Abstract
Fouling-release (FR) coatings minimise the adhesion strength of fouling organisms. This study describes improved technologies to control the settlement and adhesion of the important fouling organism Mytilus galloprovincialis by incorporating the nanofillers titanium dioxide (TiO2) and carbon nanotubes (CNTs) in polydimethylsiloxane (PDMS) matrices. The incorporation of TiO2 prevented larval settlement when photoactivated with UV light, even at the lowest concentration of the nanofiller (3.75 wt%). Notably, there was 100% mortality of pediveligers exposed to photoactivated TiO2. However, plantigrades initially settled to photoactivated TiO2, but their adhesion strength was significantly reduced on these surfaces in comparison to blank PDMS. In addition, plantigrades had high mortality after 6 h. In contrast to the enhanced antifouling and FR properties of PDMS incorporating TiO2, the incorporation of CNTs had no effect on the settlement and adhesion of M. galloprovincialis.
Introduction
The negative economic impacts of biofouling are well documented (reviewed by Braithwaite and McEvoy Citation2005; Edyvean Citation2010; Flemming et al. Citation2010) and affect all marine industries, including finfish aquaculture (de Nys and Guenther Citation2009; Fitridge et al. Citation2012), offshore and deep-sea structures used in the oil and gas industry (Apolinario and Coutinho Citation2009; Page et al. Citation2010), power stations (Henderson Citation2010), desalination plants (Henderson Citation2010), and shipping (Townsin Citation2003; Schultz et al. Citation2011). Mussels, in particular, are highly successful fouling organisms (Briand Citation2009) that incur high management and control costs (Page et al. Citation2010). Their success as foulers is due to their wide physiological tolerances, ability to withstand air exposure, high fecundity, and their ability to settle on a wide variety of substrata in high densities (Gosling Citation2003). The blue mussel Mytilus galloprovincialis is a particularly important fouling species with high recruitment rates and the ability to outcompete other species (Erlandsson et al. Citation2006).
Past and present antifouling (AF) technologies mostly rely on metal-based toxic coatings that kill the settling propagules of fouling organisms (Finnie and Williams Citation2010). However, the development of non-biocidal technologies is gaining increasing attention, with surface modification for fouling-release (FR) being the most promising AF alternative to toxic biocides (Vladkova Citation2010; Webster and Chisholm Citation2010). The concept of reducing the adhesion of biofouling using FR coatings has been commercially applied since 1987 and has substantially influenced the design of AF technologies (Townsin and Anderson 2009). Current FR coatings are based on materials with low surface energies including silicone elastomers, fluoropolymers, flourosilicones and hybrids of siloxanes with other materials (Anderson et al. Citation2003; Webster and Chisholm Citation2010). As initial settlement is not prevented, hydrodynamic shear forces are required to remove attached organisms (Finnie and Williams Citation2010). Therefore, FR coating systems are ineffective in environments with low shear forces, including slow moving or moored vessels, as well as aquaculture and oil and gas assets. There are many ways to improve these coatings and a broad range of technologies can be incorporated to enhance FR coatings (Scardino and de Nys Citation2011).
Polydimethylsiloxane (PDMS) is a model FR material well suited for investigating surface modifications to either increase or decrease settlement and enhance the detachment (release) of fouling organisms (Carman et al. Citation2006; Schumacher et al. Citation2007a, Citation2007b; Long et al. Citation2010; Martinelli et al. Citation2011). Surface microtopography features on PDMS matrices (Callow et al. Citation2002; Carman et al. Citation2006) have been shown to either enhance (Carl et al. Citation2012) or reduce (Aldred et al. Citation2010) the settlement and attachment strength of sessile organisms. In general, surface microtopographies slightly smaller than the size of the settling organism result in a reduced contact area available for adhesion and attachment (Berntsson et al. Citation2000; Callow et al. Citation2002; Scardino et al. Citation2006, Citation2008). As a consequence, the settling organism requires more energy to settle (Callow et al. Citation2002) and the number of attachment points is reduced (Scardino et al. Citation2006), resulting in reduced adhesion strength and enhanced FR properties (Aldred et al. Citation2010; Carl et al. Citation2012).
A further approach to enhance the release characteristics of these coatings is through incorporating nanofillers, which are fillers with at least one of their dimensions in the nanometer range, to reinforce polymers and manipulate mechanical properties (Sadhu and Bhowmick Citation2008). Titanium dioxide (TiO2) is a filler in that it has a photo-induced hydrophilic effect resulting in hydrophobic surfaces containing TiO2 becoming hydrophilic under UV exposure (Fujishima et al. Citation2008). Moreover, TiO2 is a photocatalyst that has the potential to improve AF properties against a broad range of fouling organisms due to its photocatalytic effect. TiO2 is used to decompose organic compounds and its photocatalytic effect has been applied for the destruction of bacteria, viruses and cancer cells (Ho et al. Citation2003; Hajkova et al. Citation2007; Lee et al. Citation2010).
Notably, photoreactions are limited to the surface of the photoexcited photocatalyst (TiO2) and therefore only molecules that are in direct contact with the catalyst surface undergo photocatalytic degradation (Gaya and Abdullah Citation2008). The photocatalytic effect is restricted to the surface due to a high reaction rate constant of the hydroxyl radicals formed (106 – 1011 dm3 mol−1 s−1; Bhatkhande et al. Citation2002). This restricts the photo-killing zone to the near-surface thereby potentially enhancing the AF attributes of non-biocidal FR coatings whilst minimising effects on non-target organisms. However, there has been no effort to deconstruct the effect of TiO2 on the settlement of key fouling organisms other than bacteria and diatoms, where materials were successful in eliminating organisms from surfaces (Ho et al. Citation2003; Kemmitt et al. Citation2012). In addition, the incorporation of TiO2 also improves a wide range of mechanical properties of elastomers, including tensile strength, elastic modulus, hardness, abrasion resistance and energy to break (Mirabedini et al. Citation2008).
Carbon nanotubes (CNTs) are an alternative nanofiller to improve FR properties. CNTs are cylindrical nanostructures formed by covalently bonded carbon atoms arranged in a hexagonal array (Thorstenson et al. Citation2001). The incorporation of CNTs affects the surface topography of the base material, as well as its wettability when immersed in water, and as a result influences the detachment of fouling organisms (Beigbeder et al. Citation2009). The FR performance of coatings depends on the homogeneous dispersion of CNTs in the polymeric matrix and the interaction between both materials (Beigbeder et al. Citation2010). Furthermore, the concentration of CNTs is critical to the enhancement of FR properties (Beigbeder et al. Citation2008, Citation2010). Another important property of CNTs is their ability to mechanically reinforce silicone elastomers when dispersed in the polymeric matrix (Frogley et al. Citation2003; Paul et al. Citation2006; Tjong Citation2006; Ci et al. Citation2008).
The major objective of this study was to improve technologies to control the settlement of the important fouling organism M. galloprovincialis by incorporating the nanofillers TiO2 and CNTs in PDMS, and also improve the efficacy of PDMS FR coatings by minimising bio-adhesion. To determine the efficacy of nanofilled (TiO2 and CNTs) PDMS, the settlement and adhesion strength of pediveligers (mussel larvae competent to settle) and plantigrades (post larvae) of M. galloprovincialis was quantified. These two ontogenic stages represent primary and secondary settlers with different settlement preferences (Buchanan and Babcock Citation1997; Alfaro and Jeffs Citation2002). Firstly, PDMS matrices filled with TiO2 in the range 3.75–15 weight percent (wt%), combined with microtexture with groove widths of 200, 300 and 600 μm, were assayed for effects on settlement and adhesion strength. Secondly, CNTs were incorporated into PDMS matrices in the range 0.2–6 wt% with the objective of reducing settlement and adhesion strength. In addition, the effect of these incorporated nanofillers on the mechanical properties of the surface of the PDMS base material was quantified.
Material and methods
Culture of pediveligers and plantigrades
Larvae and plantigrades of the mussel M. galloprovincialis were supplied by the Victorian Shellfish Hatchery in Queenscliff, Victoria, Australia. Spawning and larval production are described in detail in Pettersen et al. (Citation2010). Veliger larvae (21 days after fertilisation) and plantigrades (57 days after fertilisation) were shipped within 14 h to James Cook University, Townsville, Queensland, Australia. Larvae and plantigrades were reared in a laboratory culture as described in detail by Carl et al. (Citation2011). Mussel larvae were maintained until the pediveliger stage was reached and competent to settle. Pediveligers were then pooled and used in settlement assays. Plantigrades were maintained for 9–15 days in the laboratory prior to use in settlement assays.
Laboratory experiments
TiO2 in textured PDMS
Production of surfaces. PDMS (Sylgard 184; Dow Corning, USA) was used as a silicone elastomer to produce the micro-structured test surfaces with TiO2 (Degussa TiO2 P25) filler. PDMS was prepared using a 10:1 ratio of base elastomer (part A) to curing agent (part B). The nanofiller TiO2 (a mixture of 79.5% anatase and 20.5% rutile) with an average primary particle size of 21 nm (specified by the manufacturer) was incorporated into the PDMS matrix at concentrations of 0 (blank control), 3.75, 7.5, 11.25 and 15 wt%. The two components were mixed thoroughly and degassed in a vacuum desiccator to remove bubbles. Casting of the blank and TiO2-filled PDMS followed the production procedure described in Carl et al. (Citation2012). Smooth PDMS and microtextures with groove widths of 200, 300, and 600 μm were generated. Microtextured surfaces had a linear grating of approximately square wave profile and a mark-spacing ratio of 1 (groove width = land area width).
Characterisation of surfaces
To determine the effect of the nanofiller on surface topography, the smooth nanocomposites were coated with platinum–palladium (Pt–Pd; Cressington 208HR Sputter Coater, Cressington Scientific Instruments Ltd, UK) and images of the test surfaces were taken at random points using scanning electron microscopy (SEM; Hitachi S-4300 SE/N) at up to 3000× magnification. The distribution of TiO2 particles on the surfaces and throughout the PDMS matrices was examined using backscattered SEM. To quantify the effect of incorporated TiO2 on the properties of PDMS, the hardness and contact angles of the smooth nanocomposites were measured. Hardness was quantified using a Shore Durometer (Durometer 1600 Shore OO; Shore Instrument & Mfg Co. Inc., USA) at random locations (n = 6). Contact angles θ were measured with a goniometer (First Ten Ångstroms, FTÅ200, USA) using the sessile drop method with a 2 μl Milli-Q water droplet described by Carl et al. (Citation2012). Contact angle measurements were carried out at different locations on each sample (n = 5) before and after the exposure to UV within 5 min after the removal from UV light. The test surfaces were placed in a QUV Accelerated Weathering Tester unit for 6 h (Q Panel Lab Products, USA) and a UV Irradiance Controller was used to control the UV intensity (UV A and B intensity: 1.5 mW cm−2).
Settlement assays
To determine the effect of TiO2 and microtopography on the settlement and attachment strength of pediveligers and plantigrades of M. galloprovincialis, TiO2 was incorporated into PDMS as described above. The blank control and each of the TiO2-filled PDMS materials were cast in a linear grating of square wave profile with features widths of 200, 300, and 600 μm and a smooth cast without any surface topography (n = 12 for pediveligers and plantigrades). Circles (25 mm diameter) were cut from the PDMS surfaces using a hole punch, washed with a detergent solution (Decon 90; Decon Laboratories Ltd), rinsed with distilled water and air dried before use in assays. Circles were randomly placed in covered 6-well plates (IWAKI 3810-006) to prevent evaporation and for easier handling. Static drop settlement assays were performed with pediveligers and plantigrades as described by Carl et al. (Citation2011). For settlement assays with pediveligers, ∼20 individuals were pipetted in a 800 μl drop of filtered seawater (FSW; 0.2 μm filtered and UV sterilised) onto the test surfaces. Due to the larger size of plantigrades, only five individuals were used in each drop assay. To test for the effect of photocatalytic TiO2, half of the replicates of each test surface (n = 6 for pediveligers and plantigrades) were placed in a temperature controlled culture cabinet (Sanyo MLR-351) at 18°C without light. The other half of the replicates of each test surface (n = 6 for pediveligers and plantigrades) were placed in a temperature controlled room at 18°C under a UV light bank (UV A and B intensity: 1.5 mW cm−2) in a 12 h light:12 h dark cycle. The light bank consisted of 12 Cosmedico 15508 180W RA plus Cosmolux lights (280–400 nm) and 11 Phillips Master TL5 HO 49W/865 lights (400–700 nm). To minimise temperature fluctuations, the 6-well plates holding the test surfaces were placed in a circulating water bath (18°C) and a fan was used to enhance air circulation under the UV lights. The settlement of pediveligers and plantigrades was determined after 48 h and 6 h, respectively. Pediveligers and plantigrades were considered settled when they attached to the surface with byssal threads.
Adhesion strength
To determine the adhesion strength of settled pediveligers and plantigrades of M. galloprovincialis on each surface, a flow chamber was used following Carl et al. (Citation2012). The test surfaces were carefully removed from the 6-well plates using forceps and then randomly placed in polyvinylchloride (PVC) plates. Each PVC plate had three machined holes side by side, where the test plugs fitted precisely. To secure the test surfaces to the plate, the test plugs were fixed with cloth tape (Scotch® Colored Duct Tape 330BLU-DC-NA) from the bottom. One PVC plate at a time was placed in the flow chamber with test surface grooves parallel to the flow and secured in the raceway using customised fittings. A small PVC ramp was placed in front of the plate holding the test surfaces to ensure an even flow over the samples. The test surfaces were exposed to 4 knots (equal to 2.06 m s−1) for 2 min after which the number of remaining mussels was counted.
CNTs in PDMS
Production of surfaces
PDMS (Sylgard 184; Dow Corning, USA) was used as the silicone elastomer to produce the test surfaces and was prepared using a 10:1 ratio of base elastomer (part A) to curing agent (part B). Multi-walled CNTs (S-WNT-60100) with an outer diameter of 60–100 nm, length of 5–15 μm and a purity of > 98%, were purchased from Shenzhen Nanotech Port Co. Ltd (China). To mix the CNTs into the PDMS matrix at concentrations of 0.2, 0.5, 1, 2, 4, and 6 wt%, CNTs were dispersed in isopropyl alcohol and then placed in an ultrasonic system (Branson 3200 ultrasonic bath and Branson Sonifier B30; Branson Instruments, Danbury, USA) and sonicated for 5 h. Once the CNTs were fully dispersed, the base elastomer (part A) was added, mechanically mixed, and the isopropyl alcohol then allowed to evaporate under constant air flow with complete removal confirmed by constant weight measurements. After the isopropyl alcohol was evaporated, the curing agent (part B) was added and stirred in thoroughly. The mixture was de-gassed in a vacuum desiccator to remove bubbles before it was poured into stainless steel square moulds on a polypropylene surface.
Characterisation of surfaces
To determine the alignment of CNTs on the surface and their effect on surface topography, the nanocomposites were Pt–Pd coated and images were taken at random points using SEM (Hitachi S-4300 SE/N) up to 3000× magnification. The distribution and orientation of CNTs throughout PDMS matrices was examined by cutting cross-sections of the samples prior to SEM. To determine the effect of incorporated CNTs on the properties of PDMS, the hardness and contact angles were quantified as described above.
Settlement assays
To quantify the effect of CNTs incorporated into PDMS on the settlement and attachment strength of pediveligers and plantigrades of M. galloprovincialis, CNTs were dispersed into a PDMS matrix as described. Blank PDMS was used as a control and circles (35 mm diameter) were cut from all test surfaces using a hole punch. There were six replicates for each surface for each ontogenetic stage and consequently 42 test surfaces in total for assays with pediveligers and plantigrades. Test surfaces were randomly placed in PVC plates as previously described. This minimises handling of the surfaces between settlement assays and subsequent adhesion strength measurements in the flow chamber. The surfaces were washed with a detergent solution (Decon 90; Decon Laboratories Ltd), rinsed with distilled water, and air dried prior to use in assays.
Static drop settlement assays (n = 6 for pediveligers and plantigrades) were performed as previously described. Drop assays were covered individually to prevent evaporation (IWAKI 1000-053). Settlement assays were conducted in a temperature controlled culture cabinet (Sanyo MLR-351) at 18°C in a 12 h light:12 h dark cycle. The settlement of pediveligers and plantigrades was determined after 48 h and 6 h, respectively. Pediveligers and plantigrades were considered settled when they attached to the test surface with byssal threads.
Adhesion strength
The adhesion strength measurements followed the methodology described above (see TiO2 in textured PDMS).
Statistical analysis
Data were analysed by one- or two-factor permutational multivariate analysis of variance (PERMANOVA). The Bray–Curtis dissimilarity measure was used for all PERMANOVAs and P-values were calculated using unrestricted permutation of untransformed raw data with 9999 random permutations. If there was a significant difference, pair-wise a posteriori comparisons were made among the significant groups using the Bray–Curtis similarity measure (α = 0.05). To analyse the adhesion strength data, surfaces without initial settlement were excluded from PERMANOVAs. Statistical analyses were performed using PRIMER 6 (v. 6.1.13) and PERMANOVA+ (v. 1.0.3) (Clarke and Gorley Citation2006). Data are reported as mean ±1 standard error (SE).
Correlations between the number of settled and remaining individuals after being exposed to a water flow of 4 knots were assessed using Pearson's product-moment correlation using SPSS (v. 19).
Results
TiO2 in textured PDMS
Surface characterisation
The PDMS surfaces filled with TiO2 had no surface topography and did not differ from the blank control surface at the micron scale. Backscattered SEM showed a homogeneous dispersion of the nanofiller on the surfaces of the nanocomposites (a–e). However, TiO2 formed small aggregates at the highest concentration of 15 wt% TiO2 (e). In contrast, micrographs of cross-sections of the nanocomposites showed large aggregates of the nanofiller throughout the matrices at all concentrations (f–j).
Figure 1. Back scattered SEM images of (a–e) the surface and (f–j) cross-sections through filled PDMS with various TiO2 concentrations. (a, f) Blank PDMS controls; (b, g) PDMS matrices with 3.75 wt% TiO2; (c, h) 7.5 wt% TiO2; (d, i) 11.25 TiO2; and (e, j) 15 wt% TiO2. Light coloured spots are TiO2 particles.
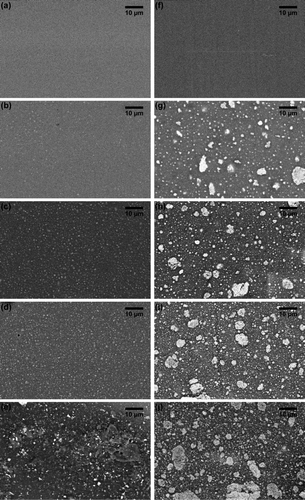
The hardness of PDMS increased with the addition of increasing amounts of TiO2 (). Consequently, the blank control was the softest surface with 84.1 ± 0.3 Durometer (Scale OO) and the hardness increased with the incorporation of TiO2 up to 87.0 ± 0.3 Durometer (Scale OO) for the nanocomposite with the highest TiO2 concentration (15 wt% TiO2).
Table 1. Mechanical properties of nanocomposites containing (a) TiO2 and (b) CNTs.
The incorporation of TiO2 had an effect on the surface wettability (a). The hydrophobicity of the TiO2 filled matrices was lower than the blank PDMS control as shown by the contact angle measurements before the exposure to UV. While the contact angle of the blank PDMS remained unchanged after UV exposure, the hydrophobicity of the TiO2-filled surfaces increased after exposure to UV light for 6 h. Notably, the TiO2 matrices generated static electricity charges, which may cause errors in contact angle measurements. This charge effect was noted as the generated electrostatic field pulled the water droplet to the test surface more strongly than in the absence of charge (ASTM Citation2004), resulting in lower contact angles for statically charged surfaces. The surfaces did not show a static charge for contact angle measurements after 6 h of UV exposure.
Settlement assay
The combination of TiO2 and photoactivation (TiO2 exposed to UV light) completely inhibited the settlement of all pediveligers of M. galloprovincialis at all tested concentrations of TiO2 (a). Notably, all pediveligers on surfaces filled with photoactivated TiO2 were dead after 48 h. In contrast, pediveligers on blank surfaces were alive at the end of the experiment. Therefore, the strong and significant effect of TiO2 (a) is based on its photoactivation, whereas UV light itself had no effect on survival of pediveligers. Consequently, settlement only occurred on control surfaces without TiO2 in assays conducted under UV exposure. Among these, the lowest number of pediveligers settled on smooth PDMS (5.7 ± 1.5%) and increased with increasing size of texture from 200 μm (5.9 ± 3.4) to 600 μm (16.5 ± 6.4%). However, these increases were not significant (a).
Figure 2. Settlement (%) of pediveligers of M. galloprovincialis on textured (200, 300, 600 μm) and smooth PDMS with different TiO2 concentrations (0, 3.75, 7.5, 11.25, 15 wt%). Assays were conducted (a) under UV exposure and (b) in the dark. Means ± SE are shown (n = 6).
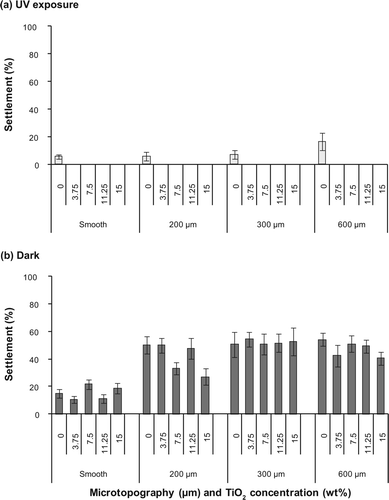
Table 2. PERMANOVA analysis on Bray–Curtis distances for differences in settlement of pediveligers (a) under UV exposure and (b) in the dark on surfaces with different microtextures (smooth, 200, 300, 600 μm) and TiO2 concentrations (0, 3.75, 7.5, 11.25, 15 wt%).
In contrast, TiO2 without photoactivation (assays conducted in the dark) had no effect on the settlement of pediveligers, while microtopography significantly enhanced the settlement of pediveligers (b, b). Settlement on smooth PDMS surfaces was significantly lower with an overall mean settlement of 15.3 ± 0.6%, compared to 41.5 ± 1.2%, 51.9 ± 2.0%, and 47.4 ± 1.5% on 200, 300, and 600 μm textured surfaces, respectively. Settlement on 300 μm topographies was significantly higher than on other tested microtopographies (b). No mortalities of pediveligers were observed when settlement assays were conducted in the dark.
For settlement assays with plantigrades under UV exposure, neither microtexture nor the incorporation of TiO2 had a significant effect on the settlement of plantigrades (a). More than 56% plantigrades settled on all surfaces under UV exposure, regardless of their microtopography and TiO2 concentration (a). The lowest number of plantigrades settled on smooth PDMS filled with 11.25 wt% TiO2 (56.7 ± 6.2%), while the highest settlement occurred on surfaces with 300 μm texture without TiO2 (90.0 ± 6.8%). Although plantigrades settled initially, all individuals were dead after 6 h on all TiO2-filled surfaces when photoactivated under UV exposure. In contrast, all plantigrades were alive on blank surfaces exposed to UV confirming that UV itself had no effect on the survival of plantigrades.
Figure 3. Settlement (%) of and plantigrades of M. galloprovincialis on textured (300, 600 μm) and smooth PDMS with different TiO2 concentrations (0, 3.75, 7.5, 11.25, 15 wt%). Assays were conducted (a) under UV exposure and (b) in the dark. Means ± SE are shown (n = 6).
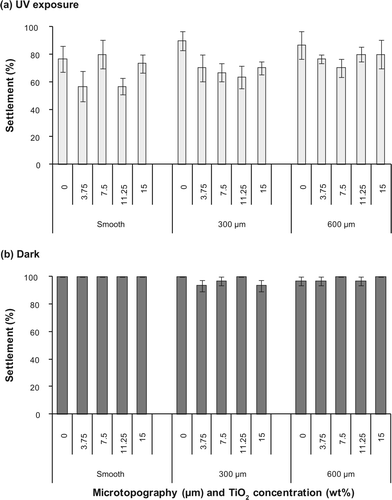
Table 3. PERMANOVA analysis on Bray–Curtis distances for differences in settlement of plantigrades (a) under UV exposure and (b) in the dark on surfaces with different microtextures (smooth, 300, 600 μm) and TiO2 concentrations (0, 3.75, 7.5, 11.25, 15 wt%).
When settlement assays were conducted in the dark, the overall settlement of plantigrades within 6 h was high on all surfaces ranging from 93.3 ± 4.2% to 100.0 ± 0.0% (b). Similar to assays conducted under UV exposure, there was no effect of TiO2 or microtexture on the settlement of plantigrades in the dark (b). Notably, no mortalities of plantigrades occurred for assays conducted in the dark.
Adhesion strength
There was no initial settlement of pediveligers on surfaces filled with TiO2 and photoactivated under UV exposure. Consequently, the differences of adhesion strength among different TiO2 concentrations could not be determined. Settlement under UV exposure only occurred on control surfaces without TiO2, and among these pediveligers adhered most strongly to the 600 μm textures with 44.3 ± 13.6% pediveligers remaining after exposure to flow (a). The number of remaining pediveligers decreased with decreasing feature widths and detachment was highest on smooth PDMS surfaces (5.6 ± 5.6%). However, the effect of microtopography on the adhesion strength of pediveligers settled under UV exposure was not significant (a).
Figure 4. Remaining (%) pediveligers on textured (200, 300, 600 μm) and smooth PDMS with different TiO2 concentrations (0, 3.75, 7.5, 11.25, 15 wt%) after being exposed to a water flow of 4 knots for 2 min. Assays were conducted (a) under UV exposure and (b) in the dark. The percentage of remaining pediveligers after being exposed to flow is based on the settlement on test surfaces. Means ± 1 SE are shown (n = 6).
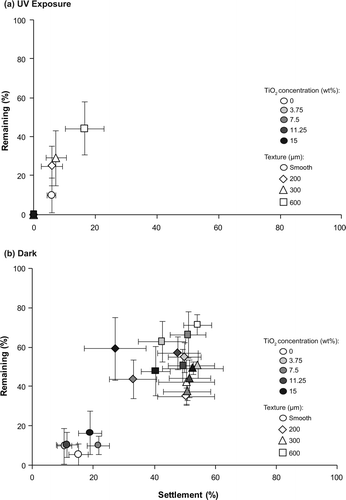
Table 4. PERMANOVA analysis on Bray–Curtis distances for differences in the number of remaining pediveligers when settled (a) under UV exposure and (b) in the dark on surfaces with different microtextures (smooth, 200, 300, 600 μm) and TiO2 concentrations (0, 3.75, 7.5, 11.25, 15 wt%) after the exposure to flow.
For assays conducted in the dark, TiO2 had no effect on the adhesion strength of pediveligers, while texture significantly affected adhesion (b). The adhesion strength of pediveligers was significantly weaker on smooth PDMS, with an overall mean of 10.6 ± 2.8% pediveligers remaining on all smooth surfaces. This compared to more than 44% remaining on all microtextured surfaces (b). Furthermore, adhesion was significantly stronger on microtextures with profiles of 600 μm than on profiles of 300 μm (b).
For plantigrades settled under UV exposure, adhesion strength on surfaces filled with TiO2 was significantly weaker than on blank control surfaces (a, a). On average, more than 82% of plantigrades remained on blank control surfaces exposed to UV, while the mean overall number remaining on TiO2 filled surfaces ranged between 32.3 ± 4.6% (11.25 wt% TiO2) and 49.3 ± 7.2% (7.5 wt% TiO2) (a).
Figure 5. Remaining (%) plantigrades on textured (300, 600 μm) and smooth PDMS with different TiO2 concentrations (0, 3.75, 7.5, 11.25, 15 wt%) after being exposed to a water flow of 4 knots for 2 min. Assays were conducted (a) under UV exposure and (b) in the dark. The percentage of remaining plantigrades after being exposed to flow is based on the settlement on test surfaces. Means ± 1 SE are shown (n = 6).
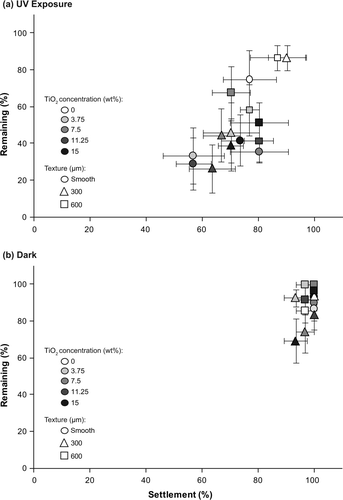
Table 5. PERMANOVA analysis on Bray–Curtis distances for differences in the number of remaining plantigrades when settled (a) under UV exposure and (b) in the dark on surfaces with different microtextures (smooth, 200, 300, 600 μm) and TiO2 concentrations (0, 3.75, 7.5, 11.25, 15 wt%) after the exposure to flow.
For assays conducted in the dark, TiO2 had no effect on the adhesion strength of plantigrades. Significantly fewer individuals remained on microtextures with profiles of 300 μm compared to profiles of 600 μm, with a mean overall remaining of 82.5 ± 4.8% and 94.8 ± 2.7% plantigrades, respectively (b, b).
Correlation of settled and remaining pediveligers and plantigrades
In general, highest numbers of pediveligers remained on surfaces with high initial larval settlement (a, b), with a significant correlation between settled and remaining pediveligers. This association was stronger when the settlement assay was conducted under UV exposure (Pearson's product–moment correlation: r = 0.797, P < 0.001; a) compared to the dark (r = 0.511, P < 0.001). For assays conducted in the dark, the low settlement and weak attachment of pediveligers settled on smooth PDMS is clearly seen with < 20% of attached individuals remaining after the exposure to flow (b). In a similar manner to pediveligers, the number of remaining plantigrades was also significantly correlated with settlement (a, b). However, in contrast to assays conducted with pediveligers, this association was stronger when the assay was conducted in the dark (r = 0.493, P < 0.001, b) and weaker for assays conducted under UV exposure (r = 0.254, P = 0.020; a).
CNTs in PDMS
Surface characterisation
The incorporation of CNTs resulted in the addition of surface features (topography) to the PDMS base material at the scale of microns (a, b), with more surface topographic features at higher concentrations. Cross-sections of nanocomposites show large aggregates of CNTs throughout the matrices at all concentrations (d), indicating an uneven dispersion of the CNTs. Although CNTs formed aggregates, there was dispersion of CNTs throughout the matrices as evidenced by the even black shade across each nanocomposite cross-section. The orientation of CNTs appeared to be random regardless of the aggregates (e, f). CNTs were only exposed from the base material when cut as micrographs of cross-sections show (d–f). Cut surfaces with exposed CNTs were not tested.
Figure 6. SEM images of (a, b) the surface and (c–f) cross-sections of (a, c) blank PDMS and (b, d–f) PDMS filled with 6 wt% CNTs. (d–f) Cross-sections of filled PDMS show CNTs protruding from the PDMS matrix where cut as illustrated in the (f) tilted view of a cross-section.
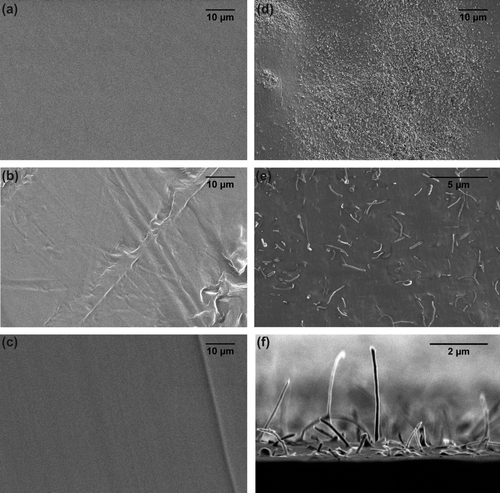
CNTs affected the material properties of the PDMS when embedded, with the mechanical property of hardness increasing with increasing amounts CNTs from 84.8 ± 0.3 Durometer (Scale OO) (blank PDMS) to 89.2 ± 0.5 Durometer (Scale OO) for the highest nanofiller concentration (6 wt% CNTs). In contrast, the change in surface wettability was marginal when CNTs were incorporated as a nanofiller (b). All nanocomposites remained hydrophobic with θ ≥ 110°.
Settlement assay
The incorporation of CNTs had no effect on the settlement of either pediveligers (one-factor PERMANOVA: F (6,35) = 1.03, P = 0.404) or plantigrades (F (6,35) = 1.09, P = 0.548). The settlement of pediveligers was below 30% on all test surfaces and there was no consistent trend between settlement and the incorporation of CNTs (a). The lowest number of pediveligers settled on PDMS surfaces filled with 4 wt% CNTs (17.2 ± 5.9%) and the highest number of pediveligers settled on PDMS filled with 1 wt% CNTs (29.5 ± 4.4%). The overall settlement of plantigrades within 6 h was generally high and ranged from 93.3 ± 4.2% to 100.0 ± 0.0% (b).
Adhesion strength
In a similar manner to the results of the settlement assays, there was no effect of the incorporation of CNTs on the adhesion strength of either pediveligers (one-factor PERMANOVA: F (6,33) = 0.40, P = 0.941) or plantigrades (F (6,35) = 0.53, P = 0.799). Less than 50% of pediveligers remained on all test surfaces after being exposed to a flow rate of 4 knots, while more than 85% plantigrades remained on all test surfaces (). Pediveligers adhered most strongly to PDMS surfaces filled with the highest concentration of CNTs (6 wt% CNTs; 49.4 ± 17.1% pediveligers remaining), while the lowest number of pediveligers remained on surfaces filled with 0.5 wt% CNTs (21.3 ± 11.4%). In contrast, the highest number of plantigrades remained on blank PDMS after the exposure to flow (96.7 ± 3.3%) and the lowest number of plantigrades remained on PDMS surfaces filled with the lowest concentration of CNTs (0.2 wt% CNTs; 85.8 ± 4.6%).
Figure 8. Correlation between initial settlement (%) and remaining (%) plantigrades and pediveligers of M. galloprovincialis on PDMS containing CNTs (0.2, 0.5, 1, 2, 4, 6 wt%) after being exposed to a water flow of 4 knots for 2 min. The percentages of remaining pediveligers and plantigrades are based on the settlement on test surfaces. Means ± SE are shown (n = 6).
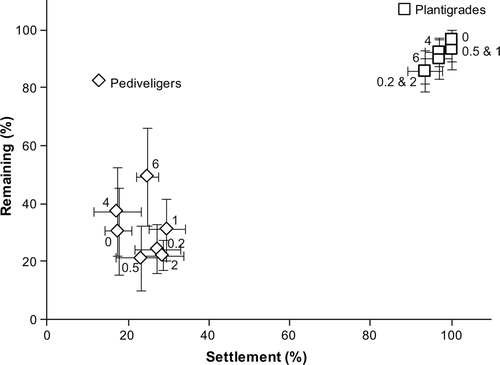
Correlation of settled and remaining pediveligers and plantigrades
There was no correlation between the initial settlement and the number of remaining pediveligers (Pearson's product–moment correlation: r =−0.011, P = 0.947) and plantigrades (r = 0.114, P = 0.474) on surfaces filled with CNTs ().
Discussion
This study tested the efficacy of incorporated TiO2 and CNTs nanofillers in PDMS matrices to improve AF and FR properties against the mussel M. galloprovincialis. The incorporation of TiO2 was a highly effective strategy to prevent larval settlement, killing pediveligers and plantigrades and weakening their adhesion strength when photoactivated. Notably, the incorporation of the photocatalyst TiO2 in a PDMS matrix was highly effective against the settlement of pediveligers, regardless of the concentration of TiO2 embedded. No pediveligers settled on any surfaces filled with photoactivated TiO2 and all pediveligers exposed to photoactivated TiO2 died after 48 h. Similarly, all plantigrades exposed to photoactivated TiO2 were dead by the end of the experiment (6 h), despite initial settlement (> 56%) to these surfaces. In addition, the adhesion strength of plantigrades on surfaces with photoactivated TiO2 was weaker than on blank controls, demonstrating improved FR properties by weakening adhesive bonding to the surfaces. In contrast, the incorporation of CNTs had no effect on either the settlement or adhesion of M. galloprovincialis.
In general, FR coatings based on silicone elastomers are inefficient against initial fouling, in particular diatoms (Holland et al. Citation2004; Molino et al. Citation2009; Dobretsov and Thomason Citation2011; Zargiel et al. Citation2011). The incorporation of TiO2 presents a promising solution to managing this initial and important stage of fouling. TiO2 is an ideal photocatalyst due to its wide availability, low production cost, photo-stability, chemical safety, and the high oxidising potential of photogenerated holes (Fujishima and Zhang Citation2006; Aprile et al. Citation2008). Silicone elastomers, such as PDMS, are resistant to photodegradation of TiO2 (Iketani et al. Citation2003) and transparent to UV light, which in turn allows photoactivation of the incorporated TiO2 (Gao and Liu Citation2003). Furthermore, TiO2 adheres strongly to silicone elastomers ensuring long-term photocatalytic activity (Silva et al. Citation2009). Overall, the use of a photocatalyst in FR coatings has the potential to minimise the build-up of biofilms, degrade chemical settling cues, prevent larval settlement, compromise adhesion strength, and kill macrofoulers as demonstrated in this study. Moreover, the incorporation of TiO2 clearly improves the efficacy of FR coating systems in environments with low shear forces. Importantly, only organisms in direct contact with the activated catalyst surface are exposed to the photocatalytic effects (Gaya and Abdullah Citation2008) thus reducing risks to non-target organisms. In addition, the risk of suspended photocatalytic nanoparticles causing phototoxicity to microalgae (Aruoja et al. 2008; Gladis et al. Citation2010; Miller et al. Citation2012) and adhesion of aggregates to aquatic organisms (Dabrunz et al. Citation2011) is low because the nanoparticles are incorporated into the PDMS matrix.
However, a limiting factor in the use of TiO2 as an AF technology is the dependence on UV light for photoactivation, which takes place at wavelengths of 300–388 nm when the photocatalytic surface (TiO2) is illuminated by light with energy above 3.2 eV (Wang et al. Citation2007; Gaya and Abdullah Citation2008). UV penetration in water decreases with increasing depth and can be impeded by water clarity (Conde et al. Citation2000). Therefore, the effectiveness of this AF approach is compromised in areas without UV exposure, including the inside of pipes and sea chests.
Non-activated TiO2 had no impact on the survival, settlement and adhesion strength of pediveligers and plantigrades in this study. For pediveligers, microtopography played a key role in settlement for assays conducted in the dark, while non-activated TiO2 had no effect. Microtopographic features significantly enhanced the settlement and adhesion strength of pediveligers, which is in accordance with previous studies where there was a positive effect of topographic features on larval settlement and retention (Filgueira et al. Citation2007; Gribben et al. Citation2011; Carl et al. Citation2012). In contrast, topographies had no effect on the settlement of plantigrades, which agrees with studies where metamorphosed mussels settle on all types of firm substrata within a short period of time (Seed Citation1976; Gosling Citation2003; Carl et al. Citation2011).
Notably, higher numbers of pediveligers and plantigrades settled on blank control surfaces when the settlement assays were conducted in the dark in comparison to assays conducted under UV exposure. While this observation coincides with studies identifying higher settlement rates of adult mussels in the dark (Kobak Citation2001), it is in contrast to previously reported enhanced settlement of pediveligers under a 12 h light:12 h dark regime compared to complete darkness (Carl et al. Citation2011). This is most probably due to the presence of UV light in this study that did not cause mortality but may affect settlement rates.
The increased hydrophobicity of TiO2 filled surfaces after exposure to UV contrasts with a photo-induced hydrophilic effect, where hydrophobic surfaces containing TiO2 become more hydrophilic (Fujishima et al. Citation2008). In this study, the TiO2 matrices initially generated an electrostatic charge, which pulled the water droplet to the test surface more strongly, resulting in lower contact angles for statically charged surfaces.
The incorporation of CNTs was ineffective in improving AF and FR properties against pediveligers and plantigrades of M. galloprovincialis. The embedment of CNTs caused minor topographic effects and resulted in a marginal increase in settlement of pediveligers in comparison to the blank PDMS control. However, CNTs incorporated in the PDMS matrix had no effect on the detachment of neither pediveligers nor plantigrades of M. galloprovincialis. Notably, the efficacy of CNTs in enhancing FR properties depends on the concentration of CNTs dispersed in the polymeric matrix although effective concentrations differ among species (Beigbeder et al. Citation2008). This has been demonstrated for the percentage removal of sporelings of U. linza by exposure to shear, which was doubled for CNT concentrations in the range of 0.05 to 1 wt% while the incorporation of CNTs at lower or higher concentrations did not result in enhanced FR performance (Beigbeder et al. Citation2010). CNT concentrations > 0.1 wt% are more likely to have bundle-like aggregates resulting in less efficient dispersion and reduced FR performance (Beigbeder et al. Citation2010). The use of CNT concentrations ≥ 0.2 wt% in the present study resulted in the formation of some aggregates. As a result, the detachment of pediveligers and plantigrades was similar among CNT concentrations tested and the blank control. Nevertheless, the incorporation of CNTs represents a promising approach to enhance FR performance. Cut surfaces in this study (f) may present a hostile surface for settlement due to the inherent high stiffness of the CNTs (1 TPa; Thorstenson et al. Citation2001), which may puncture exploring or settling organisms, forming a ‘wall of death’. As the properties of CNTs depend on atomic arrangement, morphology, diameter and length of the tubes (Thorstenson et al. Citation2001), this also provides an opportunity to further manipulate the characteristics of CNT spikes.
In conclusion, the incorporation of CNTs and TiO2 as nanofillers improved the hardness of a ‘weak’ silicone elastomer. The use of the photocatalyst TiO2 in AF and FR coatings is promising and effective. The advantages of using TiO2 are improved mechanical properties of weak coatings, enhanced FR properties, and photocatalytic destruction of a broad range of fouling organisms without effects on non-target organisms. The limitations and longevity of the photocatalytic activity, and the retention of TiO2 within the coating, require delineation and quantification. However TiO2 has been used successfully in self-cleaning tiles which are effective over a span of 20 years (Fujishima et al. Citation2008). Overall, the incorporation of TiO2 into coatings is a stepping stone aimed at improving AF technologies.
Acknowledgements
The authors thank the Fisheries Victoria and the Victorian Shellfish Hatcheries Pty Ltd for providing mussel larvae and their kind support. They also thank M. Page for help with taking SEM images and M. Spicer for help with the goniometer, N. Paul for statistical advice, as well as three anonymous reviewers for their helpful comments on the manuscript. Financial support was provided by CSIRO's Wealth from Oceans Flagship. C.C. was further supported by a CSIRO Flagship Collaboration Fund Postgraduate Top Up Scholarship and a James Cook University Postgraduate Research Scholarship.
References
- Aldred , N , Scardino , A , Cavaco , A , de Nys , R and Clare , AS . 2010 . Attachment strength is a key factor in the selection of surfaces by barnacle cyprids (Balanus amphitrite) during settlement . Biofouling , 26 : 287 – 299 .
- Alfaro , AC and Jeffs , AG . 2002 . Small-scale mussel settlement patterns within morphologically distinct substrata at Ninety Mile Beach, northern New Zealand . Malacologia , 44 : 1 – 15 .
- Anderson , C , Atlar , M , Callow , M , Candries , M , Milne , A and Townsin , RL . 2003 . The development of foul-release coatings for seagoing vessels . J Mar Des Operations , B4 : 11 – 23 .
- Apolinario , M and Coutinho , R . 2009 . “ Understanding the biofouling of offshore and deep-sea structures ” . In Advances in marine antifouling coatings and technologies , Edited by: Hellio , C and Yebra , D . 132 – 147 . Cambridge , , UK : Woodhead Publishing .
- Aprile , C , Corma , A and Garcia , H . 2008 . Enhancement of the photocatalytic activity of TiO2 through spatial structuring and particle size control: from subnanometric to submillimetric length scale . Phys Chem Chem Phys , 10 : 769 – 783 .
- Aruoja , V , Dubourguier , H-C , Kasemets , K and Kahru , A . 2009 . Toxicity of nanoparticles of CuO, ZnO and TiO2 to microalgae Pseudokirchneriella subcapitata . Sci Total Environ , 407 : 1461 – 1468 .
- ASTM . 2004 . Standard test method for Corona-treated polymer films using water contact angle measurements , Designation : D 5946–04 .
- Beigbeder , A , Jeusette , M , Mincheva , R , Claes , M , Brocorens , P , Lazzaroni , R and Dubois , P . 2009 . On the effect of carbon nanotubes on the wettability and surface morphology of hydrosilyation-curing silicone coatings . J Nanostr Polym Nanocomp , 5 : 37 – 43 .
- Beigbeder , A , Mincheva , R , Pettitt , ME , Callow , ME , Callow , JA , Claes , M and Dubois , P . 2010 . Marine fouling release silicone/carbon nanotube nanocomposite coatings: on the importance of the nanotube dispersion state . J Nanosci Nanotechno , 10 : 2972 – 2978 .
- Beigbeder , A , Degee , P , Conlan , SL , Mutton , RJ , Clare , AS , Pettitt , ME , Callow , ME , Callow , JA and Dubois , P . 2008 . Preparation and characterisation of silicone-based coatings filled with carbon nanotubes and natural sepiolite and their application as marine fouling-release coatings . Biofouling , 24 : 291 – 302 .
- Berntsson , KM , Jonsson , PR , Lejhall , M and Gatenholm , P . 2000 . Analysis of behavioural rejection of micro-textured surfaces and implications for recruitment by the barnacle Balanus improvisus . J Exp Mar Biol Ecol , 251 : 59 – 83 .
- Bhatkhande , DS , Pangarkar , VG and Beenackers , AACM . 2002 . Photocatalytic degradation for environmental applications – a review . J Chem Technol Biotechnol , 77 : 102 – 116 .
- Braithwaite , RA and McEvoy , LA . 2005 . Marine biofouling on fish farms and its remediation . Adv Mar Biol , 47 : 215 – 252 .
- Briand , JF . 2009 . Marine antifouling laboratory bioassays: an overview of their diversity . Biofouling , 25 : 297 – 311 .
- Buchanan , S and Babcock , R . 1997 . Primary and secondary settlement by the Greenshell mussel Perna canaliculus . J Shellfish Res , 16 : 71 – 76 .
- Callow , ME , Jennings , AR , Brennan , AB , Seegert , CE , Gibson , A , Wilson , L , Feinberg , A , Baney , R and Callow , JA . 2002 . Microtopographic cues for settlement of zoospores of the green fouling alga Enteromorpha . Biofouling , 18 : 237 – 245 .
- Carl , C , Poole , AJ , Vucko , MJ , Williams , MR , Whalan , S and de Nys , R . 2011 . Optimising settlement assays of pediveligers and plantigrades of Mytilus galloprovincialis . Biofouling , 27 : 859 – 868 .
- Carl , C , Polole , A , Sexton , B , Glenn , F , Vucko , M , Williams , M , Whalan , S and de Nys , R . 2012 . Enhancing the settlement and attachment of pediveligers of Mytilus galloprovincialis using surface wettability and microtopography . Biofouling , 28 : 175 – 186 .
- Carman , ML , Estes , TG , Feinberg , AW , Schumacher , JF , Wilkerson , W , Wilson , LH , Callow , ME , Callow , JA and Brennan , AB . 2006 . Engineered antifouling microtopographies – correlating wettability with cell attachment . Biofouling , 22 : 11 – 21 .
- Ci , L , Suhr , J , Pushparaj , V , Zhang , X and Ajayan , PM . 2008 . Continuous carbon nanotube reinforced composites . Nano Lett , 8 : 2762 – 2766 .
- Clarke , K and Gorley , R . 2006 . PRIMER v6: User manual/tutorial , 190 Plymouth , , UK : PRIMER-E .
- Conde , D , Aubriot , L and Sommaruga , R . 2000 . Changes in UV penetration associated with marine intrusions and freshwater discharge in a shallow coastal lagoon of the Southern Atlantic Ocean . Mar Ecol Prog Ser , 207 : 19 – 31 .
- Dabrunz , A , Duester , L , Prasse , C , Seitz , F , Rosenfeldt , R , Schilde , C , Schaumann , GE and Schulz , R . 2011 . Biological surface coating and molting inhibition as mechanisms of TiO2 nanoparticle toxicity in Daphnia magna . PLoS One , 6 : 20112
- de Nys , R and Guenther , J . 2009 . “ The impact and control of biofouling in marine finfish aquaculture ” . In Advances in marine antifouling coatings and technologies , Edited by: Hellio , C and Yebra , D . 177 – 247 . Cambridge , , UK : Woodhead Publishing .
- Dobretsov , S and Thomason , JC . 2011 . The development of marine biofilms on two commercial non-biocidal coatings: a comparison between silicone and fluoropolymer technologies . Biofouling , 27 : 869 – 880 .
- Edyvean , R . 2010 . “ Consequences of fouling on shipping ” . In Biofouling , Edited by: Dürr , S and Thomason , JC . 217 – 225 . Singapore : Wiley-Blackwell .
- Erlandsson , J , Pal , P and McQuaid , CD . 2006 . Re-colonisation rate differs between co-existing indigenous and invasive intertidal mussels following major disturbance . Mar Ecol Prog Ser , 320 : 169 – 176 .
- Filgueira , R , Peteiro , LG , Labarta , U and Fernández-Reiriz , MJ . 2007 . Assessment of spat collector ropes in Galician mussel farming . Aquac Eng , 37 : 195 – 201 .
- Finnie , AA and Williams , DN . 2010 . “ Paint and coatings technology for the control of marine fouling ” . In Biofouling , Edited by: Dürr , S and Thomason , JC . 185 – 206 . Singapore : Wiley-Blackwell .
- Fitridge , I , Dempster , T , Guenther , J and de Nys , R . 2012 . The impact and control of biofouling in marine aquaculture: a review . Biofouling , 28 : 649 – 669 .
- Flemming , H-C . 2010 . “ Why microorganism live in biofilms and the problem of biofouling ” . In Marine and industrial biofouling , Edited by: Flemming , H-C , Murthy , PS , Venkatesan , R and Cooksey , K . 3 – 12 . Berlin , , Germany : Springer .
- Frogley , M , Ravich , D and Wagner , HD . 2003 . Mechanical properties of carbon nanoparticle-reinforced elastomers . Compos Sci Technol , 63 : 1647 – 1654 .
- Fujishima , A and Zhang , X . 2006 . Titanium dioxide photocatalysis: present situation and future approaches . CR Chim , 9 : 750 – 760 .
- Fujishima , A , Zhang , X and Tryk , DA . 2008 . TiO2 photocatalysis and related surface phenomena . Surf Sci Rep , 63 : 515 – 582 .
- Gao , Y and Liu , H . 2003 . Preparation of TiO2/silicone rubber film by a fluidifying sedimentation method and its photocatalytic reactivities for the purification of water . J Mater Sci Lett , 22 : 1821 – 1823 .
- Gaya , UI and Abdullah , AH . 2008 . Heterogeneous photocatalytic degradation of organic contaminants over titanium dioxide: a review of fundamentals, progress and problems . J Photoch Photobio C , 9 : 1 – 12 .
- Gladis , F , Eggert , A , Karsten , U and Schumann , R . 2010 . Prevention of biofilm growth on man-made surfaces: evaluation of antialgal activity of two biocides and photocatalytic nanoparticles . Biofouling , 26 : 89 – 101 .
- Gosling , E . 2003 . Bivalve molluscs. Biology, ecology and culture , Oxford , , UK : Blackwell Science. 465 pp .
- Gribben , PE , Jeffs , AG , de Nys , R and Steinberg , PD . 2011 . Relative importance of natural cues and substrate morphology for settlement of the New Zealand Greenshell(™) mussel, Perna canaliculus . Aquaculture , 319 : 240 – 246 .
- Hajkova , P , Spatenka , P , Horsky , J , Horska , I and Kolouch , A . 2007 . Photocatalytic effect of TiO2 films on viruses and bacteria . Plasma Process Polym , 4 : S397 – S401 .
- Henderson , P . 2010 . “ Fouling and antifouling in other industries – power stations, desalination plants – drinking water supplies and sensors ” . In Biofouling , Edited by: Dürr , S and Thomason , JC . 288 – 305 . Singapore : Wiley-Blackwell .
- Ho , SH , Kwak , S-Y , Sohn , B-H and Park , TH . 2003 . Design of TiO2 nanoparticle self-assembled aromatic polyamide thin-film-composite (TFC) membrane as an approach to solve biofouling problem . J Membrane Sci , 211 : 157 – 165 .
- Holland , R , Dugdale , TM , Wetherbee , R , Brennan , AB , Finlay , JA , Callow , JA and Callow , ME . 2004 . Adhesion and motility of fouling diatoms on a silicone elastomer . Biofouling , 20 : 323 – 329 .
- Iketani , K , Sun , R-D , Toki , M , Hirota , K and Yamaguchi , O . 2003 . Sol–gel-derived TiO2/poly(dimethylsiloxane) hybrid films and their photocatalytic activities . J Phys Chem Solids , 64 : 507 – 513 .
- Kemmitt , T , Young , AG and Depree , C . 2012 . Observation of diatom settling and photocatalytic antifouling on TiO2 using ATR-IR spectroscopy . Mater Sci Forum , 700 : 227 – 230 .
- Kobak , J . 2001 . Light, gravity and conspecifics as cues to site selection and attachment behaviour of juvenile and adult Dreissena polymorpha Pallas, 1771 . J Mollus Stud , 67 : 183 – 189 .
- Lee , C , Hong , C , Kim , H , Kang , J and Zheng , HM . 2010 . TiO2 nanotubes as a therapeutic agent for cancer thermotherapy . Photochem Photobiol , 86 : 981 – 989 .
- Long , CJ , Finlay , JA , Callow , ME , Callow , JA and Brennan , AB . 2010 . Engineered antifouling microtopographies: mapping preferential and inhibitory microenvironments for zoospore attachment . Biofouling , 26 : 941 – 952 .
- Martinelli , E , Suffredini , M , Galli , G , Glisenti , A , Pettitt , ME , Callow , ME , Callow , JA , Williams , D and Lyall , G . 2011 . Amphiphilic block copolymer/poly(dimethylsiloxane) (PDMS) blends and nanocomposites for improved fouling-release . Biofouling , 27 : 529 – 541 .
- Miller , RJ , Bennett , S , Keller , AA , Pease , S and Lenihan , HS . 2012 . TiO2 nanoparticles are phototoxic to marine phytoplankton . PloS One , 7 : 30321
- Mirabedini , SM , Mohseni , M , PazokiFard , S and Esfandeh , M . 2008 . Effect of TiO2 on the mechanical and adhesion properties of RTV silicone elastomer coatings . Colloid Surface A , 317 : 80 – 86 .
- Molino , PJ , Campbell , E and Wetherbee , R . 2009 . Development of the initial diatom microfouling layer on antifouling and fouling-release surfaces in temperate and tropical Australia . Biofouling , 25 : 685 – 694 .
- Page , HM , Dugan , JE and Pilts , F . 2010 . “ Fouling and antifouling in oil and other offshore industries ” . In Biofouling , Edited by: Dürr , S and Thomason , JC . 252 – 266 . Singapore : Wiley-Blackwell .
- Paul , J , Sindhu , S , Nurmawati , MH and Valiyaveettil , S . 2006 . Mechanics of prestressed polydimethylsiloxane-carbon nanotube composite . Appl Phys Lett , 89 : 184101
- Pettersen , AK , Turchini , GM , Jahangard , S , Ingram , BA and Sherman , CDH . 2010 . Effects of different dietary microalgae on survival, growth, settlement and fatty acid composition of blue mussel (Mytilus galloprovincialis) larvae . Aquaculture , 309 : 115 – 124 .
- Sadhu , SD and Bhowmick , AK . 2008 . “ Elastomer-clay nanocomposites ” . In Current topics in elastomer research , Edited by: Bhowmick , AK . 23 – 56 . Boca-Raton , (FL : Taylor & Francis .
- Scardino , AJ and de Nys , R . 2011 . Mini review: Biomimetic models and bioinspired surfaces for fouling control . Biofouling , 27 : 73 – 86 .
- Scardino , AJ , Harvey , E and de Nys , R . 2006 . Testing attachment point theory: diatom attachment on microtextured polyimide biomimics . Biofouling , 22 : 55 – 60 .
- Scardino , AJ , Guenther , J and de Nys , R . 2008 . Attachment point theory revisited: the fouling response to a microtextured matrix . Biofouling , 24 : 45 – 53 .
- Schultz , MP , Bendick , JA , Holm , ER and Hertel , WM . 2011 . Economic impact of biofouling on a naval surface ship . Biofouling , 27 : 87 – 98 .
- Schumacher , JF , Aldred , N , Callow , ME , Finlay , JA , Callow , JA , Clare , AS and Brennan , AB . 2007a . Species-specific engineered antifouling topographies: correlations between the settlement of algal zoospores and barnacle cyprids . Biofouling , 23 : 307 – 317 .
- Schumacher , JF , Carman , ML , Estes , TG , Feinberg , AW , Wilson , LH , Callow , ME , Callow , JA , Finlay , JA and Brennan , AB . 2007b . Engineered antifouling microtopographies – effect of feature size, geometry, and roughness on settlement of zoospores of the green alga Ulva . Biofouling , 23 : 55 – 62 .
- Seed , R . 1976 . “ Ecology ” . In Marine mussels: their ecology and physiology , Edited by: Bayne , BL . 13 – 65 . Cambridge , , UK : Cambridge University Press .
- Silva , VP , Paschoalino , MP , Gonçalves , MC , Felisberti , MI , Jardim , WF and Yoshida , IVP . 2009 . Silicone rubbers filled with TiO2: characterization and photocatalytic activity . Mater Chem Phys , 113 : 395 – 400 .
- Thorstenson , ET , Ren , Z and Chou , T-W . 2001 . Advances in the science and technology of carbon nanotubes and their composites: a review . Compos Sci Technol , 61 : 1899 – 1912 .
- Tjong , SC . 2006 . Structural and mechanical properties of polymer nanocomposites . Mater Sci Eng R , 53 : 73 – 197 .
- Townsin , RL . 2003 . The ship hull fouling penalty . Biofouling , 19 : 9 – 15 .
- Townsin , RL and Anderson , CD . 2009 . “ Fouling control coatings using low surface energy, foul release technology ” . In Advances in marine antifouling coatings and technologies , Edited by: Hellio , C and Yebra , S . 693 – 708 . Cambridge , , UK : Woodhead Publishing .
- Vladkova , T . 2010 . “ Surface modification approach to control biofouling ” . In Marine and industrial biofouling , Edited by: Flemming , H-C , Murthy , PS , Venkatesan , R and Cooksey , K . 135 – 163 . Berlin , , Germany : Springer .
- Wang , S , Ang , HM and Tade , MO . 2007 . Volatile organic compounds in indoor environment and photocatalytic oxidation: state of the art . Environ Int , 33 : 694 – 705 .
- Webster , DC and Chisholm , BJ . 2010 . “ New directions in antifouling technology ” . In Biofouling , Edited by: Dürr , S and Thomason , JC . 366 – 387 . Singapore : Wiley-Blackwell .
- Zargiel , KA , Coogan , JS and Swain , GW . 2011 . Diatom community structure on commercially available ship hull coatings . Biofouling , 27 : 955 – 965 .