ABSTRACT
It has been argued that, throughout the Mesozoic, the immature growth forms of megaherbivorous dinosaurs competitively excluded small herbivorous dinosaur species, leading to the left-skewed species richness-body mass distributions of their fossil assemblages. By corollary, where large and small herbivores coexisted over a geologically significant period of time, they must have exhibited niche partitioning. We use multivariate ecomorphological analysis of the Late Cretaceous ornithischian dinosaur assemblage of North America to examine this prediction. Our results indicate good ecomorphological separation of most, but not all, species at small body size, although more work is required to demonstrate that these patterns were adaptive. Calculation of browse profiles using corrected abundance data and bracketed estimates of energy requirements suggests that immature megaherbivores – most particularly hadrosaurids – outstripped coexisting small ornithischian species in their control of the resource base.
Introduction
An ontogenetic niche shift (ONS) is a phenomenon whereby individuals of a species undergo a change in diet (or some other niche variable, such as habitat) with growth. Such shifts are widespread among living animals, and are often most pronounced in species whose individuals either undergo metamorphosis (e.g. various insects and amphibians) or grow slowly and continuously throughout their lifetimes (Werner and Gilliam Citation1984; Ebenman Citation1992; Claessen and Dieckmann Citation2001). Commonly cited examples employing the latter strategy are crocodilians, whose populations are highly size-structured, and whose diets incorporate increasingly fewer invertebrates and increasingly more (and larger) fishes and other vertebrates with age (Dodson Citation1975; Platt et al. Citation2006; Subalusky et al. Citation2009). Some clades characterised by a more rapid attainment of sexual and somatic maturity, such as birds, can also exhibit ONSs (McLeay et al. Citation2009; Carravieri et al. Citation2017) but these examples are not as widely documented.
Ontogenetic niche shifts can have significant outcomes on community ecology. These may have the effect of linking food webs (e.g. pelagic and benthic food webs in aquatic systems), pollination and herbivory networks, and geographically isolated metacommunities (e.g. aquatic and terrestrial) (Nakazawa Citation2014). They may likewise stabilise consumer-resource dynamics, alter conditions for the coexistence of predators, and modify trophic cascades (Miller and Rudolf Citation2011). Interspecific competition driven by ONSs can negatively impact community richness via competitive exclusion or compel niche partitioning (Sánchez‐Hernández et al. Citation2019).
Many non-avian dinosaurs (hereafter, simply ‘dinosaurs’) are thought to have experienced an ONS, due to their distinctive life histories. Varricchio (Citation2011)cited five factors that, in combination, might have contributed to such a shift: a mobile terrestrial lifestyle, oviparity, parental care, multi-year maturation, and juvenile sociality. For species represented by good growth series, ontogenetic variation in craniodental ecomorphology and enamel isotopes have been cited as evidence for the existence of ONSs among various theropods, sauropods, and ornithischians (e.g. Erickson and Zelenitsky Citation2014; Wang et al. Citation2017; Woodruff et al. Citation2018; Frederickson et al. Citation2020; Wyenberg-Henzler Citation2020; Therrien et al. Citation2021).
Ontogenetic niche shifts are thought by some to have played a role in structuring dinosaur communities (O’Gorman and Hone Citation2012; Codron et al. Citation2012a, Citation2013; Schroeder et al. Citation2021; Holtz Citation2021). These authors have argued that the left-skewed body size-species richness distributions of various dinosaur assemblages is reflective of size-mediated resource competition between small species and the immature forms of larger ones (contrast this with modern mammalian communities, where large herbivore young are suckled by their mothers, and so do not directly compete with smaller species for dietary resources). The hypothesis maintains that dietary competition from the juveniles of large-bodied species suppressed the diversity of small-bodied species in dinosaur-dominated ecosystems. The former underwent a shift in dietary ecology with growth to occupy a distinct niche at maturity, thereby offering them an escape from such ‘competition traps’ (sensu Codron et al. Citation2012a) and so a competitive advantage. In this way, these researchers have argued that, throughout the Mesozoic, competitive pressures at small body sizes maintained the left-skewed body size-species richness distributions of many dinosaur fossil assemblages.
As summarised above, the size-mediated competition hypothesis hinges on two key premises:
The left-skewed species richness-body size distributions of the various dinosaur fossil assemblages (taphocoenoses) reflect the shape that characterised the original life assemblages (biocoenoses).
Competition at small body sizes characterised the ecological interactions of many dinosaur species; the relatively few small species that were able to coexist alongside the juveniles of larger species did so because of their unique occupation of niche space, having either different fundamental or realised niches. As stated by Codron et al. (Citation2013, p. 7): ‘A key assumption of our model is that similar-sized individuals occupy overlapping niche space, and that predators and competitors are strongly influenced by this. While links between body size and niche occupancy should be expected, morphological, physiological, and behavioural constraints could easily dictate an individual’s realised niches and – in theory – lead to niche separation between individuals/species of similar size’.
Premise (1) above is highly contentious. Dinosaur fossil assemblages (taphocoenoses) are indeed frequently left-skewed but suffer from a demonstrable taphonomic size-bias (Farlow et al. Citation1995; Brown et al. Citation2012a, Citation2013b; Evans et al. Citation2013; Benson Citation2018; Brown et al. Citation2021) (but see Leach et al. Citation2021 for a notable exception). Thus, the size distributions of the original living assemblages (biocoenoses) may have been normal or even right-skewed, typical of most modern animal assemblages (Brown Citation1995; Gaston and Blackburn Citation2000; Lyons and Smith Citation2013). In a reply to criticism of their methods (Brown et al. Citation2012a), Codron et al. (Citation2012b) continued to defend the explanatory power of their population model and the body size data used to validate its projections.
Premise (2) has not been subjected to the same degree of scrutiny, and so forms the impetus for the current study. We do not, however, aver to be able to conclusively test its predictions. The effects of ecological competition are difficult to demonstrate in the fossil record (Benton Citation1996), in part owing to the associated problems of establishing both fundamental niche overlaps among fossil organisms and ancient resource limitation. The timescale over which ecological competition occurs (~100–101 yr) is also considerably shorter than that typically discernible within the fossil record (~103–106 yr) (Rull Citation2014). These difficulties compound to hinder our ability to make decisive conclusions about the action of competition at any particular time in any particular fossil community (although it may be possible in some instances to measure the outcomes with good fossil data: e.g. Janis et al. Citation1994; Tyler and Leighton Citation2011; Mallon Citation2019).
Nonetheless, we reason that if competition at small body sizes was in fact a major driver of dinosaur community structure, the long-term evolutionary effects should be most apparent in those assemblages that maintained their basic ecological structure over a geologically significant period of time, termed a ‘chronofauna’ (Olson Citation1952). The Late Cretaceous ornithischian assemblage of western North America meets these criteria (Brinkman et al. Citation2004; Mallon Citation2019), consisting of small (leptoceratopsids, pachycephalosaurids, thescelosaurids) and large (ankylosaurs, ceratopsids, hadrosaurids) herbivores, all of which coexisted throughout the ~10 Myr duration of the assemblage. In this study, we use ecomorphology to examine the question of whether these long-sympatric groups occupied distinct regions of niche hyperspace, particularly at small body sizes, as we might expect for a chronofauna purportedly shaped by the effects of resource competition.
We further reason that, if young megaherbivores were important competitors at small body sizes, they collectively should have exerted a substantial browsing pressure on the Late Cretaceous landscape. Coe et al. (Citation1987) used corrected abundance and metabolic data to reconstruct ‘browse profiles’ for Jurassic and Cretaceous ecosystems, but they did not consider the contribution of small herbivorous dinosaurs, nor did they model the demographics (e.g. representation of growth stages) of the examined megaherbivores. We build on their pioneering work here, using new data made available since their original study. We then assess the implications of such browse profiles for the size-mediated competition hypothesis.
A comment on terminology
Many of the studies cited above use the terms ‘small’ and ‘large’ in different ways. Codron et al. (Citation2012a, Citation2013) contrasted dinosaur body sizes with those of contemporaneous mammals, and considered the latter as ‘small’ (<1 kg). Dinosaurs were considered to occupy the ‘intermediate’ (1–1,000 kg) and ‘large’ (>1,000 kg) size categories. By contrast, in a study of dinosaur taphonomy within the Upper Cretaceous Dinosaur Park Formation, Brown et al. (Citation2013b) considered individuals <60 kg to be ‘small’, because this was the size threshold below which taphonomic bias acted most strongly.
Here, we distinguish between ‘large’ and ‘small’ dinosaurs, for the sake of contrast. Large dinosaurs are those ≥1,000 kg, otherwise termed ‘megaherbivores’ for those plant-eating species (Owen-Smith Citation1988). Dinosaurs weighing <1,000 kg are here considered to be small, although some exceeded 400 kg (and so might reasonably be considered ‘intermediate’ in size). For our purposes, we do not refer to an ‘intermediate’ size category but acknowledge that this may have been an ecologically important category, distinct from smaller and larger forms.
Variable abbreviations – cp-jj, coronoid process to jaw joint distance; dh, dentary height; dl, diastema length; dt-mq, distal tooth row to mid quadrate distance; oh, occiput height; ppb, paroccipital process breadth; qb, quadrate breadth; sd, snout depression below occlusal plane; sk, skull height; sl, snout length; sw, snout width; trl, tooth row length.
Institutional abbreviations – AMNH, American Museum of Natural History, New York, New York; CMN, Canadian Museum of Nature, Ottawa, Ontario; GPDM, Great Plains Dinosaur Museum, Malta, Montana; MOR, Museum of the Rockies, Bozeman, Montana; ROM, Royal Ontario Museum, Toronto, Ontario; RSM, Royal Saskatchewan Museum, Regina, Saskatchewan; TMP, Royal Tyrrell Museum of Palaeontology, Drumheller, Alberta; UALVP, University of Alberta, Edmonton, Alberta; UC, University of Calgary, Calgary, Alberta; USNM, Smithsonian Institution, National Museum of Natural History, Washington, D.C.
Methods
Model system
In addition to constituting a chronofauna, the Late Cretaceous ornithischian assemblage of North America merits our focus for several reasons. First, it is one in which ontogenetic niche partitioning is thought to have characterised the represented megaherbivorous species (e.g. Codron et al. Citation2012b; Erickson and Zelenitsky Citation2014). Herbivorous or omnivorous theropods are also known from this setting (e.g. caenagnathids, ornithomimids, therizinosauroids, and possibly troodontids), but they tend to exhibit a normal body size-species richness distribution, and so are not strongly implicated in the size-mediated competition hypothesis (Codron et al. Citation2012b). Therefore, we do not consider them further here. Second, the Late Cretaceous ornithischian assemblage of North America is well-sampled and has produced abundant material from different size classes (although, as noted in the Introduction, the smaller sizes are comparatively under-sampled). Third, the palaeoecology of this assemblage, while still incompletely known, is comparatively better understood than most others (Lehman Citation2001; Hartman et al. Citation2002; Currie and Koppelhus Citation2005; Titus and Loewen Citation2013; Fowler Citation2017; Mallon Citation2019). Fourth, sedimentary deposits from throughout the Upper Cretaceous succession show strong evidence for wet-dry seasonality (Johnston Citation1979; Eberth et al. Citation2013; Arens and Allen Citation2014); competition and niche partitioning tend to be most pronounced in such settings, particularly during the dry season (du Toit Citation1990; Kleynhans et al. Citation2011; Frank et al. Citation2021).
The Late Cretaceous ornithischian assemblage of North America () consists of small (15–400 kg) herbivores (leptoceratopsids, pachycephalosaurids, and thescelosaurids) and megaherbivores (ankylosaurs, ceratopsids and hadrosaurids). The sauropod Alamosaurus also fits the latter category, but it does not form part of this study because it is present only in the southwestern USA (Lehman Citation2001), and is not known from cranial material. Although our model system is time-averaged over a period of ~10 Myr (~76–66 Ma), and so does not constitute a ‘true’ ecological community, all the above clades are present in deposits throughout the assemblage, and so likely co-occurred (e.g. Pearson et al. Citation2002; Larson et al. Citation2010; Cullen et al. Citation2016). Therefore, so far as we can establish, time averaging does not appreciably obscure the ecological relationships of these taxa. Even so, our analyses are time-constrained where appropriate (see ‘Browse profiles’ below).
Figure 1. Time-calibrated phylogeny of major clades considered in this study. Original image credits for silhouettes: C Dylke (Centrosaurinae); MR Villarreal (modified by TM Keesey) (Chasmosaurinae); JM Wood (Lambeosaurinae); modified from Mallon et al. (Citation2013) and JM Wood (Hadrosaurinae); Boyd et al. (Citation2009) (Thescelosauridae); T Ford (Leptoceratopsidae); SA Vega (Pachycephalosauridae); B McFeeters and TM Keesey (Ankylosauridae); S Hartman (Nodosauridae).
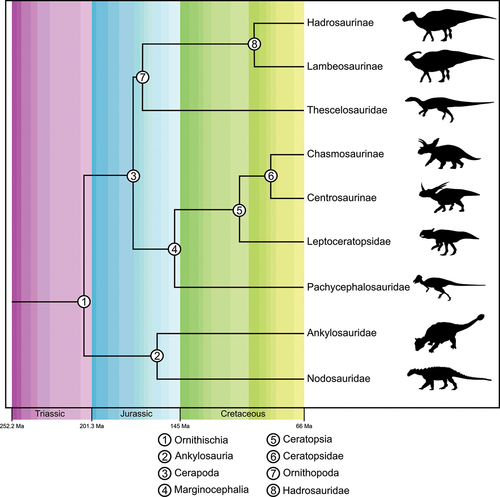
Ecomorphology
We examined ecomorphospace occupation for the assemblage to approximate niche relationships, which is very common in palaeontology (Van Valkenburgh Citation1994; Button et al. Citation2014; Stroik Citation2014), imperfect though the form-function relationship is (Lauder Citation1995). We considered 12 linear skull variables used by Mallon and Anderson (Citation2013), which are known to correlate with aspects of plant quality, growth habit, and mechanical properties in extant vertebrates, including ungulates and reptiles (e.g. Janis Citation1990; Spencer Citation1995; Stayton Citation2005) (details provided in Mallon and Anderson Citation2013 and Supplementary File S1). A thirteenth variable, snout shape index (SSI), approximates feeding selectivity (Dompierre and Churcher Citation1996), and was calculated following Whitlock (Citation2011)for ‘premaxillary shape index’ (the rostral bone, rather than the premaxilla, constitutes the cropping mechanism for ceratopsians). Ankylosaurs were not included in our ecomorphological analyses because skulls belonging to young individuals are unknown in North America. Owing to time and logistical constraints, measured skulls were limited to those we could sample from the Belly River and Edmonton groups, and to the Blackleaf, Judith River, Kaiparowits, Two Medicine, Wapiti, St. Mary River, Willow Creek, Lance, and Hell Creek formations. Most of these strata occur within the northern half of the Western Interior Basin (Alberta, Montana, and North Dakota), and are therefore biased in this sense. Nevertheless, their fossil assemblages are generally representative of the more southerly Upper Cretaceous formations (with the aforementioned exception of the Maastrichtian Alamosaurus), many of which are more poorly sampled than the best-sampled northern strata (e.g. Fruitland, Kirtland, Ojo Alamo, Aguja, Javelina, and North Horn formations). Owing to logistical constraints, our sampling of the available small skull material was not exhaustive, but nearly so.
We used ordination procedures to place our taxa within an ecomorphospace. Prior to ordination, our 13 skull measurements were log-transformed to linearise relationships among them. Missing values were imputed using the Bayesian principal component method (BPCA), which performs well on fossil data with up to 50% missing values (Brown et al. Citation2012b); specimens having >50% missing data were excluded from our study. We performed two types of ordination analyses: (1) one that included body size variation, and (2) one that corrected for it. The size-included analysis was performed using phylogenetic principal components analysis (pPCA) assuming a Brownian motion model to account for stochastic evolutionary drift in our data. Our phylogenetic supertree, also used in the size-corrected analysis, combined the recent phylogenies of Benson et al. (Citation2018), Cruzado-Caballero et al. (Citation2019), and Norman (Citation2020) (details in Supplementary File S1). The size-corrected analysis was performed by regressing the BPCA-imputed variables against basal skull length (a proxy for body size) using phylogenetic generalised least squares (pGLS) regression. As before, we specified a Brownian motion model of evolution. The pGLS residuals were finally subjected to principal components analysis (PCA) of the correlation matrix. This procedure was used in lieu of pPCA, which produces correlated axes that are not amendable to statistical testing (Polly et al. Citation2013). We limited our focus to shape differences at small body sizes by excluding all skulls larger than the largest of our small ornithischians (Pachycephalosaurus wyomingensis NSM PV 20423; basal skull length = 375 mm). These and other analyses were conducted in R v. 4.0.2 (R Core Team Citation2019). The raw data, code, and package citations are given in the Supplementary Code files S1–S2, Supplementary Table S1, and Supplementary Files S1 and S2.
We used non-parametric multivariate analysis of variance (NPMANOVA) to compare taxonomic groups for the size-adjusted analysis. Because sample size varied widely between groups, we rarefied our tests (over 1,000 iterations) to the smallest available sample (n = 5 for each clade). The 1,000 p-values from the omnibus test were combined into a single p-value using the harmonic mean p-value method, which accounts for non-independence of the p-values being combined. This method is capable of handling large datasets and controls for familywise error rate (Wilson Citation2019). When returned p-values were statistically significant (α = 0.05), follow-up pairwise comparisons were conducted on the rarified datasets over 1,000 iterations. The resulting un-adjusted p-values for each pairwise test were also combined using the harmonic mean p-value method. These p-values were then adjusted for multiple pairwise comparisons using Holm-correction (Holm Citation1979).
If the Late Cretaceous small ornithischian assemblage was structured by resource competition from immature megaherbivores, those assemblages immediately ancestral should contain a diversity of small species exhibiting greater overlap in ecomorphospace. Unfortunately, such assemblages are as-yet poorly known. Nevertheless, as a preliminary test of the prediction, we added several basal outgroup taxa to our ecomorphospace. These include the basal ceratopsians Archaeoceratops oshimai, Auroraceratops rugosus, and Yinlong downsi, and a grade of basal neornithischians and ornithopods, including Agilisaurus louderbacki, Dryosaurus altus, Hypsilophodon foxii, and Jeholosaurus shangyuanensis. To be clear, these basal taxa were not sympatric but are simply used here to approximate ancestral morphologies.
Browse profiles
We constructed browse profiles for the herbivorous groups considered above by modifying the methodology of Coe et al. (Citation1987). In this analysis, we specified the upper Campanian assemblage of Dinosaur Provincial Park, Alberta as our model because (1) it has been thoroughly collected for more than 100 years, (2) this narrowed focus minimises the confounding effects of time-averaging (ca. 1.5 million years), and (3) the herbivores are otherwise typical of many other herbivore assemblages from the Upper Cretaceous of North America (Mallon Citation2019).
Representative adult body masses for the different dinosaur groups were estimated using the recommended limb bone scaling equations of Campione and Evans (Citation2012) for quadrupeds and Campione et al. (Citation2014) for bipeds, implemented in the R package MASSTIMATE v 2.0–1 (Campione Citation2020). The body masses of immature ornithischians were estimated by allometrically scaling down the linear dimensions of the adults (a reasonable approach, given the crudeness of our calculations), and using developmental mass extrapolation (Erickson and Tumanova Citation2000), again implemented in MASSTIMATE.
Raw count data (minimum number of individuals, or MNI) were taken from the Dinosaur Provincial Park quarry occurrence database on the supplemental CD-ROM provided by Currie and Koppelhus (Citation2005). In addition to individual skeletons, the database also includes monodominant bonebed and uncollected occurrences. Monodominant dinosaur bonebeds (particularly those pertaining to some of the ceratopsids considered here) are frequently thought to have formed under unusual circumstances (e.g. sudden flooding events: Eberth Citation2015), and so are not reflective of the normal attrition of the herbivore standing crop. For this reason, each bonebed was counted as a single occurrence, in keeping with prior work (e.g. Béland and Russell Citation1978). To the best of our ability, we counted only adult occurrences, and were therefore careful to exclude specimens that were recorded as being ‘small’ or ‘juvenile’, as well as those attributed to genera now recognised as immature growth stages of other species (e.g. ‘Eoceratops’, Godfrey and Holmes Citation1995; ‘Tetragonosaurus’, Evans et al. Citation2005). Small ornithischian occurrences were nearly absent from the database of Currie and Koppelhus (Citation2005) because there is a taphonomic bias against their preservation (Brown et al. Citation2013b), and because either their associated quarries were long forgotten or their collection did not require substantial digging that would produce a quarry. We therefore sourced more comprehensive count data from additional literature (leptoceratopsids: Ryan et al. Citation2012; pachycephalosaurids: Evans et al. Citation2013; thescelosaurids: Brown et al. Citation2013a). Pachycephalosauridae is overrepresented among the small ornithischians, due to the taphonomic resistance of their distinctive frontoparietal domes (Evans et al. Citation2013; Mallon and Evans Citation2014). As such, the leptoceratopsid and thescelosaurid counts were inflated using a correction factor that incorporates the ratio of pachycephalosaur specimens known from isolated domes to those also preserving postcranial material. The above steps are conservative and would have the effect of inflating the ratio of small herbivores to megaherbivores, reflecting the condition of most extant herbivore communities.
We combined the adult counts with published survivorship estimates to infer the population proportions of the immature growth stages. Such data are not available for either ankylosaurs or the small ornithischian groups; however, for the sake of argument, we imputed the survivorship data for Psittacosaurus lujiatunensis (Erickson et al. Citation2009) to the latter. Most studied dinosaurs appear to have followed a similar type B1 survivorship curve (Erickson Citation2014), so we do not expect this to be an egregious source of error, although we recognise the potential pitfalls of this assumption.
We estimated proportional biomass by multiplying the adjusted population proportions by the average body mass estimates for each growth stage/taxon, and normalising to 100%. Proportional biomass was then multiplied by the individual nutritional requirements of each growth stage/taxon. We estimated the latter according to the daily mass-specific intake rates of carnivorous reptiles, following Coe et al. (Citation1987), to facilitate comparison with that study. However, because metabolic requirements can be approximated in other ways, and because of uncertainty surrounding the issue of whether dinosaurs were bradymetabolic or tachymetabolic (summarised in Chinsamy and Hillenius Citation2004; Padian and Horner Citation2004), we chose to further bracket our estimates by assuming the size-specific field metabolic rates (FMRs) of mammals and varanids, given in Farlow et al. (Citation2010). We likewise assessed the effect of assuming differential metabolisms by assigning the FMR of varanids to the megaherbivorous dinosaur species (including the early growth stages) and assigning the FMR of mammals to the small ornithischians.
We then calculated the proportional intake for each growth stage/taxon, and divided over their respective feeding heights in 0.5 m increments. Feeding heights were estimated following the approach of Mallon et al. (Citation2013), whereby quadrupedal feeding heights are approximated by forelimb length, and bipedal feeding heights are calculated trigonometrically. All taxa were assumed to have been capable of bipedal feeding, except the ankylosaurs and ceratopsids. Our data and annotated calculations are provided in Supplementary Tables S2–S5.
Results
Ecomorphospaces
The first two pPC axes account for approximately 84% of the total variation within the size-included ecomorphospace ()). pPC1 primarily reflects size variation, increasing positively along the axis. The leptoceratopsids, pachycephalosaurids, and thescelosaurids substantially overlap the immature megaherbivores along pPC2, but separate from one another along the same axis, on which snout shape index loads most heavily (increasing in the negative direction). There is some taxonomic separation along the subsequent pPC axes, but each of these accounts for an insignificant amount (<5%) of the total variation (Figure S2(a,b) of Supplementary File S1).
Figure 2. Results of principal component analyses. (a) Plot of pPC1 vs. pPC2 for size-included dataset. (b) Plot of PC1 vs. PC2, (c) PC3 vs. PC4 for the size-adjusted dataset. Corresponding loadings plots are available in Supplementary Figure S3. Some of the basal ornithischian text points in b and c have been shifted slightly to improve readability. Taxonomic abbreviations: Ag, Agilisaurus louderbacki; Ar, Archaeoceratops oshimai; Au, Auroraceratops rugosus; Dr, Dryosaurus altus; Hy, Hypsilophodon foxii; J, Jeholosaurus shangyuanensis; Y, Yinlong downsi.
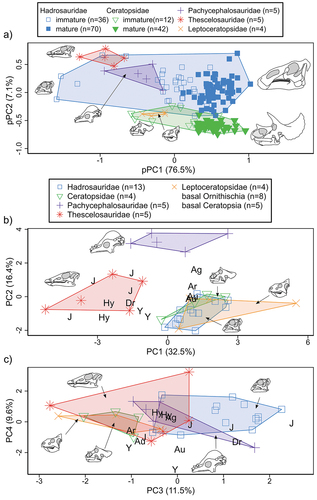
The size-adjusted PCA ( and S2(c) of Supplementary File S1) more precisely captures shape variation at small body size. The variation is spread out more evenly along the first several PC axes, but the ornithischian groups separate best along the first three (total variation PC1–3 = 60.4%). Thescelosaurids show total separation from leptoceratopsids and megaherbivores along PC1, with pachycephalosaurids plotting in between. There is still some size influence on PC1, but shape changes along the axis primarily correspond to taller skulls and dentaries, and posteriorly extended tooth rows – all features typical of leptoceratopsids and young hadrosaurids and ceratopsids (loadings provided in Figure S3 of Supplementary File 1). Pachycephalosaurids clearly separate from all other taxa along PC2, reflective of their relatively reduced diastemae, less depressed snouts, and longer tooth rows. Hadrosaurids separate from ceratopsids and leptoceratopsids primarily along PC3, which most strongly reflects the wider and squarer beak shapes of the first taxon. The leptoceratopsids and immature ceratopsids are effectively indistinguishable in morphospace.
Omnibus tests for our rarified NPMANOVA indicate highly significant differences (harmonic mean combined p-value = 0.001) between taxonomic groups. However, our pair-wise comparisons lack statistical power to detect any differences between taxa. Because leptoceratopsids overlap considerably with immature ceratopsids, we excluded them from a follow-up analysis to increase power by reducing the number of pair-wise comparisons, but significance was still not achieved (). However, based on our size-adjusted PCA, the total isolation of the thescelosaurids and pachycephalosaurids in ecomorphospace suggests that they are probably ecomorphologically distinct from the other groups, and possibly hadrosaurids as well.
Table 1. Post-hoc pairwise values for NPMANOVA tests conducted on rarified size-corrected small ornithischian and juvenile megaherbivore datasets (combined over 1000 iterations).
The more basal taxa (neornithischians, ornithopods, and ceratopsians) generally plot with and between the Late Cretaceous forms in ecomorphospace, with a tendency to cluster centrally on the plot. They overlap most substantially with the thescelosaurids.
Browse profiles
No matter the metabolic proxy used, the herbivore browse profile of the Dinosaur Provincial Park assemblage is dominated by adult hadrosaurids ( and S4 of Supplementary File S1), which make up 66–71% of the total browsing pressure, and which could feed well above the reach of the other herbivores in the ecosystem (up to 4.5 m above ground). By comparison, the relative browsing pressure of the hadrosaurids was estimated to be just 48% by Coe et al. (Citation1987), who were working with a much smaller dataset. Adult ceratopsids make up the next most important group in the assemblage (~15% total browsing pressure), and were limited to low level feeding (up to 1 m), as were most of the other herbivores. The remaining groups make up no more than 19% of the overall browsing pressure combined. Most important among these are the immature hadrosaurids (6–8%) and ceratopsids (5–7%). The combined contribution of the small leptoceratopsids, pachycephalosaurids, and thescelosaurids to overall browsing pressure is negligible (just 0.02–0.07%), even though these animals appear to have been abundant on the palaeo-landscape (~84% of the total herbivore population). This combined value can be increased to 0.32% of the total browsing pressure if it is assumed that these small ornithischians alone possessed metabolic rates on par with those of like-sized mammals, but the difference is trivial. Even assuming that the small ornithischians are under-sampled in our dataset by 100-fold, their combined browsing pressure still does not exceed 10% (under the mixed metabolism model), which is 5% less than the immature hadrosaurids and ceratopsids.
Figure 3. Browse profiles for the Dinosaur Provincial Park ornithischian assemblage. (a) Browse profile assuming reptilian intake requirements given in Coe et al. (Citation1987); (b) close-up of small herbivores given in (a); (c) browse profile assuming mixed field metabolic rates for megaherbivores and small ornithischians; (d) close-up of small herbivores given in (c). Image credits after . Associated data and calculations are available in Supplementary Tables S2 and S5.
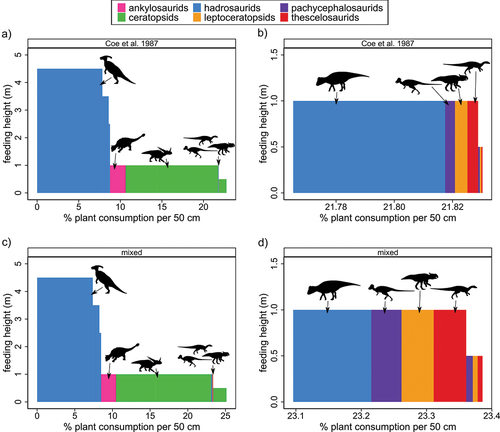
Discussion
Competition and community structure
If resource competition at small body sizes had a strong effect in structuring Late Cretaceous ornithischian communities, we predict that, over the 10+ Myr span of the entire assemblage, the coexisting small-bodied ornithischian lineages (and immature megaherbivores) would have evolved to occupy distinct fundamental niches, approximated here using ecomorphospace. Ideally, we might address this question by examining ecomorphospace occupation at subsequent time intervals (e.g. Van Valkenburgh Citation1994; Foffa et al. Citation2018; Mallon Citation2019) for specific depositional settings, but the data are presently too sparse (especially at the smallest body sizes) to allow such an approach. Instead, we take a coarser, necessarily less sophisticated tack by looking at the distribution of the assemblage as a whole and note that: (1) most small herbivore forms exhibit good separation along at least one axis of ecomorphospace, and (2) the approximated ancestral forms tend to occupy a similar area of ecomorphospace to each other. These results are broadly consistent with what we would expect, given the evolutionary effects of size-mediated competition. Interestingly, the wide overlap of the thescelosaurids with the more basal outgroup taxa included here possibly suggests that the former retained the plesiomorphic niche requirements, and that the hadrosaurids, leptoceratopsids, and ceratopsids evolved away from this condition. However, all the foregoing considerations come with several important caveats.
First, as mentioned already, our findings are not statistically robust owing to small sample size. However, we expect that the general spatial relationships we recovered will maintain with new fossil discoveries. The broad lack of intrafamilial sympatry among small, Late Cretaceous species might be yet further evidence to this end (cf. Mallon Citation2019 for megaherbivores), but species richness is still poorly sampled at these small body sizes (Brown et al. Citation2012a, Citation2013b, Citation2021; Evans et al. Citation2013) so that their stratigraphic distributions are still not entirely understood. We do not think that increased sampling is likely to yield particularly novel or surprising ecomorphotypes, given microfossil data from throughout the stratigraphic column attest to the consistent presence of the same families examined here (e.g. Pearson et al. Citation2002; Larson et al. Citation2010; Cullen et al. Citation2016).
Second, although the centralised clustering of our ancestral proxies in ecomorphospace is consistent with the size-mediated competition hypothesis, it may likewise reflect phylogenetic effects; on a Brownian motion model, ancestral taxa should plot centrally in morphospace, wholly apart from their niche relationships, simply because they have not experienced the same divergence times as their descendant species (Pagel Citation2002; Symonds and Blomberg Citation2014). An assessment of phylogenetic community structure is beyond the scope of this study, but we might predict a higher phylogenetic evenness (lower relatedness among co-occurring species than expected by chance) for these Late Cretaceous herbivore assemblages compared to those immediately ancestral, a pattern thought to be driven by competitive exclusion (Fraser et al. Citation2015). Unfortunately, earlier Late Cretaceous (Santonian to early Campanian) dinosaur assemblages in North America are poorly known, and so cannot be used for comparison.
Third, leptoceratopsids and immature ceratopsids provide a notable exception to the pattern we document here. These two groups coexisted in North America for at least 20 million years (Ryan et al. Citation2012), and yet their near-total overlap in ecomorphospace (assuming our 13 linear variables adequately reflect niche relationships) suggests that their ecological interactions were not strongly competitive in nature. Therefore, any dietary resources shared by the two groups may not have been limiting in the same way. Leptoceratopsids and ceratopsids do exhibit clear differences in dental occlusal morphology (Ostrom Citation1966) but the unique stepped occlusal surface of the leptoceratopsids is an artefact of differential wear via incomplete shear of the upper teeth against their lower counterparts (Varriale Citation2016), and is not obviously related to diet.
Our reconstructed browse profiles for Dinosaur Provincial Park, which are likely more broadly applicable in space and time (Mallon Citation2019), identify hadrosaurids as the dominant herbivores on the landscape, consuming a vast majority of the available browse (see Mallon et al. Citation2013 for a discussion of available vegetation at different feeding heights). The energetic demands of the large adults were especially high, and if these animals browsed in the herbaceous layer (which included various ferns, cycads, lycopods, angiosperm herbs, and gymnosperm saplings) with any regularity, they likely would have greatly reduced the quantity of food available to the smaller herbivores. Even among these, the browsing pressure from just the yearling hadrosaurids feeding below 1 m is up to five times that of the combined browsing pressure of the small ornithischians (depending on the assumed metabolic rates). These numbers accord with the size-mediated competition hypothesis of Codron et al. (Citation2013), which posits juvenile megaherbivores as important competitors for small ornithischians. The additional and substantial low-browsing pressure of the ceratopsids and ankylosaurs probably ensured that the forage still available to would-be small competitors was unlikely to have been plentiful.
Considering the foregoing discussion, the Late Cretaceous herbivore assemblage of North America is reminiscent of today’s mammal-dominated ecosystems in East and Southern Africa in some respects, but not others. Like the hadrosaurids, the megaherbivorous elephants are comparatively low in number (just 1% of the population in Amboseli National Park; Coe et al. Citation1987), and yet they make up the majority (or plurality – 33% in Amboseli) of the overall browsing pressure (farmed cattle excluded). The impacts of elephant foraging can so influence the landscape as to convert woodland environments to grasslands (Laws Citation1970; Guldemond and van Aarde Citation2008), and significantly impede the numbers of herbivorous ungulates (particularly browsers and mixed feeders), while at the same time facilitating the coexistence of the grazing ungulates (Fritz et al. Citation2002). Perhaps, hadrosaurids had similar effects on their ecosystems. However, whereas other megaherbivore groups also contributed substantially to overall browsing pressure during the Late Cretaceous, the contributions of megaherbivorous hippopotamus, rhinoceros, and giraffe in modern Amboseli are comparatively insignificant (<2%) (Coe et al. Citation1987). Instead, it is the smaller but more populous zebra and wildebeest that sustain much of the remaining browsing pressure. Populations of small ungulates not constrained by resource competition are very frequently limited by top-down processes, such as predation (Sinclair et al. Citation2003), and the same was probably true of those small herbivorous dinosaur groups that similarly escaped resource competition (Farlow and Holtz Citation2002; Hone and Rauhut Citation2010).
Dietary considerations
It is generally accepted that small ornithischians specialised on higher quality foodstuffs than their megaherbivorous counterparts, given their smaller and more selective beaks, weaker dentition, lesser jaw adductor musculature, and higher mass-specific energy requirements (Weishampel Citation1984; Coe et al. Citation1987; Farlow Citation1987). Our results are largely consistent with this interpretation. However, even within the small herbivore guild, dinosaurs probably varied in diet; small herbivores tend to divide heterogeneous environments more finely than large ones (Van Wieren and Van Langevelde Citation2008).
The types of foods these groups selected for are more difficult to establish, which explains why few have explored this line of research. Almost certainly, their diets overlapped to some degree. Pachycephalosaurids are thought to have eaten leaves, stems, seeds, fruits, and perhaps insects (Maryańska and Osmólska Citation1974; Maryańska et al. Citation2004). They are distinguished here by their relatively depressed snouts below the occlusal plane, shorter diastemae and correspondingly longer tooth rows. The first two features conceivably were an upshot of the strong facial sloping produced by the frontoparietal dome, but a relatively elongate tooth row would have helped in the slicing of tough foods requiring continuous crack propagation (like leaves and insect cuticle) (Lucas Citation2004). Their simple, phylliform teeth and rotund bodies imply that most of the processing occurred via gut fermentation (Bakker Citation1986; Button and Zanno Citation2020).
On average, thescelosaurids possessed the smallest skulls of any of the forms examined here. They were undoubtedly highly selective as a result, and their low skulls and dentaries betray a propensity for relatively soft, pulpy foods requiring little oral processing. Their long, low snouts may have been used to probe shrubs for the most delicate fruits and shoots. The recent finding of a basal ?stegosaurian (Han et al. Citation2017), possessing a cranial ecomorphology not unlike that of thescelosaurids, was shown to have stomach contents composed almost entirely of cycad seeds (Salgado et al. Citation2017).
The similar ecomorphologies of the leptoceratopsids and young ceratopsids give reason to suspect these animals broadly overlapped in diet. Both taxa have comparatively deep skulls and dentaries, which would have withstood the forces generated by masticating resistant foodstuffs (Henderson Citation2010; Mallon and Anderson Citation2014a; Longrich Citation2016). Their narrow and pointed beaks indicate that they were selective feeders, which are typically not associated with consumers of very fibrous plants (Mallon and Anderson Citation2014a). Ceratopsids notably have a larger shearing surface of the dental battery (Ostrom Citation1966; Mallon and Anderson Citation2014b; Varriale Citation2016), and so might have incorporated a higher proportion of fibrous plants in their diets.
The ecomorphology of immature hadrosaurid skulls differs most appreciably from that of leptoceratopsids and young ceratopsids in the possession of a broader, squarer beak. They were therefore probably less selective in their diets, and yet shared a tooth battery with the ceratopsids that could rend the toughest plant parts (Lull and Wright Citation1942; Ostrom Citation1961; Mallon and Anderson Citation2014b). Although structured differently (Erickson et al. Citation2012, Citation2015), the dental batteries of subadult hadrosaurids in particular resembled those of ceratopsids in having a strong shearing component (Erickson and Zelenitsky Citation2014).
Conclusions
There are good reasons, stemming from considerations of life history and cranial ontogenetic allometry, to suppose that at least some dinosaurs experienced ONSs. If modern ecological studies are any indication, such niche shifts may have had reverberating effects on the interactions within, and the structuring of, the wider community. Some researchers have suggested that size-mediated resource competition pitted small dinosaur species against the immature forms of larger species, the latter having had a competitive advantage due to their ability to eventually ‘outgrow’ the competition as they changed niches with age.
We make no strong claims to being able to provide a definitive test of the size-mediated competition hypothesis; however, we do provide some observations from the Late Cretaceous ornithischian assemblage of North America that appear consistent with it. First, with the notable exception of the leptoceratopsids and young ceratopsids, the immature megaherbivores and small ornithischian lineages exhibit good separation along at least one axis of ecomorphospace, as might be expected of a chronofauna shaped in its early stages by ecological competition (although further work is required to demonstrate that these ecomorphological differences were adaptive). Second, at small body sizes, immature megaherbivores – particularly hadrosaurids – appear to have consumed most of the available low-level browse, which would have made them formidable competitors, capable of controlling much of the available resource base.
Continued sampling at finer temporal scales, and using other ecological proxies (e.g. tooth wear, isotopes) will help to refine (or refute) our arguments. There is an obvious need to better understand the assemblages immediately ancestral to those examined here, dating to the Santonian and early Campanian. This time corresponds to a period when several of the implicated herbivore groups arose, and study of their assemblages will eventually clarify the possible interactions among the groups (negating the need for the ancestral proxies used here). Consideration of the influence that carnivorous theropod distributions (cf. Holtz Citation2021) had on those of herbivorous dinosaurs is another interesting avenue for future research. We also stress the need for ‘supply-side’ modelling of available browse during the Late Cretaceous to compliment our ‘demand-side’ models above. Such an undertaking is itself replete with assumptions and beyond the scope of this paper but would go a long way towards clarifying the availability of plant resources both horizontally and vertically.
Supplementary_Code_S2.R
Download R Objects File (15.1 KB)Supplementary_Code_S1.R
Download R Objects File (57.5 KB)Supplementary_Tables.xlsx
Download MS Excel (95.3 KB)Supplementary_File_S2.tre
Download (1.2 KB)Supplementary_File_S1.docx
Download MS Word (1.5 MB)Acknowledgments
Constructive criticism of early drafts of this paper was provided by G. Blouin-Demers, C. Brown, D. Evans, N. Longrich, M. Ryan, A. Simons, F. Therrien and four prior anonymous reviewers. Helpful conversations and methodological support were offered by B. Arnaout, D. Fraser, and N. Campione. Thanks to curators and their assistants at AMNH, CMN, GPDM, MOR, ROM, RSM, TMP, USNM, UALVP, and UC, and to A. Knapp and L. Witmer for access to materials and models. Thanks also to J. Farlow and J. Foster for their constructive reviews of the most recent manuscript, and to G. Dyke for his editorial assistance.
Disclosure statement
No potential conflict of interest was reported by the authors.
Data availability statement
The authors confirm that the data supporting the findings of this study are available within the article and its supplementary materials.
Supplementary material
Supplemental data for this article can be accessed here
Additional information
Funding
References
- Arens NC, and Allen SE. 2014. A florule from the base of the Hell Creek Formation in the type area of eastern Montana: implications for vegetation and climate. In: Wilson GR, Clemens WA, Horner JR, and Hartman KH , editors. Through the end of the Cretaceous in the type locality of the Hell Creek Formation in Montana and adjacent areas Boulder (CO): The Geological Society of America. p: 173–207.
- Bakker RT. 1986. The dinosaur heresies: new theories unlocking the mystery of the dinosaurs and their extinction. New York (NY): William Morrow and Co.
- Béland P, Russell DA. 1978. Paleoecology of Dinosaur Provincial Park, Alberta, interpreted from the distribution of articulated vertebrate remains. Can J Earth Sci. 15:1012–1024. doi:10.1139/e78-109.
- Benson RBJ. 2018. Dinosaur macroevolution and macroecology. Annu Rev Ecol Evol Syst. 49:379–408. doi:10.1146/annurev-ecolsys-110617-062231.
- Benson RBJ, Hunt G, Carrano MT, Campione N 2018 Aug 30. Data from: cope’s rule and the adaptive landscape of dinosaur body size evolution [dataset]. Dryad Digital Repository. [accessed 2021 Oct 2]. doi:10.1111/pala.12329.
- Benton MJ. 1996. Testing the roles of competition and expansion in tetrapod evolution. Proc R Soc B. 263(1370):641–646. doi:10.1098/rspb.1996.0096.
- Boyd CA, Brown CM, Scheetz RD, Clarke JA. 2009. Taxonomic revision of the basal neornithischian taxa Thescelosaurus and Bugenasaura. J Vertebr Paleontol. 29(3):758–770. doi:10.1671/039.029.0328.
- Brinkman DB, Russell AP, Eberth DA, Peng J. 2004. Vertebrate palaeocommunities of the lower Judith River Group (Campanian) of southeastern Alberta, Canada, as interpreted from vertebrate microfossil assemblages. Palaeogeogr Palaeoclimatol Palaeoecol. 213(3–4):295–313. doi:10.1016/j.palaeo.2004.07.016.
- Brown CM, Arbour JH, Jackson DA. 2012b. Testing the effect of missing data estimation and distribution in morphometric multivariate data analyses. Syst Biol. 61(6):941–954. doi:10.1093/sysbio/sys047.
- Brown CM, Campione NE, Giacomini HC, O’Brien LJ, Vavrek MJ, Evans DC. 2012a. Ecological modelling, size distributions and taphonomic size bias in dinosaur faunas: a comment on Codron et al. (2012). Biol Lett. 9(1):20120582. doi:10.1098/rsbl.2012.0582.
- Brown CM, Campione NE, Wilson Mantilla GP and Evans DC. (2021). Size-driven preservational and macroecological biases in the latest Maastrichtian terrestrial vertebrate assemblages of North America. Paleobiology 1–29. doi:10.1017/pab.2021.35
- Brown CM, Evans DC, Campione NE, O’Brien LJ, Eberth DA. 2013b. Evidence for taphonomic size bias in the Dinosaur Park Formation (Campanian, Alberta), a model Mesozoic terrestrial alluvial‐paralic system. Palaeogeogr Palaeoclimatol Palaeoecol. 372:108–122. doi:10.1016/j.palaeo.2012.06.027.
- Brown CM, Evans DC, Ryan MJ, Russell AP. 2013a. New data on the diversity and abundance of small-bodied ornithopods (Dinosauria, Ornithischia) from the Belly River Group (Campanian) of Alberta. J Vertebr Paleontol. 33(3):495–520. doi:10.1080/02724634.2013.746229.
- Brown JH. 1995. Macroecology. Chicago (IL): University of Chicago Press.
- Button DJ, Rayfield EJ, Barrett PM. 2014. Cranial biomechanics underpins high sauropod diversity in resource-poor environments. Proc R Soc B. 281(1795):20142114. doi:10.1098/rspb.2014.2114.
- Button DJ, Zanno LE. 2020. Repeated evolution of divergent modes of herbivory in non-avian dinosaurs. Curr Biol. 30(1):158–168. doi:10.1016/j.cub.2019.10.050.
- Campione NE. 2020. MASSTIMATE: body mass estimation equations for vertebrates [R package]. Version 1.4. [accessed 2020 May 1]. https://CRAN.R-project.org/package=MASSTIMATE
- Campione NE, Evans DC. 2012. A universal scaling relationship between body mass and proximal limb bone dimensions in quadrupedal terrestrial tetrapods. BMC Biol. 10(60). doi:10.1186/1741-7007-10-60.
- Campione NE, Evans DC, Brown CM, Carrano MT. 2014. Body mass estimation in non‐avian bipeds using a theoretical conversion to quadruped stylopodial proportions. Methods Ecol Evol. 5(9):913–923. doi:10.1111/2041-210X.12226.
- Carravieri A, Weimerskirch H, Bustamante P, Cherel Y. 2017. Progressive ontogenetic niche shift over the prolonged immaturity period of wandering albatrosses. R Soc Open Sci. 4(10):171039. doi:10.1098/rsos.171039.
- Chinsamy A, Hillenius WJ. 2004. Physiology of nonavian dinosaurs. In: Weishampel DB, Dodson P, Osmólska H, editors. The Dinosauria. 2nd ed. Berkeley and Los Angeles: University of California Press; p. 643–659.
- Claessen D, Dieckmann U. 2001. Ontogenetic niche shifts and evolutionary branching in size-structured populations. Evol Ecol Res. 4(2):189–217.
- Codron D, Carbone C, Clauss M. 2013. Ecological interactions in dinosaur communities: influences of small offspring and complex ontogenetic life histories. PLoS ONE. 8(10):e77110. doi:10.1371/journal.pone.0077110.
- Codron D, Carbone C, Müller DW, Clauss M. 2012a. Ontogenetic niche shifts in dinosaurs influenced size, diversity and extinction in terrestrial vertebrates. Biol Lett. 8(4):620–623. doi:10.1098/rsbl.2012.0240.
- Codron D, Carbone C, Müller DW, Clauss M. 2012b. Ecological modelling, size distributions and taphonomic size bias in dinosaur faunas: reply to Brown et al. Biol Lett. 9(1):20120922. doi:10.1098/rsbl.2012.0922.
- Coe MJ, Dilcher DL, Farlow JO, Jarzen DM, Russell DA. 1987. Dinosaurs and land plants. In: Friis EM, Chaloner WG, Crane PR, editors. The origins of angiosperms and their biological consequences. Cambridge (UK): Cambridge University Press; p. 225–258.
- Cruzado-Caballero P, Gasca JM, Filippi LS, Cerda IA, Garrido AC. 2019. A new ornithopod dinosaur from the Santonian of Northern Patagonia (Rincon de los Sauces, Argentina). Cretac Res. 98:211–229. doi:10.1016/j.cretres.2019.02.014.
- Cullen TM, Fanti F, Capobianco C, Ryan MJ, Evans DC. 2016. A vertebrate microsite from a marine-terrestrial transition in the foremost formation (Campanian) of Alberta, Canada, and the use of faunal assemblage data as a paleoenvironmental indicator. Palaeogeogr Palaeoclimatol Palaeoecol. 444:101–114. doi:10.1016/j.palaeo.2015.12.015.
- Currie PJ, Koppelhus EB. 2005. Dinosaur Provincial Park: a spectacular ancient ecosystem revealed. Bloomington (IN): Indiana University Press.
- Dodson P. 1975. Functional and ecological significance of relative growth in Alligator. J Zool. 175(3):315–355. doi:10.1111/j.1469-7998.1975.tb01405.x.
- Dompierre H, Churcher CS. 1996. Premaxillary shape as an indicator of the diet of seven extinct late cenozoic new world camels. J Vertebr Paleontol. 16(1):141–148. doi:10.1080/02724634.1996.10011292.
- du Toit JT. 1990. Feeding‐height stratification among African browsing ruminants. Afr J Ecol. 28(1):55–61. doi:10.1111/j.1365-2028.1990.tb01136.x.
- Ebenman B. 1992. Evolution in organisms that change their niches during the life cycle. Am Nat. 139(5):990–1021. doi:10.1086/285370.
- Eberth DA. 2015. Origins of dinosaur bonebeds in the Cretaceous of Alberta, Canada. Can J Earth Sci. 52(8):655–681. doi:10.1139/cjes-2014-0200.
- Eberth DA, Evans DC, Brinkman DB, Therrien F, Tanke DH, Russell LS. 2013. Dinosaur biostratigraphy of the Edmonton group (Upper Cretaceous), Alberta, Canada: evidence for climate influence. Can J Earth Sci. 50(7):701–726. doi:10.1139/cjes-2012-0185.
- Erickson GM. 2014. On dinosaur growth. Annu Rev Earth Planet Sci. 42:675–697. doi:10.1146/annurev-earth-060313-054858.
- Erickson GM, Krick BA, Hamilton M, Bourne GR, Norell MA, Lilleodden E, and Sawyer WG. 2012. Complex dental structure and wear biomechanics in hadrosaurid dinosaurs. Science. 338:98–101 doi:10.1126/science.1224495. .
- Erickson GM, Makovicky PJ, Inouye BD, Zhou C, Gao K. 2009. A life table for Psittacosaurus lujiatunensis: initial insights into ornithischian dinosaur population biology. Anat Rec. 292(9):1514–1521. doi:10.1002/ar.20992.
- Erickson GM, Sidebottom MA, Kay DI, Turner KT, Ip N, Norell MA, Sawyer WG, and Krick BA. 2015. Wear biomechanics in the slicing dentition of the giant horned dinosaur Triceratops. Sci Adv. 1:e1500055 doi:10.1126/sciadv.1500055. .
- Erickson GM, Tumanova TA. 2000. Growth curve of Psittacosaurus mongoliensis Osborn (Ceratopsia: Psittacosauridae) inferred from long bone histology. Zool J Linn Soc. 130(4):551–566. doi:10.1111/j.1096-3642.2000.tb02201.x.
- Erickson GM, Zelenitsky DK. 2014. Osteohistology of Hypacrosaurus stebingeri teeth throughout ontogeny with comments on wear induced form and function. In: Eberth DA, Evans DC, editors. Hadrosaurs. Bloomington: University of Indiana Press; p. 422–432.
- Evans DC, Forster CF, Reisz RR. 2005. The type specimen of Tetragonosaurus erectofrons (Ornithischia: Hadrosauridae) and the identification of juvenile lambeosaurines. In: Currie PJ, Koppelhus E, editors. Dinosaur Provincial Park: a spectacular ancient ecosystem revealed. Bloomington: University of Indiana Press; p. 349–366.
- Evans DC, Schott RK, Larson DW, Brown CM, Ryan MJ. 2013. The oldest North American pachycephalosaurid and the hidden diversity of small-bodied ornithischian dinosaurs. Nat Commun. 4:1–11. doi:10.1038/ncomms2749.
- Farlow JO. 1987. Speculations about the diet and digestive physiology of herbivorous dinosaurs. Paleobiology. 13(1):60–72. doi:10.1017/S0094837300008587.
- Farlow JO, Coroian ID, Foster JR. 2010. Giants on the landscape: modelling the abundance of megaherbivorous dinosaurs of the morrison formation (Late Jurassic, western USA). Hist Biol. 22(4):403–429. doi:10.1080/08912961003787598.
- Farlow JO, Dodson P, Chinsamy A. 1995. Dinosaur biology. Annu Rev Ecol Syst. 26:445–471. doi:10.1146/annurev.es.26.110195.002305.
- Farlow JO, Holtz TR. 2002. The fossil record of predation in dinosaurs. Paleontol Soc Pap. 8:251–266. doi:10.1017/S108933260000111X.
- Foffa D, Young MT, Stubbs TL, Dexter KG, Brusatte SL. 2018. The long-term ecology and evolution of marine reptiles in a Jurassic seaway. Nat Ecol Evol. 2(10):1548–1555. doi:10.1038/s41559-018-0656-6.
- Fowler DW. 2017. Revised geochronology, correlation, and dinosaur stratigraphic ranges of the Santonian-Maastrichtian (Late Cretaceous) formations of the Western Interior of North America. PLoS ONE. 12(11):e0188426. doi:10.1371/journal.pone.0188426.
- Frank SJD, Gopi GV, Sankar K, Hussain SA. 2021. Dry season resource selection among sympatric ungulates in a tropical coastal landscape: implications for conservation and management. Trop Ecol. 62(3):418–426. doi:10.1007/s42965-021-00156-3.
- Fraser D, Gorelick R, Rybczynski N. 2015. Macroevolution and climate change influence phylogenetic community assembly of North American hoofed mammals. Biol J Linn Soc. 114(3):485–494. doi:10.1111/bij.12457.
- Frederickson JA, Engel MH, Cifelli RL. 2020. Ontogenetic dietary shifts in Deinonychus antirrhopus (Theropoda; Dromaeosauridae): insights into the ecology and social behaviors of raptorial dinosaurs through stable isotope analysis. Palaeogeogr Palaeoclimatol Palaeoecol. 552:109780. doi:10.1016/j.palaeo.2020.109780.
- Fritz H, Duncan P, Gordon IJ, Illius AW. 2002. Megaherbivores influence trophic guilds structure in African ungulate communities. Oecologia. 131:620–625. doi:10.1007/s00442-002-0919-3.
- Gaston KJ, Blackburn TM. 2000. Pattern and process in macroecology. Oxford (UK): Blackwell Publishing.
- Godfrey SJ, Holmes R. 1995. Cranial morphology and systematics of Chasmosaurus (Dinosauria: Ceratopsidae) from the Upper Cretaceous of western Canada. J Vertebr Paleontol. 15(4):726–742. doi:10.1080/02724634.1995.10011258.
- Guldemond R, van Aarde R. 2008. A meta‐analysis of the impact of African elephants on savanna vegetation. J Wildl Manage. 72(4):892–899. doi:10.2193/2007-072.
- Han F, Forster CA, Xu X, Clark JM. 2017. Postcranial anatomy of Yinlong downsi (Dinosauria: Ceratopsia) from the Upper Jurassic Shishugou Formation of China and the phylogeny of basal ornithischians. J Syst Palaeontol. 16(14):1159–1187. doi:10.1080/14772019.2017.1369185.
- Hartman JH, Johnson KR, and Nichols DJ . 2002. The Hell Creek Formation and the Cretaceous-Tertiary boundary in the northern Great Plains: An Integrated continental record of the end of the Cretaceous. Boulder (CO): Geological Society of America.
- Henderson DM. 2010. Skull shapes as indicators of niche partitioning by sympatric chasmosaurine and centrosaurine dinosaurs. In: Ryan MJ, Chinnery-Allgeier BJ, Eberth DA, editors. New perspectives on horned dinosaurs: the royal tyrell museum ceratopsian symposium. Bloomington: Indiana University Press; p. 293–307.
- Holm S. 1979. A simple sequentially rejective multiple test procedure. Scand J Stat. 6(2):65–70 Accessed 11 2021. https://www.jstor.org/stable/4615733
- Holtz TR Jr. 2021. Theropod guild structure and the tyrannosaurid niche assimilation hypothesis: implications for predatory dinosaur macroecology and ontogeny in later Late Cretaceous Asiamerica. Can J Earth Sci. 58(9):778–795. doi:10.1139/cjes-2020-0174.
- Hone DWE, Rauhut OWM. 2010. Feeding behaviour and bone utilization by theropod dinosaurs. Lethaia. 43(2):232–244. doi:10.1111/j.1502-3931.2009.00187.x.
- Janis C. 1990. Correlations of cranial and dental variables with dietary preferences in mammals: a comparison of macropodoids and ungulates. Mem Qld Mus. 28(1):349–366.
- Janis CM, Gordon IJ, Illius AW. 1994. Modelling equid/ruminant competition in the fossil record. Hist Biol. 8(1–4):15–29. doi:10.1080/10292389409380469.
- Johnston PA. 1979. Growth rings in dinosaur teeth. Nature. 278(5705):635–636. doi:10.1038/278635a0.
- Kleynhans EJ, Jolles AE, Bos MRE, Olff H. 2011. Resource partitioning along multiple niche dimensions in differently sized African savanna grazers. Oikos. 120(4):591–600. doi:10.1111/j.1600-0706.2010.18712.x.
- Larson DW, Brinkman DB, Bell PR. 2010. Faunal assemblages from the upper Horseshoe Canyon Formation, an early Maastrichtian cool-climate assemblage from Alberta, with special reference to the Albertosaurus sarcophagus bonebed. Can J Earth Sci. 47(9):1159–1181. doi:10.1139/E10-005.
- Lauder GV. 1995. On the inference of function from structure. In: Thomason JJ, editor. Functional morphology in vertebrate paleontology. Cambridge (UK): Cambridge University Press; p. 1–18.
- Laws RM. 1970. Elephants as agents of habitat and landscape change in East Africa. Oikos. 21(1):1–15. doi:10.2307/3543832.
- Leach CT, Hoffman E, Dodson P. 2021. The promise of taphonomy as a nomothetic discipline: taphonomic bias in two dinosaur-bearing faunas in North America1. Can J Earth Sci. 58(9):852–869. doi:10.1139/cjes-2020-0176.
- Lehman TM. 2001. Late Cretaceous dinosaur provinciality. In: Tanke DH, Carpenter K, editors. Mesozoic vertebrate life. Bloomington: Indiana University Press; p. 310–328.
- Longrich NR. 2016. A ceratopsian dinosaur from the Late Cretaceous of eastern North America, and implications for dinosaur biogeography. Cretac Res. 57:199–207. doi:10.1016/j.cretres.2015.08.004.
- Lucas PW. 2004. Dental functional morphology: how teeth work. Cambridge (UK): Cambridge University Press.
- Lull RS, Wright NE. 1942. Hadrosaurian dinosaurs of North America. Geol Soc Am Spec Pap. 40:1–238.
- Lyons SK, Smith FA. 2013. Macroecological patterns of body size in mammals across time and space. In: Smith FA, Lyons SK, editors. Body size: linking pattern and process across space, time, and taxonomy. Chicago (IL): University of Chicago Press; p. 116–146.
- Mallon JC. 2019. Competition structured a Late Cretaceous megaherbivorous dinosaur assemblage. Sci Rep. 9:15447. doi:10.1038/s41598-019-51709-5.
- Mallon JC, Anderson JS. 2013. Skull ecomorphology of megaherbivorous dinosaurs from the Dinosaur Park Formation (upper Campanian) of Alberta, Canada. PLoS ONE. 8(7):e67182. doi:10.1371/journal.pone.0067182.
- Mallon JC, Anderson JS. 2014a. Implications of beak morphology for the evolutionary paleoecology of megaherbivorous dinosaurs from the Dinosaur Park Formation (upper Campanian) of Alberta, Canada. Palaeogeogr Palaeoclimatol Palaeoecol. 394:29–41. doi:10.1016/j.palaeo.2013.11.014.
- Mallon JC, Anderson JS. 2014b. The functional and palaeoecological implications of tooth morphology and wear for the megaherbivorous dinosaurs from the Dinosaur Park Formation (upper Campanian) of Alberta, Canada. PLoS ONE. 9(6):e98605. doi:10.1371/journal.pone.0098605.
- Mallon JC, Evans DC. 2014. Taphonomy and habitat preference of North American pachycephalosaurids (Dinosauria, Ornithischia). Lethaia. 47(4):567–578. doi:10.1111/let.12082.
- Mallon JC, Evans DC, Ryan MJ, Anderson JS. 2013. Feeding height stratification among the herbivorous dinosaurs from the Dinosaur Park Formation (upper Campanian) of Alberta, Canada. BMC Ecol. 13:14. doi:10.1186/1472-6785-13-14.
- Maryańska T, Chapman RE, Weishampel DB. 2004. Pachycephalosauria. In: Weishampel DB, Dodson P, Osmólska H, editors. The Dinosauria. 2nd ed. Berkeley and Los Angeles: University of California Press; p. 464–477.
- Maryańska T, Osmólska H. 1974. Pachycephalosauria, a new suborder of ornithischian dinosaurs. Palaeontol Polonica. 30:45–102.
- McLeay LJ, Page B, Goldsworthy SD, Ward TM, Paton DC. 2009. Size matters: variation in the diet of chick and adult crested terns. Mar Biol. 156:1765–1780. doi:10.1007/s00227-009-1211-4.
- Miller TE, Rudolf VH. 2011. Thinking inside the box: community-level consequences of stage-structured populations. Trends Ecol Evol. 26(9):457–466. doi:10.1016/j.tree.2011.05.005.
- Nakazawa T. 2014. Ontogenetic niche shifts matter in community ecology: a review and future perspectives. Popul Ecol. 57(2):347–354. doi:10.1007/s10144-014-0448-z.
- Norman DB. 2020. Scelidosaurus harrisonii (Dinosauria: Ornithischia) from the Early Jurassic of Dorset, England: biology and phylogenetic relationships. Zool J Linn Soc. 191(1):1–86. doi:10.1093/zoolinnean/zlaa061.
- O’Gorman EJ, Hone DW. 2012. Body size distribution of the dinosaurs. PLoS ONE. 7(12):e51925. doi:10.1371/journal.pone.0051925.
- Olson EC. 1952. The evolution of a Permian vertebrate chronofauna. Evolution. 6(2):181–196. doi:10.2307/2405622.
- Ostrom JH. 1961. Cranial morphology of the hadrosaurian dinosaurs of North America. Bull Am Mus Nat Hist. 122(2):39–186.
- Ostrom JH. 1966. Functional morphology and evolution of the ceratopsian dinosaurs. Evolution. 20(3):290–308. doi:10.2307/2406631.
- Owen-Smith RN. 1988. Megaherbivores: the influence of very large body size on ecology. Cambridge (UK): Cambridge University Press.
- Padian K, Horner JR. 2004. Dinosaur physiology. In: Weishampel DB, Dodson P, Osmólska H, editors. The Dinosauria. 2nd ed. Berkeley and Los Angeles: University of California Press; p. 660–671.
- Pagel M. 2002. Modelling the evolution of continuously varying characters on phylogenetic trees: the case of hominid cranial capacity. In: MacLeod N, Forey PL, editors. Morphology, shape and phylogeny. Boca Raton (FL): CRC Press; p. 269–286.
- Pearson DA, Schaefer T, Johnson KR, Nichols DJ, Hunter JP. 2002. Vertebrate biostratigraphy of the Hell Creek formation in southwestern North Dakota and northwestern South Dakota. Geol Soc Am Spec Pap. 361:145–167.
- Platt SG, Rainwater TR, Finger AG, Thorbjarnarson JB, Anderson TA, McMurray ST. 2006. Food habits, ontogenetic dietary partitioning and observations of foraging behaviour of Morelet’s crocodile (Crocodylus moreletii) in Northern Belize. Herpetol J. 16(3):281–290.
- Polly PD, Lawing AM, Fabre A, Goswami A. 2013. Phylogenetic principal components analysis and geometric morphometrics. Hystrix. 24(1):1–9. doi:10.4404/hystrix-24.1-6383.
- R Core Team. 2019. R: a language and environment for statistical computing [software]. Version 4.1.0. Accessed 11 2021 Vienna (AT): R Foundation for Statistical Computing. https://www.R-project.org/
- Rull V. 2014. Time continuum and true long-term ecology: from theory to practice. Front Ecol Evol. 2(75). doi:10.3389/fevo.2014.00075.
- Ryan MJ, Evans DC, Currie PJ, Brown CM, Brinkman D. 2012. New leptoceratopsids from the upper Cretaceous of Alberta, Canada. Cretac Res. 35:69–80. doi:10.1016/j.cretres.2011.11.018.
- Salgado L, Canudo JI, Garrido AC, Moreno-Azanza M, Martínez LCA, Coria RA, Gasca JM. 2017. A new primitive Neornithischian dinosaur from the Jurassic of Patagonia with gut contents. Sci Rep. 7:42778. doi:10.1038/srep42778.
- Sánchez‐Hernández J, Nunn AD, Adams CE, Amundsen P. 2019. Causes and consequences of ontogenetic dietary shifts: a global synthesis using fish models. Biol Rev. 94(2):539–554. doi:10.1111/brv.12468.
- Schroeder KS, Lyons K, and Smith FA. 2021. The influence of juvenile dinosaurs on community structure and diversity. Science. 371(6532):941–944 doi:10.1126/science.abd9220.
- Sinclair ARE, Mduma S, Brashares JS. 2003. Patterns of predation in a diverse predator–prey system. Nature. 425:288–290. doi:10.1038/nature01934.
- Spencer LM. 1995. Morphological correlates of dietary resource partitioning in the African Bovidae. J Mamm. 76(2):448–471. doi:10.2307/1382355.
- Stayton CT. 2005. Morphological evolution of the lizard skull: a geometric morphometrics survey. J Morphol. 263(1):47–59. doi:10.1002/jmor.10288.
- Stroik LK. 2014. Dietary competition in an extant mammalian guild: application of a quantitative method to evaluate reconstructed niche overlap in paleocommunities. Int J Primatol. 35:1222–1252. doi:10.1007/s10764-014-9793-1.
- Subalusky AL, Fitzgerald LA, Smith LL. 2009. Ontogenetic niche shifts in the American Alligator establish functional connectivity between aquatic systems. Biol Conserv. 142(7):1507–1514. doi:10.1016/j.biocon.2009.02.019.
- Symonds MRE, Blomberg SP. 2014. A primer on phylogenetic generalised least squares. In: Garamszegi L, editor. Modern phylogenetic comparative methods and their application in evolutionary biology. Berlin (DE): Springer; p. 105–130.
- Therrien F, Zelenitsky DK, Voris JT, Tanaka K. 2021. Mandibular force profiles and tooth morphology in growth series of Albertosaurus sarcophagus and Gorgosaurus libratus (Tyrannosauridae: Albertosaurinae) provide evidence for an ontogenetic dietary shift in tyrannosaurids. Can J Earth Sci. 58(9):812–828. doi:10.1139/cjes-2020-0177.
- Titus AA, Loewen MA. 2013. At the top of the grand staircase: the Late Cretaceous of southern Utah. Bloomington (IN): Indiana University Press.
- Tyler CL, Leighton LR. 2011. Detecting competition in the fossil record: support for character displacement among Ordovician brachiopods. Palaeogeogr Palaeoclimatol Palaeoecol. 307(1–4):205–217. doi:10.1016/j.palaeo.2011.05.020.
- Van Valkenburgh B. 1994. Ecomorphological analysis of fossil vertebrates and their paleocommunities. In: Wainwright PC, Reilly SM, editors. Ecological morphology: integrative organismal biology. Chicago (IL): University of Chicago Press; p. 140–166.
- Van Wieren SE, Van Langevelde F. 2008. Structuring herbivore communities: the role of habitat and diet. In: Prins HHT, and Van Langevelde F, editors. Resource ecology: spatial and temporal aspects of foraging. Dordrecht (Netherlands): Springer; p. 237–262.
- Varriale FJ. 2016. Dental microwear reveals mammal-like chewing in the neoceratopsian dinosaur Leptoceratops gracilis. PeerJ. 4: e2132. doi: 10.7717/peerj.2132.
- Varricchio DJ. 2011. A distinct dinosaur life history? Hist Biol. 23(1): 91–107. doi:10.1080/08912963.2010.500379.
- Wang S, Stiegler J, Amoit R, Wang X, Du G, Clark JM, and Xu X. 2017. Extreme Ontogenetic Changes in a Ceratosaurian Theropod. Curr Biol. 27(1): 144–148. doi:10.1016/j.cub.2016.10.043.
- Weishampel DB. 1984. Evolution of jaw mechanisms in ornithopod dinosaurs. Adv Anat Embryol Cell Biol. 87:1–109. doi:10.1007/978-3-642-69533-9.
- Werner EE, and Gilliam JF. 1984. The Ontogenetic Niche and Species Interactions in Size-Structured Populations. Annu Rev Ecol Syst. 15:393–425. doi:10.1146/annurev.es.15.110184.002141.
- Whitlock JA. 2011. Inferences of Diplodocoid (Sauropoda: Dinosauria) Feeding Behavior from Snout Shape and Microwear Analyses. PLoS ONE. 6(4): e18304. doi:10.1371/journal.pone.0018304.
- Wilson DJ. 2019. The harmonic mean p-value for combining dependent tests. Proc Natl Acad Sci. 116(4):1195–1200. doi:10.1073/pnas.1814092116.
- Woodruff DC, Carr TD, Storrs GW, Waskow K, Scannella JB, Nordén KK, and Wilson JP. 2018. The Smallest Diplodocid Skull Reveals Cranial Ontogeny and Growth-Related Dietary Changes in the Largest Dinosaurs. Sci Rep 8:14341. doi:10.1038/s41598-018-32620-x.
- Wyenberg-Henzler T 2020. Ontogenetic niche shifts in megaherbivorous dinosaurs of Late Cretaceous North America and their ecological implications [master’s thesis]. Ottawa (ON): Carleton University.