the XXD formula-active component-target regulatory network
XXD formula, its matching active components, and intersecting targets were imported into Cytoscape software to construct the ‘XXD-Active component-Target’ network.
Path prediction of XXD in treating AKI
The core targets obtained were subjected to the Gene Ontology (GO) function and Kyoto Encyclopedia of Genes and Genomes (KEGG) pathway enrichment analyses using R software. Bar graphs illustrating the top 20 KEGG pathways based on p value were generated with a filtering condition of p < .05. Biological process (BP), cellular component (CC), molecular function (MF), and KEGG pathways with the top 20 p values were selected and plotted as bar charts. The obtained KEGG pathway data were imported into Cytoscape software to construct the ‘KEGG pathways-core targets’ network [Citation27].
Experimental validation studies
Experimental animals
The Ethics Committee of Zhejiang University of Traditional Chinese Medicine (IACUC-202111-32) approved the in vivo study protocol, adhering to the Guide for the Care and Use of Laboratory Animals published by the NIH (eighth edition, updated 2011). A total of 60 male Sprague Dawley rats, aged 6 weeks, and weighing 180–200 g, were obtained from Sberford (Beijing) Biotechnology Co., Ltd. (Beijing, China) (license number SYXK (Zhe) 2018-0012) as specific-pathogen-free-grade animals. The rats were housed in the animal room of Zhejiang University of Traditional Chinese Medicine at a temperature of 23 ± 2 °C, humidity of (65% ± 5%), with a 12-h light cycle, and had free access to clean drinking water and food. The rats were euthanized by CO2 inhalation at 30% vol/min. Death was confirmed by the absence of breathing, stiffness, and dilated pupils, after which the CO2 was terminated, and the rats were observed for 2 min to verify death. After euthanasia, the bodies were taken out and stored in a designated freezer. Urine, kidneys, blood samples, colon tissue, and its contents were obtained for subsequent analysis.
Experimental drugs
Based on previous experience [Citation23], XXD consists of 15 g ‘Huang Qi’ (Hedysarum Multijugum Maxim.; Inner Mongolia, no. 20231016), 6 g ‘Chuan Niu Xi’ (Cyathulae Radix; Sichuan, no. 20230901), 6 g ‘Tao Ren’ (Persicae Semen; Sichuan, no. 231122), 5 g ‘Da Huang’ (Radix Rhei Et Rhizome; Hubei, no. 20231001), 10 g ‘Che Qian Cao’ (Plantaginis Herba; Jiangxi, no. 231218), and 6 g ‘Di Long’ (Pheretima vulgaris Clen; Guangxi, no. 240123). The XXD extract was obtained from the dispensary of the First Affiliated Hospital of Zhejiang Chinese Medical University (Zhejiang, China). Each sample stock contained a total of 48 g of crude drug preparation. A dose of 0.800 g/kg crude drug is recommended, assuming an average human body weight of 60 kg. The conversion factor of body surface area between humans and rats is 6.3. Therefore, the dose administered to the rats was calculated to be 5.04 g/kg crude drug preparation, equivalent to that administered to the medium-dose group of animals. The XXD was soaked in 10 volumes of water for 1 h, then decocted for 1 h with 100 °C distilled water, followed by filtration using gauze. This decoction step was repeated twice. The resulting fluid was mixed three times and concentrated to solutions equivalent to 0.504 g/mL clinical dose groups. After preparation, the drug was stored in a refrigerator at 4 °C for future use and shaken well before use.
Animal grouping and modeling
After one week of adaptive feeding, the rats were randomly divided into two groups: the sham group (n = 30) and the AKI group (n = 30). The unilateral renal IRI (U-IRI) method was used to prepare the AKI model [Citation28]. The rats were anesthetized, and their limbs were fixed with a cotton rope. The abdominal skin was disinfected with iodophor and then cut open along the midline. The renal capsule was bluntly separated during the operation, and microscopic forceps were used to separate the renal pedicle. A noninvasive arterial clamp was used to clamp the right renal peduncles. The right kidney changed from red to purple-black, indicating successful clamping. After 45–60 min of ischemia, the arterial clamp was released, and the renal blood flow was observed for 3–5 min until it recovered. The right kidney changed from purple-black to red, indicating that the pathological and physiological process of renal IRI was achieved. To ensure 24-h perfusion, the rats were injected with 1 mL of warm sterile saline into the abdominal cavity before layered suture closure. After surgery, to avoid excessive loss of body fluids, the rats were supplemented with water and feed. The sham surgery group underwent all the surgical procedures except for inducing the IRI-AKI process.
Drug-dosing interventions
Rats with appropriate AKI scores [Citation29] or serum blood creatinine levels more than twice as high 24 h after the operation were considered successfully modeled and selected for further study. The animals were randomly allocated into four groups, each consisting of 15 animals:
The sham group (sham group).
Sham group with traditional Chinese medicine group (sham + XXD group).
IRI-AKI group (AKI group).
IRI-AKI group with traditional Chinese medicine group (AKI + XXD group).
On the same day after modeling, drugs were administered to all four groups. 0.9% saline was given to both the sham and AKI groups, while 5.04 g/kg XXD was given to both the sham + XXD and AKI + XXD groups for a week. On the 1st and 7th days after drug intervention, seven animals in each group were euthanized and examined. Before sampling, metabolic cages were used to collect 24-h urine, and then serum, kidney, feces, and colon tissues were collected and preserved by placing them in a refrigerator set at a temperature of −80 °C.
Detection of urinary enzyme and renal function
On the first and seventh days following the intervention, metabolic cages were used to collect urine from the rats over a 24-h period. The urine was then tested to measure urinary N-acetyl-β-d-glucosaminidase (NAG) enzyme levels. Additionally, all rats from each group were sacrificed under 2% sodium pentobarbital anesthesia conditions. Serum creatinine, blood urea nitrogen (BUN), and cystatin C (Cys-c) levels were measured using a serum multiple automatic biochemical analyzer, with blood collected via the abdominal aortic approach. The blood samples underwent centrifugation at 3000 revolutions per minute for 15 min, after which the serum was gathered and preserved at −80 °C.
Histopathological analysis
Kidney and colon tissues were fixed in a solution containing 4% paraformaldehyde for 24 h, dehydrated, and made transparent. The resulting tissue sections, approximately 4 μm thick, were subjected to staining using the hematoxylin–eosin (HE) method. The sections were then examined under a microscope to observe any pathological changes in the kidney and colon tissues post-HE staining. The AKI score criteria are based on the percentage of damage area in the visual field. Ten visual fields are selected, and each is viewed 20 times. The score is calculated as follows: no damage (0 points), 0–25% (1 point), 25–50% (2 points), 50–75% (3 points), and greater than 75% (4 points). The infiltration of inflammatory cells in the colon was scored as follows [Citation30]: standard (0 points): intermittent presence of inflammatory cells within the lamina propria; mild (1 point): increased inflammatory cells within the lamina propria; moderate (2 points): fused inflammatory cells infiltrating the submucosal layer; severe (3 points): transmural extension of inflammatory cells. Tissue damage in the colon was scored as follows [Citation30]: normal (0 points): no damage seen in the mucosa; mild (1 point): punctate mucosal erosion; moderate (2 points): surface mucosal or localized ulcerative erosion; severe (3 points): significant impairment of the mucosal lining, with involvement of underlying layers within the intestinal wall.
Immunohistochemical analysis
Immunohistochemical staining was performed on prepared paraffin sections of colon and kidney tissues after seven days of drug intervention using the Envision method. To prepare the sections, they underwent regular dewaxing and hydration and were immersed in a citrate buffer with pH 6.0 for 5 min to repair the antigen. The endogenous peroxidase was inactivated using 3% H2O2, and the sections were titrated with I-anti working solution, including IL-1β (1:300, AF5103), NF-κB P65 (1:300, AF5006), NLRP3 (1:300, DF7438), TLR4 (1:300, AF5145), occludin (1:300, DF7504), ZO-1 (1:300, AF5145), claudin (1:300, AF0127), all from Affinity (San Francisco, CA); and KIM-1 (1:400, 30948-1-AP, Proteintech, Wuhan, China). The solution was left to incubate at 4 °C overnight, followed by an additional incubation period of 50 min at room temperature with horseradish enzyme-labeled anti-polymerase II. The sections were washed with phosphate-buffered saline (PBS) after each step. DAB was employed for color development, hematoxylin was used for restaining, and neutral resin was used to seal the film. Finally, image analysis was performed using ImageJ software (Bethesda, MD).
Detection of intestinal metabolites and inflammatory factors in serum by ELISA
Metabolic indicators of intestinal origin, such as LPSs, d-lactate (d-LA), indole phenol sulfate (IS), p-cresol sulfate (PCS), SCFAs, and inflammatory factors such as IL-1 β, IL-6, IL-17A, and TNF-α within each group, were detected using ELISA. ELISA kits (TSZ, Boston, MA, and Wuhan Enzyme Free Biotechnology, Wuhan, China) were measured with a plate reader (Thermo, Waltham, MA) using an experimental reagent kit and quantified by an enzyme-linked double antibody sandwich technique with a detection limit of 1.25 pg/mL to 80 pg/mL, a sensitivity of 0.31 pg/mL, a testing time of 4 h, a sample volume of 100 µL, and a detection wavelength of 450 nm.
Reverse transcription-quantitative PCR (RT-qPCR)
TRIzol solution (TRNzol Universal, TIANGEN, Beijing, China) was used to extract the total RNA after kidney and colonic tissues were collected from all groups of animals. Reverse transcription was performed using the All-in-One™ First-Strand cDNA Synthesis Kit (cat. no. AORT-0050; GeneCopoeia, Inc., Rockville, MD) following the manufacturer’s guidelines. The 2−ΔΔCt method was used for mRNA expression quantification after qPCR in the ABI7500 system (Thermo Fisher Scientific, Inc., Waltham, MA). The qPCR experiment was conducted using Platinum® SYBR® Green Realtime PCR Master Mix (cat. no. QPK-201; Toyobo Life Science, New York, NY). GAPDH was used as an internal reference control. Primer sequences can be found in .
Table 1. Primers for reverse transcription-quantitative polymerase chain reaction analysis.
Western blot analysis
Total kidney tissue proteins were isolated using RIPA lysis buffer enhanced with protease and phosphatase inhibitors (04693132001 and 4906837001, Roche, Basel, Switzerland). These proteins were then electrophoresed on SDS-PAGE gels (LABLAED, P41215, P00815) ranging from 7.5% to 15% and subsequently blotted onto PVDF membranes (IPVH00010, Millipore, Billerica, MA). After blocking with 5% BSA, the membranes were left to incubate overnight at 4 °C with primary antibodies at a dilution of 1:1000, specifically targeting NF-κB (8242, CST, Boston, MA) and phosphorylated NF-κB (3033, CST, Boston, MA). The membranes were then washed and incubated with secondary antibodies at a dilution of 1:2000, including HRP-conjugated Goat anti-Rabbit IgG (A0208, Beyotime, Shanghai, China), for an hour at ambient temperature. Chemiluminescent detection of protein bands was carried out using a substrate (WBKLS0500, Millipore, Billerica, MA) in a detection system (Bio-Rad, Hercules, CA). Band intensities were quantified with ImageJ software (National Institutes of Health, Bethesda, MD, https://imagej.net/software/).
Fecal sample processing
Fresh fecal samples from the rats were collected at approximately 10 a.m., one day, and seven days after the start of the experimental drug intervention using the tail-pull method. The samples were collected in sterile tubes, freeze-dried, and rapidly frozen using liquid nitrogen for 5 min, followed by storage at −80 °C.
Gut microbiota detection
16S rDNA sequencing of gut microbiota was performed using the Illumina MiSeq/NovaSeq platform by Tianjin Genink Biotechnology Co., Ltd. (Tianjin, China). Samples were subjected to genomic DNA extraction, followed by quantification using Nanodrop (Seattle, WA). The quality of DNA extraction was evaluated using agarose gel electrophoresis with a concentration of 1.2%. PCR amplification was conducted utilizing Barcode-specific sequences for the 16S V3-V4 region. The purification process involved the addition of magnetic beads (Vazyme VAHTSTM DNA Clean Beads, Nanjing, China) in a volume that was 0.8 times the PCR product’s volume, which amounted to 25 μL. Following that, the Illumina TruSeq Nano DNA LT Library Prep Kit (San Diego, CA) was employed to perform gel electrophoresis. The target bands were recovered, and double-end sequencing was performed with the NovaSeq sequencer to evaluate bacterial diversity and abundance.
Bioinformatics analysis
After preliminary screening of raw data based on sequence quality, libraries and samples were categorized based on index and Barcode data, and any barcode sequences were eliminated [Citation31]. Qiime dada2 denoise-paired was used to call DADA2 for quality assurance, noise reduction, and removal of chimeric sequences. Operational taxonomic units (OTU clustering) were performed based on 97% high similarity. The analysis [Citation32] included identifying the precise makeup of every sample across various taxonomic hierarchies, the distribution of ASV/OTU across various samples, evaluating the level of alpha diversity in every individual sample, checking sequencing depth appropriateness through rarefaction curves, and examining beta diversity differences and significance with principal coordinate analysis (PCoA). At the taxonomic level, variations in species abundance composition between different samples (groups) were measured. R language programs were used to create species distribution bar charts at different taxonomic levels to identify indicator species. The community was described based on OTU and their relationships, and the functionality of specific microbial groups was determined by comparing sequences with functional databases such as KEGG. Linear discriminant analysis (LEfSe) and microbial recursive partitioning (RPart) were used to analyze their effect categories [Citation33].
Statistical methods
The statistical analysis of the experiment’s data was conducted using SPSS (version 26.0; IBM Corp., Armonk, NY) and GraphPad Prism (version 8.0; Dotmatics, Boston, MA). Data normality was analyzed using the Shapiro–Wilk test and was expressed as the mean ± SD. If the data conformed to normality and Chi-square, a t-test was used to compare two groups, and ANOVA followed by Tukey’s and LSD tests was used to compare multiple groups. A difference was considered significant when the p value was less than .05. Alpha diversity index analysis was performed using QIIME2 dada2 software; beta diversity analysis and Venn diagrams were performed using R language scripts.
Results
XXD ameliorates renal and colonic pathological injury in AKI rats
In our study, the kidney and colonic pathological changes among different groups were observed on the first and seventh days. The sham and sham + XXD groups showed no inflammatory cell infiltration or fibrosis, and the AKI score was not significantly different from the other groups. The renal tubules were well-arranged, and the tubular epithelial cells were regularly arranged with a typical brush border. However, on the first day of AKI, there was a detachment of the brush border, necrotic and exfoliated tubular epithelial cells, and a bare basement membrane visible. There was also a tubular protein pattern and a minor presence of inflammatory cell infiltration. On the seventh day of AKI, there was a large amount of bare basement membrane, pronounced compensatory dilatation of the tubular lumen of the renal tubules, and inflammatory cell infiltration and fibrosis in the interstitium (). The AKI score was statistically significant for AKI-1d and AKI-7d vs. the sham group (**p < .01). After XXD treatment, the above pathology improved, and the AKI score showed a statistically significant improvement compared to the AKI group at corresponding time points (##p < .01, ). Immunohistochemical detection of the renal tubular injury marker KIM-1 results showed that there was a statistically significant difference between the AKI and sham groups (**p < .01), and the degree of KIM-1 was reduced after one day of XXD intervention compared to the AKI group at the same time point (##p < .01). However, there was no significant difference between the AKI-7d group and the AKI + AAD-7d group (nsp > .05). In the case of colonic pathological changes, the sham group and sham + XXD group showed no inflammatory cell infiltration from day 1 to day 7. However, on the first day of AKI, the intestinal epithelial tissue displayed structural deficits, epithelial cell damage, and loss of cup cells. Inflammatory cell infiltration increased with time, and by day 7, the intestinal mucosal barrier was compromised, and the severity of inflammatory cell infiltration was observed (). After XXD treatment, the condition of the colonic mucosal barrier and the extent of infiltration by inflammatory cells showed significant improvement ().
Figure 1. (A) Renal histopathology (HE staining ×200); (B) AKI score (compared to the sham group, **p < .01, nsp > .05; compared to the AKI group, ##p < .01, nsp > .05); (C) kidney tissue immunohistochemistry for KIM-1 (IHC staining ×200); (D) expression of KIM-1 of renal tissue (compared to the sham group, **p < .01, nsp > .05; compared to the AKI group, ##p < .01, nsp > .05); (E) colonic histopathology (bar = 250 μm); (F) colonic barrier tissue damage (%); (G) colonic barrier inflammatory cell infiltration (%) (Note. In the renal pathology pictures, △ indicates renal tubules were closely spaced back to back with normal brush border; ○ indicates tubular epithelial cells were regularly arranged; the black arrow indicates renal tubular brush border detachment with necrosis and exfoliation of tubular epithelial cells; the purple arrow indicates significant compensatory dilatation of renal tubular lumen; the yellow arrow indicates protein tubulin; the blue arrow indicates small amount of brush border detachment, bare basement membrane; the green arrow indicates small amount of interstitial inflammatory cell infiltration; the blue arrow indicates interstitial inflammatory cell infiltration and fibrosis; in the colonic pathology pictures, the red boxes indicate structural defects in colonic epithelial tissue, epithelial cell damage and detachment, thinning of the mucosa, and shallow depth of the crypts; the red arrows indicate disappearance of superficial cup cells in the colonic mucosa and infiltration of inflammatory cells).
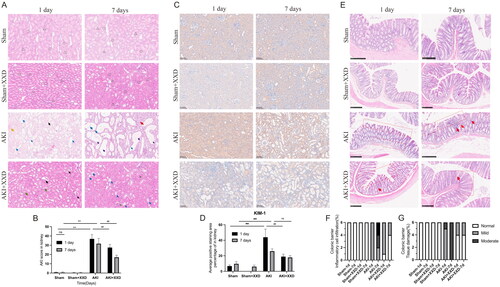
Drugs and disease corresponding targets
Through TCMSP database screening, ‘Huang Qi’ (Hedysarum Multijugum Maxim), ‘Chuan Niu Xi’ (Cyathulae Radix), ‘Tao Ren’ (Persicae Semen), ‘Da Huang’ (Radix Rhei Et Rhizome), and ‘Che Qian Cao’ (Plantaginis Herba), were searched, yielding a total of 73 potentially effective active components and 219 targets after removing duplicates. In addition, CNKI searched six chemical constituents of ‘Di Long’ (Pheretima vulgaris Clen), and two active constituents and eight targets were obtained after the screening with GI absorption results as ‘High’ and Lipinski results as ‘Yes.’ After converting to UniProt format and eliminating duplicate entries, a sum of 224 drug targets was acquired. See the data of active components of XXD in and . As shown in , 1778 AKI-related targets were obtained through the GeneCards, PharmGKB, DisGeNET, and TTD databases, including 140 targets possibly regulated by AKI in XXD.
Figure 2. (A) Xiaoyu Xiezhuo decoction components list; (B) Venn diagram of totally genes associated with AKI (Note. The green block indicates the related genes in DisGeNET; the pink block indicates the related genes in GeneCards; the blue block indicates the related genes in PharmGKB; the grey blocks indicate the related genes in TTD); (C) Venn diagram of intersection target between XXD and AKI (Note. The purple circle indicates the related genes in diseases; the yellow circle indicates the related genes in drug); (D) PPI network of Xiaoyu Xiezhuo decoction in treatment of AKI; (E) PPI screening process (Note. The yellow squares represent genes retained during the PPI screening process); (F) ‘XXD – active component – Target’ network (Note. The purple nodes indicate the active ingredients of traditional Chinese medicine present in each drug composition, the yellow nodes indicate the herbs corresponding to each active ingredient and the targets of XXD for the regulation of AKI are marked as red).
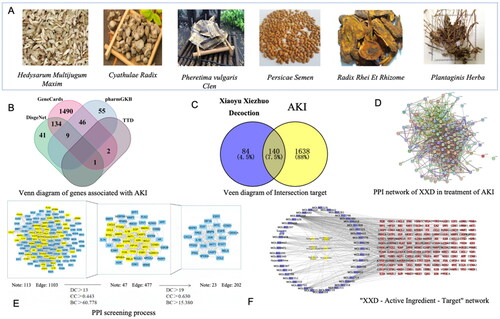
Table 2. Active components of XXD (top 20 of the core targets).
PPI predictions of core targets of XXD treating AKI
We imported the resulting 140 intersecting targets into the STRING database, where they were scored with an interaction score greater than 0.700. After hiding the free nodes, we constructed a PPI network for XXD in treating AKI (). The network has 131 nodes, with nine free nodes eliminated, and encompasses 1103 edges that depict the interaction relationship between the targets. We utilized Cytoscape software to import the data acquired from STRING. By employing the CytoNCA plugin, we conducted an initial filtering process and generated a sub-network by calculating the median values of node DC, BC, and CC. The median values were 13, 60.778, and 0.443, respectively. We subsequently determined the median DC, BC, and CC values for nodes within the sub-network to be 19, 15.380, and 0.630, respectively. These values were employed as the primary criteria during the second screening process to identify the essential targets of XXD in managing cases of AKI, as shown in . The screening process is shown in . We selected the core targets with the top five DC values in order of magnitude as the core targets: EGFR, TP53, AKT1, JUN, and IL1-β.
Table 3. The essential targets of XXD in AKI.
Drug-active component-disease target regulatory networks
The ‘Traditional Chinese Medicine-Active Component-Target’ network for treating AKI by XXD was constructed using Cytoscape software. shows the network, which contains 189 nodes and 505 edges. The network’s node degrees were computed and the resulting data were exported to Excel. We identified the top five potential active components in treating AKI by XXD. These components are quercetin, luteolin, kaempferol, baicalein, and isorhamnetin.
GO and KEGG predictions of key pathways of XXD treating AKI
The threshold used for screening core targets in R software for GO enrichment analysis was a significance level of p < .05. The resulting output consisted of 1978 entries related to GO, as depicted in . The number of genes is represented on the horizontal axis, while the GO entries are depicted on the vertical axis. The colors indicate the significance of enrichment, with redder colors indicating more significant gene enrichment. Among the entries, there were 2264 BPs primarily involved in BPs such as response to oxidative stress, reactive oxygen species, xenobiotic stimulus, and cellular response to chemical stress. In addition, there were 75 CCs, mainly related to the membrane raft, membrane microdomain, cyclin-dependent protein kinase holoenzyme complex, and serine/threonine protein kinase complex. Furthermore, there were 174 MFs, primarily involving DNA-binding transcription factor binding, RNA polymerase II-specific DNA-binding transcription factor binding, transcription coregulator binding, and ubiquitin-like protein ligase binding. The KEGG analysis yielded 149 entries, as shown in . The number of genes is represented on the horizontal axis, while the KEGG pathway is depicted on the vertical axis. The significance of gene enrichment is represented by the colors, with redder colors indicating more significant gene enrichment. The analysis revealed that the IL-17, TNF, p53, PI3K-Akt, and other signaling pathways were mainly involved. According to the KEGG data provided, a ‘KEGG pathway-gene’ network was created () consisting of 125 nodes, including 105 target genes and 20 KEGG pathways. The network is interconnected by a total of 538 edges. The network suggests that these 105 target genes regulate the 20 KEGG pathways that were enriched. The data were exported to Excel and organized based on the DC values, resulting in the identification of RELA, AKT1, TP53, MAPK1, and IKBKB as the top five genes. These genes regulated 17, 17, 16, 15, and 15 pathways, respectively.
Figure 3. (A) Column diagram of GO enrichment analysis; (B) column diagram of KEGG pathway enrichment analysis; (C) ‘KEGG pathway – gene’ network diagram (Note. KEGG pathway enrichment analysis. The column size represented the number of targets coincident with this path in the regulation of AKI by XXD and the color represented the p value of KEGG enrichment, the yellow nodes indicate KEGG pathway and the targets of XXD for the regulation of AKI are marked as green. KEGG: Kyoto Encyclopedia of Genes and Genomes).
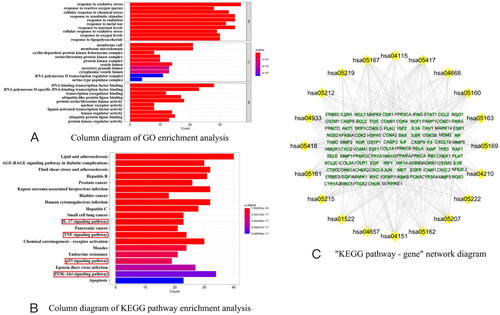
XXD improves renal function, urinary enzymes, and reduces serum intestinal metabolites and inflammatory factor release in AKI rats
The experiment results, shown in , indicate that Scr, serum BUN, and urinary NAG enzyme levels were higher in rats with AKI at 1 d and 7 d compared to the sham group at corresponding time points (p < .05). However, after XXD intervention, these indicators improved (p < .05). Serum Cys-c is a high-value and sensitive indicator in the clinical assessment of early kidney injury in patients with AKI. There was no statistically significant difference in Cys-c between the sham and sham + XXD groups (nsp > .05), but there was a statistically significant difference in the AKI group compared to the sham group (**p < .01). Cys-c was significantly lower in the AKI + XXD-1d group after one day of XXD intervention (##p < .01), but similar to the KIM-1 metrics, there was no significant difference between the AKI-7d group and the AKI + XXD-7d group (nsp > .05). Furthermore, the serum of AKI rats had significantly higher secretion of harmful toxins such as LPSs, d-LA, IS, and PCS (*p < .05 or **p < .01), as shown in . The protective factor of SCFAs decreased (*p < .05 or **p < .01), and this effect worsened over time. However, after XXD intervention, the expression of SCFAs increased, and the secretion of harmful toxins decreased (#p < .05 or ##p < .01). The AKI model rats exhibited a significant increase in the protein expression of inflammatory factors when compared to the sham group (*p < .05 or **p < .01). Significantly lower expression levels of IL-6, TNF-α, IL-1β, and IL-17A protein were seen in the 1 d and 7 d groups of the XXD treatment compared to the AKI group at corresponding time points (#p < .05 or ##p < .01, ).
Figure 4. (A) Surgical procedure diagram of the experiment design in the present study; (B) serum expression of Scr, BUN, and Cys-c; urinary expression of NAG enzyme; (C) serum metabolic indicators of intestinal origin LPS, d-LA, is, PCS, SCFAS; (D) expression of serum inflammatory factors TNF-α, IL-6, IL-17A, and IL1-β; (nsp > .05 vs. sham group; **p < .01 vs. sham group; #p < .05 or ##p < .01 vs. AKI group). NAG: N-acetyl-β-d-glucosaminidase.
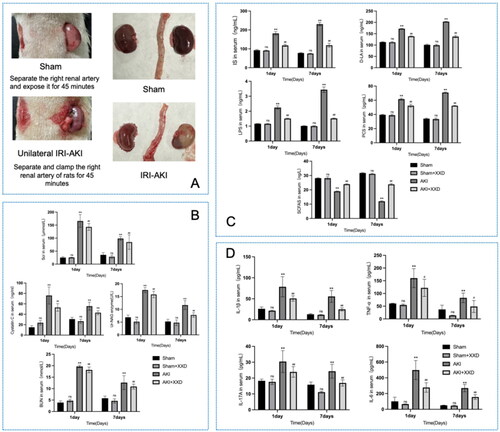
XXD regulates the TLR4/NF-κB/NLRP3 pathway to inhibit inflammatory genes in IRI-AKI
Protein expression of the TLR4/NF-κB/NLRP3 pathway was detected using IHC. The findings indicated a notable increase in the levels of these proteins among the AKI group of rats compared to the sham group (*p < .05 or **p < .01, ). After seven days of XXD intervention, significantly lower expression levels of TLR4, NF-κB, NLRP3, and IL-1β proteins were seen in the AKI + XXD group vs. the model group (#p < .05 or ##p < .01, ). The Western blotting results show that the ratio of phospho-NF-κB p65/NF-κB p65 in the AKI group is higher than in the sham group (**p < .01). After seven days of XXD intervention, trends in the above indicators showed a significant decrease (##p < .01, ). The levels of IL-6, TNF-α, and IL-17A mRNA were assessed in the renal tissues of rats, and significantly increased mRNA expression of these inflammatory factors was shown in the AKI group rats vs. the sham group. A time-dependent effect was observed (*p < .05 or **p < .01, ). However, significantly lower mRNA expression of IL-6, TNF-α, IL-1β, and IL-17A proteins in the 1 d and 7 d groups of the AKI + XXD group was shown vs. the AKI group at corresponding time points (#p < .05 or ##p < .01, ).
Figure 5. (A) Expression of TLR4/NF-κB/NLRP3/IL1-β of renal tissue (IHC staining ×200); (B) expression of p- NF-κB/NF-κB of renal tissue; (C) mRNA expression of IL-6, TNF-α, and IL-17A in the renal tissue of rat in each treatment group (nsp > .05 vs. sham group; *p < .05 or **p < .01 vs. sham group; #p < .05 or ##p < .01 vs. AKI group).
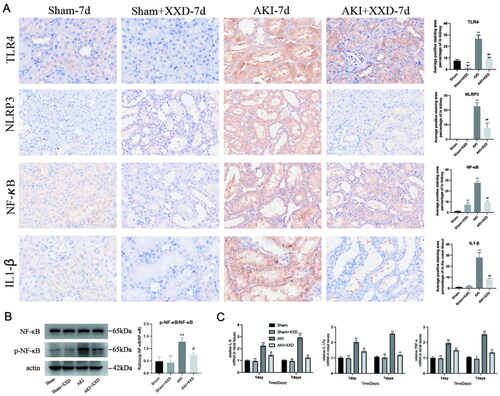
XXD modulation of TLR4/NF-κB/NLRP3 pathway protects intestinal mucosal barrier in AKI rats
The IHC technique was utilized to investigate the protein expression of claudin, occludin, and ZO-1, which play a crucial role in maintaining the integrity of the intestinal mucosal barrier, and significantly lower expression was shown in the AKI group of rats vs. the sham group (*p < .05 or **p < .01, ). After seven days of XXD intervention, significantly higher expression levels of these proteins were seen in the AKI + XXD group vs. the AKI group (#p < .05 or ##p < .01, ). Furthermore, IHC was used to detect the protein expression of the TLR4/NF-κB/NLRP3 pathway. The AKI group of rats exhibited a notably elevated expression of these proteins compared to the sham group (*p < .05 or **p < .01, ). However, after seven days of XXD intervention, the AKI + XXD group exhibited significantly reduced expression levels of TLR4, NF-κB, NLRP3, and IL-1β proteins compared to the AKI group (#p < .05 or ##p < .01, ). Additionally, the levels of inflammatory and chemotactic factors in the colon tissue of rats were analyzed by measuring mRNA expression. The AKI group rats exhibited a significant upregulation in the mRNA expression levels of IL-6, TNF-α, IL-17A, ICAM-1, MCP-1, and MIP-2 compared to the sham group (*p < .05 or **p < .01, ). However, the AKI + XXD group exhibited a notable decrease in mRNA expression of these genes at 1 d and 7 d compared to the AKI group during corresponding time intervals (#p < .05 or ##p < .01, ).
Figure 6. (A) Expression of intestinal barrier markers (claudin-1, occludin, and ZO-l) and TLR4/NF-κB/NLRP3/IL1-β (IHC staining ×200); (B) mRNA expression of IL-6, TNF-α, IL-17A, ICAM-1, MCP-1, and MIP-2 in the colon tissue of rat in each treatment group (nsp > .05 vs. sham group; **p < .01 vs. sham group; ##p < .01 vs. AKI group).
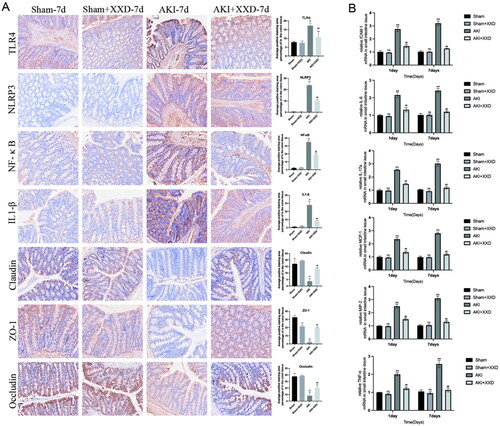
XXD improves spectrum of intestinal flora in AKI rats
Our study assessed the impacts of different treatments on gut microbial communities in rats with AKI. We used 16S rRNA gene sequencing analysis of cecum contents to assess microbial community abundance and homogeneity. The Chao1 index was significantly reduced in the AKI group but significantly enhanced in the sham group or the AKI + XXD group (p < .05; p < .01) (). To visualize the beta diversity and evaluate variations in species complexity, we conducted principal component analysis (PCA) using weighted UniFrac distances at the OTU level (). The gut microbial community structure of the seven-day dosing and one-day dosing groups was significantly different from that of the model group, except for no significant difference between 1 d and 7 d dosing.
Figure 7. (A) Chao index analysis; (B) principal component analysis (PCA); (C) species composition at the phylum level; (D) species composition at genus level.
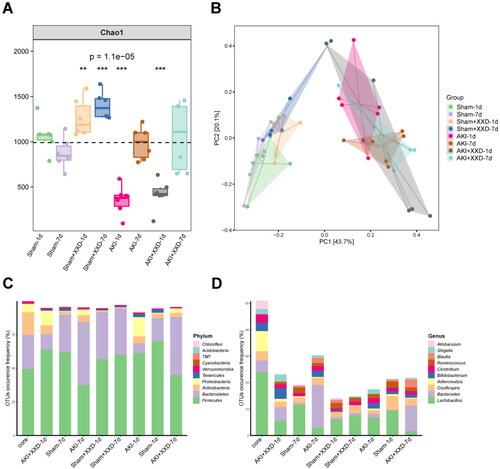
Our results demonstrated significant differences in the abundance of some specific bacteria at the group level, even though the gut microbial community structure was similar between groups at the overall level (). The LEfSe survey identified bacterial communities that were highly affected in the study group (). Microbial biomarkers with statistically significant differences depending on relative abundance were shown in the branching diagrams and histograms of the distribution of LDA values (). We compared the species composition differences between samples and achieved species abundance distributions across samples by analyzing the top 50 bacterial genera in terms of mean abundance using heatmaps (). The AKI group exhibited a notable decrease in the relative abundance of Turicibacter, Aggregatibacter, and Alistipes, whereas there was a significant increase in the relative abundance of Melissococcus microbiota compared to the sham group. However, the administration of XXD significantly reversed these alterations in microbiota composition. By employing PICRUSt for flora function prediction in each group, we visualized most of the functional pathways altered in the AKI group, with some being reverse-regulated after treatment (). Notably, KEGG pathway enrichment analyses showed that immunity and inflammation of infections and metabolic pathways are associated with amino acid metabolism, carbohydrate metabolism, energy metabolism, and lipid metabolism.
Discussion
Piling up studies have shown that approximately 36–70% of end-stage renal disease patients exhibit gut dysfunction [Citation34], confirming changes in gut microbiota composition and structure, impaired gut barrier function, and increased entry of harmful metabolites into the bloodstream, leading to exacerbated inflammatory response and the progression of kidney dysfunction [Citation35]. Recently, a growing body of research findings has indicated that gut microbiota and its metabolic products also contribute significantly to the advancement of AKI [Citation34]. Therefore, the impact and role of gut microbiota on AKI are expected to become a new direction for AKI treatment. AKI is also associated with a high and low volume load, endotoxin, and macromolecules to circulate. Persistent dysfunction of the intestinal epithelial barrier, leading to compromised permeability and integrity [Citation36], facilitates the secretion of inflammatory agents and the migration of bacteria and LPS. In this study, the AKI model was established through the U-IRI method in our pharmacodynamic study. The clinical manifestations, laboratory parameters, immune inflammation, and pathological changes are similar to human AKI. The success could be judged from the kidney and colon pathological damage score and biomarkers of renal damage, such as KIM-1, after treatment with XXD, which guaranteed the reliability of the subsequent study results.
In the present study, the network relationship of the ‘drug-active component-disease target’ was constructed through the network pharmacological method, where the potential active components contained within XXD for treating AKI were found. Among those in this list were quercetin, beta-sitosterol, kaempferol, luteolin, 7-O-methylisomucronulatol, hederagenin, baicalein, formononetin, isorhamnetin, and stigmasterol. Quercetin belongs to a group of flavonoids known for their documented abilities in reducing inflammation and providing antioxidant benefits [Citation37]. Mahmoud Hashemi et al. [Citation38] previously found that quercetin can reduce the production of inflammatory cytokines and IL-17 by inhibiting the Toll-like receptor (TLR) 4/MAPK signaling pathway, thereby reducing the polarization of T helper cells 17 (Th17) and inhibiting the inflammatory response. In addition, core targets of XXD in treating AKI found in our study include EGFR, TP53, AKT1, JUN, IL1-β, CASP3, HIF1-α, MMP9, MYC, FOS, BCL2, CXCL8, PTGS2, STAT1, CCND1, RELA, ESR1, EGF, CDKN1A, CCL2, IFN-γ, NFKBIA, and MAPK1 following analysis of the PPI network. The KEGG findings revealed that the primary targets were predominantly enriched in pathways associated with inflammation, including but not limited to the IL-17 signaling pathway, TNF signaling pathway, p53 signaling pathway, and PI3K-Akt signaling pathway.
Combining the above network pharmacological analyses, we preliminarily validated the biochemical indicators, expression of IL-17- and TNF-a-related inflammatory factors, and intestinal metabolites in serum. As shown in our study, XXD improves not only renal function, urinary enzymes, Cys-c, and serum inflammatory factors such as IL-17, TNF-α, IL-6, and IL1-β but also intestinal metabolites including LPS, d-LA, IS, PCS, and SCFAs. Under an optical microscope, XXD ameliorates renal and colonic pathological injury in AKI rats, reducing the AKI score, renal pathological damage, improving the intestinal mucosa’s inflammatory infiltration, and repairing the components of the mucosal barrier named claudin-1, occludin, and ZO-1. In general, the curative effect of this TCM is time-dependent. We suggested that receiving XXD orally has the potential to ameliorate the inflammatory condition of AKI rats as a whole.
Regarding indicators of inflammatory factors, IL-17A protein has cytokine properties and can induce NF-κB activation. It has been demonstrated that serum levels of IL-17A, when measured upon AKI diagnosis or ICU admission, exhibit a notable elevation in critically ill individuals with AKI compared to those without this condition [Citation39]. The intestinal epithelial barrier [Citation40] can be described as a tripartite mechanism comprising a biological, physical, and immune component. AKI triggers an inflammatory response with high-volume loading, which alters the mesenteric vascular bed’s permeability and promotes intestinal edema formation, resulting in a disruption of the TJ and a reduction of intestinal epithelial survival. Changes in the TJ increase the permeability of the physical barrier, allowing for the spillover of bacteria, endotoxins, and macromolecules into the circulation. Claudin-1, occludin, and ZO-1, as essential components of the intestinal barrier, are critical players in intestinal permeability. In contrast, serum d-LA level indirectly reflects the degree of intestinal mucosal damage and changes in permeability. When there is an elevation in the permeability of the intestinal mucosa, d-LA gains access to the bloodstream via the compromised mucosal barrier, and the elevated d-LA level can be an essential indicator of the breakdown of the intestinal mucosal barrier. In the present study, LPSs, an enterogenic endotoxin, along with phenol sulfate and PCS, are uremic toxins associated with the intestinal flora. Additionally, d-LA, as mentioned above, is an indicator of intestinal barrier function. SCFAs are produced by the fermentation of intestinal anaerobic bacteria, with acetic acid, propionic acid, and butyric acid accounting for about 95% of the total. SCFAs provide a large portion of the energy required by colonic epithelial cells. SCFAs promote cell morphology and functional stability, positively regulate immune inflammation, and strengthen the intestinal mucosal barrier’s protective effect. Short-chain fatty acids positively protect against acute renal tubular injury in the AKI mouse model. Conversely, colonization by harmful gut microorganisms can accelerate the progression of renal disease by producing excessive uremic toxins.
During shock, intestinal hypoperfusion, and intestinal edema, the gut is a potentiator in causing systemic inflammatory response syndromes, and gut exacerbation of the systemic inflammatory response, in turn, exacerbates AKI, causing a vicious cycle. It appears that inflammation and its pathway response are important factors linking the gut–kidney axis. At the same time, we found that in AKI, the basic targets of XXD () include core genes such as RELA (nuclear factor NF-Kappa-B P65 subunit), CCL2 (MCP-1), and NFKBIA (NFκB inhibitor alpha). We experimentally verified that XXD may inhibit the phosphorylation of NF-κB protein, reduce the inflammatory response, and depress inflammatory or chemokine factors, alleviating systemic or local inflammatory responses [Citation41].
AKI leads to tissue damage and inflammatory responses [Citation42] that trigger pathogen-associated molecular patterns (PAMPs) and damage-associated molecular patterns (DAMPs), including hypoxia-inducible factor (HIF), high mobility group protein 1 (HMGP1), upregulated leukocyte adhesion molecules, TNF, and chemokines, and driver pattern recognition receptors (PRRs) such as T cells. PAMPs and DAMPs include HIF, HMGP1, which induces the up-regulation of leukocyte adhesion molecules, TNF, and chemokines, and drives the signal transduction of PRRs, such as the TLR, NOD-like receptor (NLR), etc., resulting in significant leukocyte extravasation and rapid infiltration leading to multiple programmed cell deaths and kidney damage. TLR4 [Citation43] is an essential protein involved in the inflammatory response in vivo and a vital protein molecule connecting specific and nonspecific immunity in vivo, mainly distributed on the surface of immune cells such as monocytes, polymorphonuclear cells, T cells, and B cells. Inflammatory responses induce the production and release of more pro-inflammatory cytokines, adhesion molecules, and other inflammatory mediators. The TLR4/NF-κB pathway is associated with the inflammatory response and immune response in vivo, and NF-κB activates the NOD-like receptor protein 3 (NLRP3) inflammatory vesicle, which promotes the release of IL-1β. The critical role of NLRP3 inflammatory vesicles in regulating renal inflammation is demonstrated in different models of renal disease, including I/R injury [Citation44]. Previous studies [Citation45,Citation46] showed that necrotic renal tubular cells could activate NLRP3 inflammatory vesicles in macrophages by releasing live mitochondria. NLRP3 depletion exhibited a protective effect against renal inflammation and tissue injury after I/R injury. The deficiency of NLRP3 in renal tubular cells enhanced regenerative reactions. These results indicate that targeting NLRP3 inflammatory cysts could be a promising approach for the treatment of renal ischemia/reperfusion injury.
The findings of this study suggest that modulation of the TLR4/NF-κB/NLRP3 pathway by XXD protects both the intestinal mucosal barrier and kidney injury in AKI rats. Moreover, increased enrichment of chemokine genes, such as MCP-1 and MIP-2, which recruit leukocytes to the injury site, was observed at the colonic site in the AKI group. Additionally, increased enrichment of genes for endothelial and leukocyte adhesion molecules, such as ICAM-1, also promotes leukocyte and immune cell recruitment to the area of injury by interacting with endothelial cells, which was reduced by XXD treatment. The KEGG results of the related intestinal microflora after XXD treatment showed a close correlation with immunity, infection, and inflammation compared with the AKI group, whose hypothesis was verified by our animal experiments described above. Among these, the treatment group increased the relative abundance of Alistipes, which aligns with the findings of prior studies [Citation47]. The relative abundance of Turicibacter significantly decreased in the AKI group and increased after treatment; intestinal probiotics can regulate glucose, lipid metabolism, and bile acid metabolism through the influence of polysaccharide substances, which can positively affect body immunity.
Limitations
There are limitations, such as the delayed database update and incomplete inclusion degree; further verification should be performed based on continuous supplementation. Moreover, we have only verified potential targets and pathways of XXD in animal experiments; further verification should involve using inhibitors of the TLR4/NF-κB/NLRP3 pathway to further clarify the effects of the formula. The next aim should be to clarify the specific microorganism mechanism of XXD during AKI therapy to enhance the theoretical foundation for the effective management of AKI in clinical settings. For example, comparing efficacy in AKI by fecal microbiota transplantation (FMT) for XXD treatment can be considered for future experiments.
Author contributions
SCH and HBC designed the study; YJ and YMX performed the experiments and wrote the paper. SPL and YHF analyzed the data. YZC and BY calibrated the data and revised the paper. All authors revised and approved the final version of this manuscript.
Ethical approval
The current research received ethical approval from the Medical and Experimental Animal Ethics Committee of Zhejiang University of traditional Chinese Medicine (approval no. IAUCU-202111-32).
Disclosure statement
No potential conflict of interest was reported by the author(s).
Data availability statement
The datasets utilized and/or examined in the present investigation can be obtained upon a reasonable solicitation from the corresponding author.
Additional information
Funding
References
- Acute kidney injury. Nat Rev Dis Primers. 2021;7(1):1. doi: 10.1038/s41572-021-00291-0.
- Monard C, Bianchi N, Kelevina T, et al. Epidemiology and outcomes of early versus late septic acute kidney injury in critically ill patients: a retrospective cohort study. Anaesth Crit Care Pain Med. 2023;43(1):101332. doi: 10.1016/j.accpm.2023.101332.
- Suren K, Caroline A, Sheetal B, et al. Clinical practice guideline for acute kidney injury (AKI). London: The UK Kidney Association; 2019.
- Stanski NL, Basu RK, Cvijanovich NZ, et al. External validation of the modified sepsis renal angina index for prediction of severe acute kidney injury in children with septic shock. Crit Care. 2023;27(1):463. doi: 10.1186/s13054-023-04746-6.
- Meng Y, Lin Y, Zhang JW, et al. Aspirin intervention before ICU admission reduced the mortality in critically ill patients with acute kidney injury: results from the MIMIC-IV. Front Pharmacol. 2023;14:1292745. doi: 10.3389/fphar.2023.1292745.
- Cui J, Bai X, Chen X. Autophagy and acute kidney injury. Adv Exp Med Biol. 2020;1207:469–18. doi: 10.1007/978-981-15-4272-5_34.
- Xu Y, Jiang W, Zhong L, et al. miR-195-5p alleviates acute kidney injury through repression of inflammation and oxidative stress by targeting vascular endothelial growth factor A. Aging. 2020;12(11):10235–10245. doi: 10.18632/aging.103160.
- Zhong J, Yang HC, Yermalitsky V, et al. Kidney injury-mediated disruption of intestinal lymphatics involves dicarbonyl-modified lipoproteins. Kidney Int. 2021;100(3):585–596. doi: 10.1016/j.kint.2021.05.028.
- Lohia S, Vlahou A, Zoidakis J. Microbiome in chronic kidney disease (CKD): an omics perspective. Toxins. 2022;14(3):176. doi: 10.3390/toxins14030176.
- Rysz J, Franczyk B, Ławiński J, et al. The impact of CKD on uremic toxins and gut microbiota. Toxins. 2021;13(4):252. doi: 10.3390/toxins13040252.
- Kuo WT, Odenwald MA, Turner JR, et al. Tight junction proteins occludin and ZO-1 as regulators of epithelial proliferation and survival. Ann N Y Acad Sci. 2022;1514(1):21–33.
- Venugopal S, Anwer S, Szászi K. Claudin-2: roles beyond permeability functions. Int J Mol Sci. 2019;20(22):5655.
- Chou YT, Kan WC, Shiao CC. Acute kidney injury and gut dysbiosis: a narrative review focus on pathophysiology and treatment. Int J Mol Sci. 2022;23(7):3658.
- Wang X, Yang S, Li S, et al. Aberrant gut microbiota alters host metabolome and impacts renal failure in humans and rodents. Gut. 2020;69(12):2131–2142. doi: 10.1136/gutjnl-2019-319766.
- Sato N, Kakuta M, Hasegawa T, et al. Metagenomic profiling of gut microbiome in early chronic kidney disease. Nephrol Dial Transplant. 2021;36(9):1675–1684. doi: 10.1093/ndt/gfaa122.
- Lloréns-Rico V, Vieira-Silva S, Gonçalves PJ, et al. Bench marking microbiome transformations favors experimental quantitative approaches to address compositionality and sampling depth biases. Nat Commun. 2021;12(1):3562. doi: 10.1038/s41467-021-23821-6.
- Zha Z, Wang X, Wang G, et al. Synthesis and structural characterization of xylan acetate ester and its antinephritic effects in rats with experimental chronic kidney disease. Int J Biol Macromol. 2023;240:124413. doi: 10.1016/j.ijbiomac.2023.124413.
- Girdhar K, Dogru YD, Huang Q, et al. Dynamics of the gut microbiome, IgA response, and plasma metabolome in the development of pediatric celiac disease. Microbiome. 2023;11(1):9. doi: 10.1186/s40168-022-01429-2.
- Wu W, Wang W, Liang L, et al. Treatment with quercetin inhibits SARS-CoV-2 N protein-induced acute kidney injury by blocking Smad3-dependent G1 cell-cycle arrest. Mol Ther. 2023;31(2):344–361.
- Islam MS, Miao L, Yu H, et al. Ethanol extract of Illicium henryi attenuates LPS-induced acute kidney injury in mice via regulating inflammation and oxidative stress. Nutrients. 2019;11(6):1412. doi: 10.3390/nu11061412.
- Lu K, Xie Z, Hongzhen MA. Effects of Xiaoyu Xiezhuo Yin on the expression of TGF-β1 of renal tissue in rat with UUO. Chin J Integr Tradit West Nephrol. 2011;12(8):665–668.
- Lu K-D, Zhang B-B, Xie Z-J, et al. The protective effect of Xiaoyu Xiezhuo Yin on kidney of renal interstitial fibrosis rats. Chin Archiv Tradit Chin Med. 2011;7(29):1537–1540.
- Ye Q, Chen H, Ma H, et al. Xiaoyu Xiezhuo Drink protects against ischemia–reperfusion acute kidney injury in aged mice through inhibiting the TGF-β1/Smad3 and HIF1 signaling pathways. Biomed Res Int. 2021;2021:9963732.
- Kurata Y, Nangaku M. Use of antibiotics as a therapeutic approach to prevent AKI-to-CKD progression. Kidney Int. 2023;104(3):418–420.
- Guo W, Huang J, Wang N, et al. Integrating network pharmacology and pharmacological evaluation for deciphering the action mechanism of herbal formula Zuojin pill in suppressing hepatocellular carcinoma. Front Pharmacol. 2019;10:1185. doi: 10.3389/fphar.2019.01185.
- Szklarczyk D, Gable AL, Lyon D, et al. STRING v11: protein–protein association networks with increased coverage, supporting functional discovery in genome-wide experimental datasets. Nucleic Acids Res. 2019;47(D1):D607–D613. doi: 10.1093/nar/gky1131.
- Zhang J, Zhou Y, Ma Z. Multi-target mechanism of Tripterygium wilfordii Hook for treatment of ankylosing spondylitis based on network pharmacology and molecular docking. Ann Med. 2021;53(1):1090–1098. doi: 10.1080/07853890.2021.1918345.
- Wei Q, Dong Z. Mouse model of ischemic acute kidney injury: technical notes and tricks. Am J Physiol Renal Physiol. 2012;303(11):F1487–F1494.
- Liu D, Lun L, Huang Q, et al. Youthful systemic milieu alleviates renal ischemia–reperfusion injury in elderly mice. Kidney Int. 2018;94(2):268–279. doi: 10.1016/j.kint.2018.03.019.
- Han Y, Wang X, Cheng X, et al. Close homolog of L1 deficiency exacerbated intestinal epithelial barrier function in mouse model of dextran sulfate sodium-induced colitis. Front Physiol. 2020;11:584508. doi: 10.3389/fphys.2020.584508.
- Gao Y, Yang R, Guo L, et al. Qing-Re-Xiao-Zheng formula modulates gut microbiota and inhibits inflammation in mice with diabetic kidney disease. Front Med. 2021;8:719950. doi: 10.3389/fmed.2021.719950.
- Özkurt E, Fritscher J, Soranzo N, et al. LotuS2: an ultrafast and highly accurate tool for amplicon sequencing analysis. Microbiome. 2022;10(1):176; Felizardo RJF, Watanabe IKM, Dardi P, et al. The interplay among gut microbiota, hypertension and kidney diseases: the role of short-chain fatty acids. Pharmacol Res. 2019;141:366–377.
- Kozik AJ, Begley LA, Lugogo N, et al. Airway microbiota and immune mediator relationships differ in obesity and asthma. J Allergy Clin Immunol. 2023;151(4):931–942. doi: 10.1016/j.jaci.2022.11.024.
- Saranya GR, Viswanathan P. Gut microbiota dysbiosis in AKI to CKD transition. Biomed Pharmacother. 2023;161:114447. doi: 10.1016/j.biopha.2023.114447.
- Andrianova NV, Popkov VA, Klimenko NS, et al. Microbiome–metabolome signature of acute kidney injury. Metabolites. 2020;10(4):142. doi: 10.3390/metabo10040142.
- Gong J, Noel S, Pluznick JL, et al. Gut microbiota-kidney cross-talk in acute kidney injury. Semin Nephrol. 2019;39(1):107–116. doi: 10.1016/j.semnephrol.2018.10.009.
- Marunaka Y, Marunaka R, Sun H, et al. Actions of quercetin, a polyphenol, on blood pressure. Molecules. 2017;22(2):209. doi: 10.3390/molecules22020209.
- Mahmoud Hashemi A, Solahaye Kahnamouii S, Aghajani H, et al. Quercetin decreases Th17 production by down-regulation of MAPK–TLR4 signaling path on T cells in dental pulpitis. J Dent. 2018;19(4):259–264.
- Collett JA, Ortiz-Soriano V, Li X, et al. Serum IL-17 levels are higher in critically ill patients with AKI and associated with worse outcomes. Crit Care. 2022;26(1):107. doi: 10.1186/s13054-022-03976-4.
- Linh HT, Iwata Y, Senda Y, et al. Intestinal bacterial translocation contributes to diabetic kidney disease. J Am Soc Nephrol. 2022;33(6):1105–1119. doi: 10.1681/ASN.2021060843.
- Cai S, Chen J, Li Y. Dioscin protects against diabetic nephropathy by inhibiting renal inflammation through TLR4/NF-κB pathway in mice. Immunobiology. 2020;225(3):151941. doi: 10.1016/j.imbio.2020.151941.
- Zheng Z, Xu K, Li C, et al. NLRP3 associated with chronic kidney disease progression after ischemia/reperfusion-induced acute kidney injury. Cell Death Discov. 2021;7(1):324. doi: 10.1038/s41420-021-00719-2.
- Ren Q, Guo F, Tao S, et al. Flavonoid fisetin alleviates kidney inflammation and apoptosis via inhibiting Src-mediated NF-κB p65 and MAPK signaling pathways in septic AKI mice. Biomed Pharmacother. 2020;122:109772. doi: 10.1016/j.biopha.2019.109772.
- Yin F, Zheng PQ, Zhao LQ, et al. Caspase-11 promotes NLRP3 inflammasome activation via the cleavage of pannexin1 in acute kidney disease. Acta Pharmacol Sin. 2022;43(1):86–95. doi: 10.1038/s41401-021-00619-2.
- Li S, Lin Q, Shao X, et al. NLRP3 inflammasome inhibition attenuates cisplatin-induced renal fibrosis by decreasing oxidative stress and inflammation. Exp Cell Res. 2019;383(1):111488. doi: 10.1016/j.yexcr.2019.07.001.
- Lin Q, Li S, Jiang N, et al. Inhibiting NLRP3 inflammasome attenuates apoptosis in contrast-induced acute kidney injury through the upregulation of HIF1A and BNIP3-mediated mitophagy. Autophagy. 2021;17(10):2975–2990. doi: 10.1080/15548627.2020.1848971.
- Gharaie S, Lee K, Newman-Rivera AM, et al. Microbiome modulation after severe acute kidney injury accelerates functional recovery and decreases kidney fibrosis. Kidney Int. 2023;104(3):470–491. doi: 10.1016/j.kint.2023.03.024.