Abstract
Diatom biofilms are abundant in the marine environment. It is assumed (but untested) that extracellular polymeric substances (EPS), produced by diatoms, enable cells to cope with fluctuating salinity. To determine the protective role of EPS, Cylindrotheca closterium was grown in xanthan gum at salinities of 35, 50, 70 and 90 ppt. A xanthan matrix significantly increased cell viability (determined by SYTOX-Green), growth rate and population density by up to 300, 2,300 and 200%, respectively. Diatoms grown in 0.75% w/v xanthan, subjected to acute salinity shock treatments (at salinities 17.5, 50, 70 and 90 ppt) maintained photosynthetic capacity, Fq′/Fm′, within 4% of pre-shock values, whereas Fq′/Fm′ in cells grown without xanthan declined by up to 64% with hypersaline shock. Biofilms that developed in xanthan at standard salinity helped cells to maintain function during salinity shock. These results provide evidence of the benefits of living in an EPS matrix for biofilm diatoms.
Introduction
Microbial photosynthetic biofilms consist of prokaryotic and eukaryotic phototrophs embedded in a matrix of extracellular polymeric substances (EPS). Biofilms occur widely in almost all terrestrial and aquatic environments and provide important ecosystem functions, including sediment stabilisation (Paterson Citation1997; Lan et al. Citation2014), primary production (Underwood & Kromkamp Citation1999) and nutrient cycling (Nedwell et al. Citation1999), but also cause fouling on artificial maritime surfaces (eg Molino & Wetherbee Citation2008; Mieszkin et al. Citation2013). In many environments, such as intertidal sediment flats (Rothrock & Garcia-Pichel Citation2005; McKew et al. Citation2011), sea ice (Gleitz & Thomas Citation1993; Krembs et al. Citation2011), ships’ hulls, soil and desert biocrusts (Lan et al. Citation2014), salt marshes (Underwood Citation1997) and glacial surfaces (Yallop et al. Citation2012), biofilms are exposed to fluctuating (and in some cases, extreme) conditions of water availability and salinity (Gleitz & Thomas Citation1993; Underwood Citation1997; McKew et al. Citation2011). It has long been speculated that the ubiquity of EPS in biofilms is partly due to microorganisms modifying their immediate surroundings to reduce the negative impacts of water stress by changing the production and composition of their EPS (Decho Citation1990; Krembs & Deming Citation2008). This microscale environmental buffering by microbes has macroscale consequences (Decho Citation1994) as it allows the physical development and succession of primary producer assemblages in harsh environments, for example in large scale stabilisation of desert soils by cyanobacterial filaments and EPS (Lan et al. Citation2014), biostabilisation of marine sediment habitats (Yallop et al. Citation2000), and in the structure and organic carbon composition of polar sea ice (Krembs et al. Citation2011; Underwood et al. Citation2013).
Low or fluctuating water potentials are known to stimulate the production of EPS by bacteria (Dudman Citation1977; Roberson & Firestone Citation1992); the EPS provides a beneficial, hydrated microenvironment around the cell (Tonn & Gander Citation1979; Roberson & Firestone Citation1992; Chang & Halverson Citation2003). However, this process has received much less attention in microalgae. As microalgae, and in particular diatoms, produce EPS, an equivalence between the function of bacterial and microalgal EPS has long been suspected (Hostetter & Hoshaw Citation1970; Davis Citation1972).
Benthic diatoms associated with sea ice and intertidal sediment are well adapted to fluctuating conditions and can tolerate a wide range of salinities (Gleitz & Thomas Citation1993; Clavero et al. Citation2000; Krell et al. Citation2008). Some of these diatom species, eg Cylindrotheca closterium (Zargiel et al. Citation2011), are also known to adhere to artificial surfaces and cause biofoulingCitation. Diatoms can reduce the effects of salt stress by the active transport of ions out of the cell or into the vacuole (Shi et al. Citation2002) and by the regulation of cellular osmolytes (Clavero et al. Citation2000). However, these mechanisms are energetically expensive and can therefore halt or slow cell division (Krell et al. Citation2007). Hence, an EPS-based mechanism that buffers the effects of fluctuating water potential may provide a competitive advantage to diatoms, as it does for bacteria. For example, the benthic diatom Phaeodactylum tricornutum increases production of EPS and produces a higher proportion of uronic acids and sulphates at elevated salinities (Abdullahi et al. Citation2006), potentially allowing the EPS to retain more water. Nitzschia frustulum produces more rhamnose and xylose sugars under elevated salinity, causing the EPS gel to become thicker (Allan et al. Citation1972), which restricts the diffusion of anions towards the cell. The sea-ice diatom Fragilariopsis cylindrus increases EPS production at reduced temperatures and elevated salinity. The EPS inhibits ice formation and therefore acts as a cryoprotectant (Aslam et al. Citation2012). Although these adaptations support the concept that living within an EPS matrix is beneficial, there have been few, if any, direct tests of this hypothesis.
To further understand EPS protection of diatoms, a model system was used that allowed broad conclusions to be reached that are applicable to natural biofilms, which vary substantially in terms of species composition and microstructure. Cylindrotheca is a common maritime fouling genus and the species C. closterium has been reported in previous biofouling studies (Zargiel et al. Citation2011; Zargiel & Swain Citation2014). C. closterium is commonly used as a model species for studies of EPS (Staats et al. Citation1999; de Brouwer & Stal Citation2002) and previously caused a mucilage event in the Adriatic Sea (Najdek et al. Citation2005). It is common in mud flats and is known to modify its EPS production with changes in the environment (Alcoverro et al. Citation2000; Apoya-Horton et al. Citation2006).
The monosaccharide composition of diatom EPS varies between species but generally glucose, mannose, galactose and rhamnose are the most abundant sugars (Hoagland et al. Citation1993). C. closterium EPS consists of a highly hydrated matrix of strands with glucose and mannose as the dominant monosaccharides, which are acidified to uronic acids (Hoagland et al. Citation1993; Apoya-Horton et al. Citation2006), a composition also found in Navicula species (Bhosle et al. Citation1995; Staats et al. Citation1999). Specifically, C. closterium EPS fractions produced under a 12:12 h dark:light cycle contained 82.5% glucose and 7.6% mannose in the non-attached state (removed by centrifugation) (Staats et al. Citation1999) and in the attached state (extracted in 30°C water for 1 h), 22.9% glucose and 14.7% rhamnose. The prevalence of uronic acids was a common feature measured in the biofouling model species Craspedostauros australis and Amphora coffeaeformis (Poulsen et al. Citation2014). This common composition of diatom EPS is similar to xanthan gum, a polysaccharide gel synthesised by the bacterium Xanthomonas campestris and widely used as a reference standard for EPS in the marine environment (Passow & Alldredge Citation1995; Krembs et al. Citation2011) and for soil biofilms (Hart et al. Citation2001). Xanthan gum consists of glucose, mannose and glucuronic acid in the ratio 2:2:1 with terminal ends of pyruvate, which is thought to be important in cross-linking the xanthan molecules and so contributing structure to the gel. Pyruvate has also been detected, accounting for 20% of the biofilm EPS of the diatom Amphora rostrata (Khandeparker & Bhosle Citation2001). However, the high proportion of uronic acids in diatom EPS suggests that their molecular cross-linking is due to ionic interactions between divalent cations and the carboxylic group of the uronic acids, as occurs in bacterial biofilms (Sutherland Citation2001). The similarities between xanthan gum and C. closterium EPS make xanthan a useful tool for studying the protective qualities of EPS to cells.
This study investigated how the presence of an EPS matrix can influence the survival of C. closterium during growth at standard (35 ppt) and elevated salinities and during salinity fluctuations. For the first time experiments were explicitly designed that directly measured the effects of EPS on diatom cell viability and photosynthetic capacity, both of which govern population growth and persistence. The study examined whether increased EPS concentrations enhanced population growth and long term viability, and whether this effect was greater at higher salinity. The study also examined whether the presence of an EPS matrix protected cells from acute osmotic shock over short time periods.
Materials and methods
Culture conditions
Cylindrotheca closterium (Reimann et Lewin) from the University of Essex culture collection was grown in an artificial seawater base (35 ppt Reefsalt, AquaWorld, Swallow Aquatics, Southend-on-Sea, UK) enriched with f/2 medium (Guillard Citation1975). Cultures were maintained at salinity 35 ppt prior to the study and were not acclimated to the experimental conditions. Batch cultures were grown in a Qualicool 260 incubator (LTE Scientific Ltd, Oldham, UK) at 20°C and 24 h light at an irradiance of 12 ± 0.5 μmol photons m−2 s−1 provided by fluorescent lamps. Stock cultures were treated with gentamicin and penicillin–streptomycin solution (5 μg ml−l, Sigma-Aldrich, Dorset, UK), before experimental use, in order to minimise bacterial growth. Aseptic techniques were used throughout all procedures.
Long-term growth at elevated salinities
Cultures were grown in a 4 × 4 matrix of treatments: salinities of 35, 50, 70 and 90 (ppt/unitless) and xanthan gum (Sigma-Aldrich) at concentrations of 0, 0.04, 0.38 and 0.75% w/v. Salinity was increased from medium salinity (35 ppt) by adding NaCl. Xanthan gum was dissolved overnight into the growth medium and stock culture cells were added at starting population densities of 1.5 × 105 cells ml−1; determined by a haemacytometer count (Neubauer, improved bright-line). For treatments at salinities 35, 50 and 70 ppt, 12 sterile 5 ml Petri dishes (Sigma-Aldrich) were inoculated with 4 ml of the prepared cell culture and sealed with Parafilm (M laboratory film, Sigma-Aldrich) and incubated as above. For the treatments at salinity 90 ppt, 18 replicates were inoculated to allow additional sampling in the latter part of the study, due to the predicted suppression of growth rate and hence elongation of the growth cycle, at high salinity. In addition, C. closterium was maintained in the dark (20°C, n = 3) at salinities 35, 70 and 90 ppt with 0 or 0.75% xanthan gum (w/v) and measured for population density only, to establish whether heterotrophic utilisation of EPS components occurred (Tuchman et al. Citation2006).
On the day of inoculation (day 0) and every one to three days after, the maximum quantum efficiency of PSII photochemistry (Fv/Fm) was measured in a sub-sample (three dishes) of each treatment. The cultures were dark-adapted for 30 min before measurement. The minimum (Fo) and maximum (Fm) fluorescence yields were measured with a Xenon-PAM (pulse amplitude modulated) fluorometer (HeinzWalz GmbH, Effeltrich, Germany) as described by Waring et al. (Citation2006) with saturating light pulses (0.6 s) of ~ 4,000 μmol photons m−2 s−1 for measurement of Fm. The ratio Fv/Fm was calculated, where variable fluorescence Fv = Fm – Fo (Oxborough et al. Citation2000; Baker Citation2008).
On days 3, 8, 11 and 24 (also 29 and 37 for treatments at salinity 90 ppt) sub-samples of three dishes from each treatment were destructively sampled. Cells were counted using a haemacytometer. For the period of active growth, the specific growth rate, μ (day−1, Gotelli Citation1995), was determined for each treatment. The proportion of cells with compromised cell membranes (ie non-viable cells) was determined using the nucleic acid stain SYTOX-Green (Molecular Probes, S-34860, Life Technologies). SYTOX-Green was applied to a 1 ml sub-sample at a final concentration of 0.5 μM (Veldhuis et al. Citation2001) and incubated for 30 min in the dark, at culture temperature (conditions optimised prior to the study), before viewing cells via epifluorescence microscopy (Leitz, Ortholux II, Wetzlar, Germany). Total cells were counted under bright field, followed by SYTOX-Green stained cells under dark field. The proportion of live cells was calculated as population viability = (total no. of cells/no. of SYTOX-positive cells).
Short-term response to salinity fluctuations
Cultures of C. closterium with starting population densities of 6.7 × 105 cells ml−1, were grown in f/2 medium with 0 or 0.75% w/v xanthan gum (at standard seawater salinity of 35 ppt). Replicate (n = 5) cultures (1 ml) were grown in 5 ml wells (Sterilin, 25 well plates) sealed with Parafilm. After a growth period of 5 days, saline solution (1 ml, distilled water and NaCl) was applied to the top of the cultures. The applied solution was adjusted to produce final salinities within the cultures of 17.5, 35, 50, 70 and 90 ppt, giving a ‘salinity shock’.
The operating efficiency of PSII photochemistry (Fq′/Fm′) (Oxborough et al. Citation2000) was calculated before and after salt shock (at 15 s, 30 s, 1, 2, 4 and 6 min) to monitor changes in cell photosynthetic performance. Readings of F′ (steady state fluorescence measured under actinic light) and Fm′ (maximum fluorescence under actinic light) were taken at constant irradiance (5 ± 0.2 μmol photons m−2 s−2) 10 s before and 15 s, 30 s, 60 s, 2 min, 4 min and 6 min after treatment. The difference between Fm′ and F′, (Fq′ = Fm′ – F′) and the ratio Fq′/Fm′ were calculated. Maximum photosynthetic capacity (dark adapted Fv/Fm) was measured 24 h before, 1 h before and 24 h after the salt shock.
Statistical analysis
Sample distributions were tested for normality and equal variance using the Anderson–Darling test and F-test respectively, conducted using Minitab software. All sample distributions in this study were positive for both normality (p > 0.05) and equal variance (p > 0.05); further parametric testing was applied using SPSS software. A two way analysis of variance (2-way ANOVA) test was used to detect differences between treatments with a post hoc Tukey test applied subsequently to determine differences between factors. A one way ANOVA was used when comparing between more than two levels of one variable (eg between the proportions of live cells at all salinities within one xanthan concentration). The post hoc Holm–Sidak method was then used to make all comparisons between all factors.
Results
Growth and viability at elevated salinities in a model EPS matrix
The maximum population density of C. closterium, attained during the growth cycle (day 0 to day 37) was significantly higher in cultures containing xanthan gum (0.38 and 0.75%), compared to those grown with low or no xanthan gum (0.04 and 0%) (Figure ; 2-way ANOVA: F = 38.295, df = 3, p < 0.001, Holm–Sidak: p < 0.001). At salinities 35 and 50 ppt, growth in 0.38% and 0.75% xanthan gum more than doubled the maximum cell densities achieved compared to the controls and those in 0.04% xanthan gum (Figure ). At salinities 35 and 50 ppt, population growth rates were significantly increased in cultures with 0.75% xanthan gum, compared to the controls and those in 0.04% xanthan gum (Table ; ANOVA: F = 6.413, 61.055 with df = 3 and p = 0.05, Holm–Sidak: p < 0.05). Cultures incubated in dark conditions did not achieve any population growth (data not shown), hence the enhanced growth of cultures in high concentrations of xanthan gum was not due to heterotrophic utilisation of xanthan by the diatom cells.
Figure 1. C. closterium culture population densities (ml−1) in salinities of (A) 35, (B) 50, (C) 70 and (D) 90 ppt (mean and SE, n = 3). The growth medium contained xanthan gum concentrations of 0, 0.04, 0.38 and 0.75%.
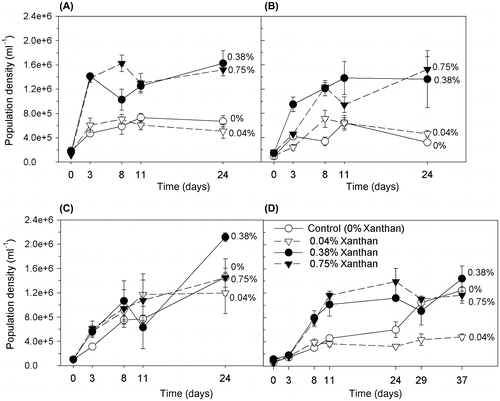
Figure 2. Proportion of live C. closterium cells (population viability) during growth in salinities of (A) 35, (B) 50, (C) 70 and (D) 90 ppt (mean and SE, n = 3). The growth medium contained xanthan gum at concentrations of 0, 0.04, 0.38 and 0.75%.
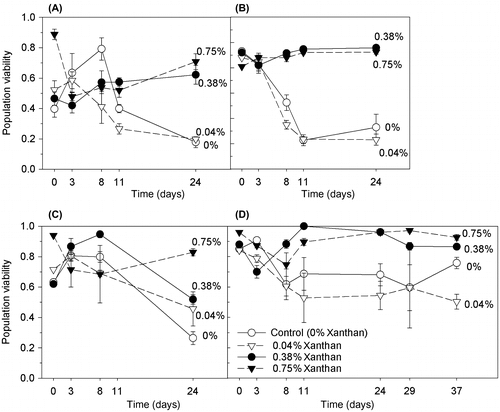
Table 1. Average specific growth rates, μ (d−1) for the period of active growth of C. closterium cultures (n = 3).
At the high salinities (70 and 90 ppt) the positive effect of xanthan gum (0.38 and 0.75%) on growth rate and maximum population density was not as conclusive as at the lower salinity (35 and 50 ppt) treatments. However, at salinity 90 ppt, growth rates were elevated in the 0.38% and 0.75% xanthan cultures, compared to those in 0.04% or no xanthan gum (Table ; ANOVA: F = 273 with df = 3 and p = <0.001, Holm–Sidak: p < 0.001. Cells at this highest experimental salinity (90 ppt) without xanthan gum had an extended lag phase of 24 days, followed by a period of growth (Figure D) while those in 0.04% xanthan gum did not undergo an active growth period. Cultures at salinity 90 ppt in high levels of xanthan gum (0.38 and 0.75%) had short three-day lag periods followed by active growth. All cultures at salinity 70 ppt achieved relatively high maximum cell densities (Figure ), compared with all other treatments, and all except those with 0.04% xanthan gum remained in active growth throughout the experimental period (Figure C) and growth rates were not significantly different between xanthan concentrations (Table ; ANOVA: F = 0.149 with df = 3 and p = 0.927).
C. closterium grown in 0.38 and 0.75% xanthan gum concentrations maintained higher levels of population viability after 24 days (0.81 ± 0.06, mean ± SE, n = 8), compared to cultures grown in low or no xanthan gum (0.36 ± 0.06, Figure , ANOVA; F = 22.8 with df = 3 and p < 0.001). The largest effect on survival occurred at salinity 50 ppt where population viability was increased when the medium contained 0.75% xanthan gum, compared to no xanthan gum, equating to the survival of 60% more of the total population. At salinities 35 and 70 ppt, population viabilities were more than tripled in cultures containing 0.75% xanthan gum compared to no xanthan gum, from 0.18 to 0.71 and from 0.26 to 0.83, respectively (Figure ). Differences were observed in the distributions of cells between xanthan treatments, regardless of salinity. C. closterium cells without xanthan gum grew as a lawn of cells on the bottom of the Petri dishes, spread out and attached. Cells in 0.38 and 0.75% xanthan gum grew in dispersed clumps suspended throughout the xanthan, suggesting that motility was curtailed, but not completely prevented, in this thickness of matrix.
Effect of EPS on the photosynthetic efficiency of cells exposed to long-term salt stress
The degree and direction of the effect of xanthan gum on the maximum potential photosynthetic efficiency of PSII (Fv/Fm), of the C. closterium cultures was dependent on the concentration of the xanthan gum and on the salinity of the medium. The presence of xanthan gum had a protective effect on Fv/Fm when cultures were grown in 0.75% xanthan gum, with Fv/Fm significantly increased in cultures grown at salinities 50 and 70 ppt (Figure ; ANOVA: F = 4.825, 3.594 with df = 3 and p = 0.05, Holm–Sidak: p < 0.009, 0.01). The presence of a low amount of xanthan gum (0.04%) had either a negative or negligible effect on Fv/Fm (Figure ). However at salinity 90 ppt, cultures grown in 0.38% xanthan gum had an increased Fv/Fm (ANOVA: F = 7.807 with df = 3 and p < 0.001, Holm–Sidak: p < critical level).
Protection of photosynthetic efficiency by xanthan gum during salinity fluctuations
Cultures grown in 0 and 0.75% xanthan gum were subjected to an acute salinity shock by the application of saline solutions. The cultures grown in xanthan gum maintained high Fq′/Fm′ (operating efficiency of PSII photochemistry) levels (Figure ); between 96 and 100% of their original values (Figure ) after the salinity shock was applied. In cultures grown in 0.75% xanthan gum a subtle decline in Fq′/Fm′ occurred between 2 and 6 min after application. However, across all treatments, Fq′/Fm′ did not fall below 97% of its original value (Figure ). By 24 h after treatment, cultures treated with solutions of 50, 70 and 90 ppt salinity had increased Fv/Fm (Figure A); the same response as cultures treated with the control solution at 35 ppt salinity (Figure B). This response was statistically significant at salinities 70 and 35 ppt only (t-test: t = –3.924, –3.881 with df = 6, 8 and p = 0.008, 0.005).
Figure 4. Fq′/Fm′ response of C. closterium cultures grown at salinity 35 ppt in 0 and 0.75% xanthan gum when salt shocked to salinities (A) 17.5, (B) 35, (C) 50, (D) 70 and (E) 90 ppt. Mean and SE bars shown (n = 5). = time of shock (0 min).
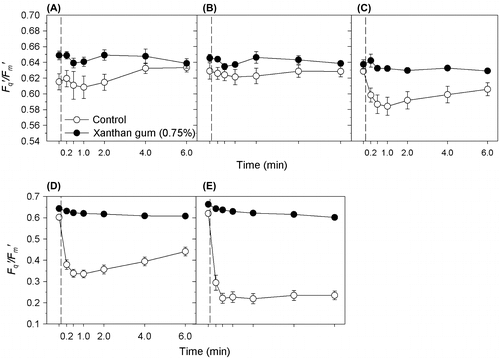
Figure 5. The initial percentage reduction in Fqʹ/Fmʹ in C. closterium cultures grown in 0% and 0.75% xanthan gum, 30 s after exposure to salinities of 17.5, 35, 50, 70 and 90 ppt (mean and SE, n = 5).
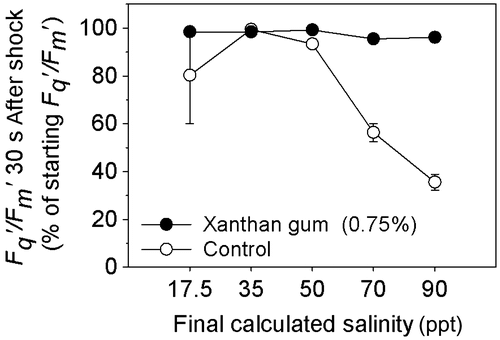
Figure 6. Fv/Fm of C. closterium cultures grown in (A) 0% and (B) 0.75% xanthan gum. Means and SE bars are shown for Fv/Fm before and 24 h after exposure to salt shock (of final salinities 17.5 to 90 ppt) (n = 5).
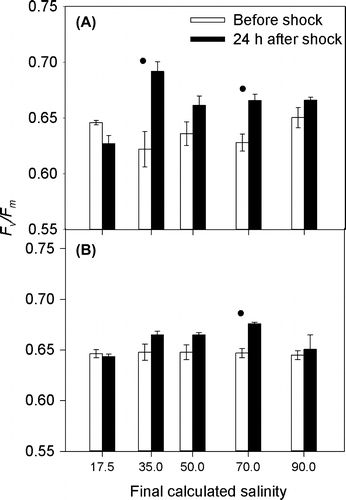
Cells grown in liquid medium (without xanthan gum), when exposed to a rapid change in salinity, suffered a steep decline in Fq′/Fm′ (Figure ) within 30 s of treatment. The level of decline in Fq′/Fm′ was dependant on the magnitude of the salinity change (Figure ), with the largest decrease observed after application of solution at salinity 90 ppt (65%). Recovery of Fq′/Fm′ was observed (Figure ), with recovery time increasing with the increasing salinity of the treatment (Figure ). By 24 h after treatment, the Fv/Fm of all cultures had returned to their original level (as before treatment), with the exception of salinity 70 ppt where Fv/Fm increased (Figure B). Therefore the maintenance of Fv/Fm was not dependent on growth in xanthan gum.
Discussion
Natural biofilms consist of a heterogeneous range of microbial taxa, chemical constituents and physical structures (Dobretsov et al. Citation2013; Mieszkin et al. Citation2013). The complexity present in natural biofilms provides challenges in determining the exact nature of cell–cell and cell–environment interactions, though broad scale patterns can be determined. Experimental studies using simplified systems have been used successfully to demonstrate species-specific positive and negative interactions between bacteria and diatoms, EPS production and concentrations, cell settlement and environmental conditions such as flow, carbon cycling and salinity conditions (Bruckner et al. Citation2011; Krembs et al. Citation2011; Aslam et al. Citation2012; Taylor et al. Citation2013; Zargiel & Swain Citation2014). The reductionist approach (a model system) was used in the present study to assess the role of EPS in protecting a biofilm-forming diatom during elevated and fluctuating salinities. EPS is a key characteristic of diatom-dominated biofilms (Underwood & Paterson Citation2003), and the results presented here demonstrate that the presence of an EPS matrix leads to a higher prevalence of viable cells (cells which have the capacity to divide in the future) in biofilms undergoing elevated and fluctuating salinities, such as would be experienced on ships’ hulls, in sea-ice and intertidal benthic environments. A natural biofilm contains an EPS matrix consisting of polymers from multiple sources (algal, bacterial, fungal, animal) and can be subject to various levels of both production and degradation by heterotrophic organisms (Dobretsov et al. Citation2013; Taylor et al. Citation2013). Such complexity is not possible to replicate under control conditions, but xanthan gum is widely used and accepted as an analogue for EPS in many studies (Passow & Alldredge Citation1995; Hart et al. Citation2001; Krembs et al. Citation2011; Aslam et al. Citation2012). Therefore EPS (represented by xanthan gum) appears to be an important adaptation which promotes population persistence for diatom biofilms, as has been previously demonstrated in bacterial biofilms.
Very few studies on diatom biofilm EPS production and composition also include measurements of the potential growth benefits of being in an EPS matrix. The experimental design used here aimed at removing as many other possible variables known to effect diatom physiology and EPS production, such as light–dark cycles and varying irradiance levels. This enabled the focus to be on the impacts of the EPS matrix on cell growth and viability. The growth rates and photosynthetic parameters of C. closterium reported here are very similar to those reported from a range of other studies (Smith & Underwood Citation2000; Staats et al. Citation2000; Underwood et al. Citation2004; Waring et al. Citation2006). This provides confidence in the design of the presented model system, and shows that there are benefits to cells of growing in an EPS matrix when challenged with salinity stress. However, the elevated growth rates observed with the addition of EPS at standard salinity in this study are in contrast to results from a sea-ice diatom, Fragilariopsis cylindrus, where growth rate was unaffected by addition of 0.1 and 0.5% xanthan gum at standard (34 ppt) and elevated (52 ppt) salinities (Aslam et al. Citation2012). However, for C. closterium at standard and elevated salinities, the growth rate was increased by the addition of xanthan gum at 0.38 and 0.75%, respectively. The two studies are not directly comparable, as in the Aslam et al. (Citation2012) study F. cylindrus cultures at salinities 34 and 52 ppt at 0°C had a significant pre-experimental acclimation period. Growth rates of F. cylindrus at salinities 34 and 52 ppt were 0.25 d−1 ± 0.03 and 0.13 d−1 ± 0.133 respectively (Aslam et al. Citation2012), which are comparable to growth rates of C. closterium at standard (35 ppt) salinity containing 0.38% xanthan gum and elevated (50 ppt) salinities without xanthan gum (0.2 d−1 ± 0.005 and 0.12 d−1 ± 0.03 respectively). Aslam et al. (Citation2012) found that addition of xanthan gum inhibited freezing of the medium during stepwise decreases in temperature from 0°C to –12°C. F. cylindrus at standard salinity without xanthan gum continued to grow at –4°C. However, growth was suppressed in cultures with 0.1% xanthan gum. F. cylindrus with 0.5% xanthan gum continued to grow at –4°C and freezing was avoided until the temperature was reduced to –12°C (Aslam et al. Citation2012). The differences in the level of ‘growth enhancement’ due to the presence of xanthan gum between these studies may therefore reflect an interplay between the presence of an EPS matrix, the salinity of the medium and ice formation in the low temperatures tested by Aslam et al. (Citation2012). There may also be species or habitat specific responses to living in xanthan gum. In general, sea-ice diatoms are more acclimated to changes in salinity due to seasonal changes with temperature (Ewert & Deming Citation2013). Aslam et al. (Citation2012) found that F. cylindrus produced proportionally higher amounts of complex EPS; hence such species may be pre-adapted to salinity changes.
Other studies on algal EPS have concentrated on factors affecting production (Wolfstein & Stal Citation2002) and analysis of structure (Mishra & Jha Citation2009). Hence the findings of elevated population viability and maximum population densities with the addition of xanthan, at standard salinity, provides the first evidence for benthic diatoms that being embedded in an EPS matrix is beneficial to growth regardless of salinity. The mechanistic basis of this benefit may be similar to the EPS capsule of bacteria, where nutrients and exoenzymes are concentrated close to the cell membrane (Tonn & Gander Citation1979), thus facilitating faster growth. However, at standard salinity the presence of xanthan gum at a low concentration of 0.04% had a detrimental effect on Fv/Fm, and at high salinity (90 ppt) it also had a detrimental effect on growth and viability. There may be a particular level of matrix density that is required for the physical benefits of being in a biofilm to manifest themselves. Below this threshold, at very low EPS concentrations, potential negative effects include reduction in nutrient diffusion to the cells, and the inhibition of movement. These concepts were not addressed in this study and require further investigation.
The beneficial effects of EPS were greater at the higher salinities tested in this study. However, the type and level of physiological benefit provided by xanthan (at 0.75 and 0.38%) varied with its salinity, with population density, growth rate and viability most enhanced at salinities 35 and 50 ppt, when Fv/Fm was also maintained. At salinity 70 ppt, Fv/Fm was enhanced by xanthan gum (at 0.75 and 0.38%). However, population density and viability data were inconclusive. Cultures at salinity 90 ppt had a similar growth curve, after a three-day lag period, as control cells (at salinity 35 ppt) providing xanthan was present at 0.75 or 0.38% but viability was increased with the presence of xanthan gum. Salinity of 75 ppt is a known upper limit for growth in many benthic diatom species (Clavero et al. Citation2000) and natural benthic microalgal diversity is known to decrease at salinities above 75 ppt (Herbst & Blinn Citation1998). Hence resource allocation was similar at salinities 35 and 50 ppt but a change in allocation occurred at salinities 70 and 90 ppt.
Ambient salinity is known to affect the quantity and composition of EPS in diatoms (Allan et al. Citation1972; Abdullahi et al. Citation2006; Apoya-Horton et al. Citation2006). When acclimated to elevated salinity (52 ppt) the sea-ice diatom F. cylindrus produced around three times more EPS than at standard salinity (34 ppt) (Aslam et al. Citation2012). C. closterium cells may have changed the composition of their EPS. This is a known response to hyper-salinity and an adaptation to resist freezing in brine channels during sea-ice formation when growth is limited (Krembs & Deming Citation2008), for example in Phaeodactylum tricornutum (Abdullahi et al. Citation2006) and Nitzschia frustulum (Allan et al. Citation1972). It was not possible to test this in the experiments presented due to the masking nature of the xanthan in the medium. EPS used for motility is also altered during hypo- and hyper-saline conditions in C. closterium (Apoya-Horton et al. Citation2006), which is known to affect diatom migration (Sauer et al. Citation2002).
Living in xanthan gum allowed the operating efficiency of PSII photochemistry (Fq′/Fm′) of C. closterium cells to be maintained during salinity shock; hence it is assumed that the photosynthetic apparatus was protected, most likely through forming a physical barrier around cells. Living in an EPS matrix when the external environment is undergoing salinity changes will slow the permeation of salt and osmotic stress to embedded cells. The mechanical action of forming a physical diffusion barrier is a known function of EPS in sea ice, where brine channels are ‘plugged’ by the EPS (Krembs & Deming Citation2008). If that change in salinity is transient then cells within the gel will not have used energy acclimating to a changing external salinity environment. However, if the altered salinity is permanent the gel would mediate the penetration of ions, so reducing osmotic stress to the cells and thereby the amount of energy used in protective mechanisms. In addition, an EPS matrix may collect and concentrate compatible solutes close to the cells which would help to retain water activity, stabilise macromolecules and aid osmoregulation (Yancey et al. Citation1982), allowing the maintenance of cellular function during salinity shocks. Under hypo-saline conditions, diatoms expel compatible solutes into the environment, which are retained by the EPS matrix and can be re-imported during hyper-saline conditions, thus avoiding the metabolic cost of synthesis. For example in hypo-saline conditions, C. closterium rapidly excretes dimethylsulfonioproprionate (DMSP) (Nilsson & Sundbäck Citation1996; Van Bergeijk et al. Citation2003) and amino acids (Admiraal et al. Citation1984) into the surrounding EPS. A possible mechanism for the release and import of compatible solutes is a change in the selective permeability of the cytoplasmic membrane caused by an altered environmental salinity, with the level of permeability depending on the magnitude of the salinity change (Schobert Citation1980). Regulation of compatible solutes by export and import is thought to be a mechanism of short term osmo-acclimation for diatoms living in the highly fluctuating salinities of intertidal sediments (Van Bergeijk et al. Citation2003).
The results presented also demonstrate that the development of a diatom biofilm under normal growth conditions can help cells to maintain photosynthetic function when subjected to salinity shocks such as those found in intertidal sediments during sea ice development, permanent structures in tidal estuaries, and in maritime shipping. It is also highly likely that other organisms (eg bacteria, fungi and macroalgal sporelings; Dobretsov et al. Citation2013; Mieszkin et al. Citation2013) living within photosynthethic biofilms benefit from the EPS matrix predominantly produced by diatoms. Of particular interest is the idea that cell lysis and local decomposition of the matrix creates pores allowing better nutrient transport to the cells (Webb et al. Citation2003). Aggregates of dead cells were observed in medium containing xanthan gum, indicating mechanistic control of cell death. A relationship between cell aggregation and population viability has also been reported in the diatom Thalassiosira weissflogii; aggregation prolonged high population viabilities in dark, nutrient-limited conditions (Garvey et al. Citation2007). Quorum sensing, a cell–cell communication mechanism used by Gram-negative bacteria is known to help regulate certain components of EPS (De Kievit Citation2009; Borlee et al. Citation2010) and an adaptive role for diatom cell death could potentially be investigated in the C. closterium model EPS system developed here.
In conclusion, this study has demonstrated for the first time that the presence of EPS can increase the viability, maximum population density and growth rate of diatom populations in biofilms in standard and elevated salinities. C. closterium is a cosmopolitan diatom species, widely distributed in biofilms on natural silts, sand, rocks, sea ice, biotic and artificial substrata and has a similar photophysiology and EPS physiology to many of the other widespread biofilm-forming diatom genera, eg Achnanthes, Nitzschia and Navicula (Underwood & Paterson Citation2003; Chiovitti et al. Citation2004; Bellinger et al. Citation2005; Apoya-Horton et al. Citation2006). These taxonomic similarities, and the similarity in the emergent properties of mature biofilms (resilience, intense microbial coupling, and productivity), despite both structural and taxonomic diversity (Decho Citation1990; Dobretsov et al. Citation2013; Mieszkin et al. Citation2013) suggest that the beneficial properties of being embedded in an EPS matrix for cell growth, viability and photosynthesis described here may be applicable to marine photosynthetic biofilms in general.
Acknowledgements
This work was partly supported by the UK Natural Environment Research Council (NE/E016251/1).
References
- Abdullahi AS, Underwood GJC, Gretz MR. 2006. Extracellular matrix assembly in diatoms (Bacillariophyceae). V. Environmental effects on polysaccharide synthesis in the model diatom, Phaeodactylum tricornutum. J Phycol. 42:363–378.10.1111/jpy.2006.42.issue-2
- Admiraal W, Laane R, Peletier H. 1984. Participation of diatoms in the amino acid cycle of coastal waters; uptake and excretion in cultures. Mar Ecol Prog Ser. 15:303–306.10.3354/meps015303
- Alcoverro T, Conte E, Mazzella L. 2000. Production of mucilage by the Adriatic epipelic diatom Cylindrotheca closterium (Bacillariophyceae) under nutrient limitation. J Phycol. 36:1087–1095.10.1046/j.1529-8817.2000.99193.x
- Allan GG, Lewin J, Johnson PG. 1972. Marine polymers. IV Diatom polysaccharides. Bot Mar. 15:102–108.
- Apoya-Horton MD, Yin L, Underwood GJC, Gretz MR. 2006. Movement modalities and responses to environmental changes of the mudflat diatom Cylindrotheca closterium (Bacillariophyceae). J Phycol. 42:379–390.10.1111/jpy.2006.42.issue-2
- Aslam SN, Cresswell-Maynard T, Thomas DN, Underwood GJC. 2012. Production and characterization of the intra- and extracellular carbohydrates and polymeric substances (EPS) of three sea-ice diatom species, and evidence for a cryoprotective role for EPS. J Phycol. 48:1494–1509.10.1111/jpy.12004
- Baker NR. 2008. Chlorophyll fluorescence: a probe of photosynthesis in vivo. Annu Rev Plant Biol. 59:89–113.10.1146/annurev.arplant.59.032607.092759
- Bellinger BJ, Abdullahi AS, Gretz MR, Underwood GJC. 2005. Biofilm polymers: relationship between carbohydrate biopolymers from estuarine mudflats and unialgal cultures of benthic diatoms. Aquat Microb Ecol. 38:169–180.10.3354/ame038169
- Bhosle NB, Sawant SS, Garg A, Wagh AB. 1995. Isolation and partial chemical analysis of exopolysaccharides from the marine fouling diatom Navicula subinflata. Bot Mar. 38:103–110.
- Borlee BR, Goldman AD, Murakami K, Samudrala R, Wozniak DJ, Parsek MR. 2010. Pseudomonas aeruginosa uses a cyclic-di-GMP-regulated adhesin to reinforce the biofilm extracellular matrix. Mol Microbiol. 75:827–842.10.1111/mmi.2010.75.issue-4
- Bruckner CG, Rehm C, Grossart H-P, Kroth PG. 2011. Growth and release of extracellular organic compounds by benthic diatoms depend on interactions with bacteria. Environ Microbiol. 13:1052–1063.10.1111/emi.2011.13.issue-4
- Chang WS, Halverson LJ. 2003. Reduced water availability influences the dynamics, development, and ultrastructural properties of Pseudomonas putida biofilms. J Bacteriol. 185:6199–6204.10.1128/JB.185.20.6199-6204.2003
- Chiovitti A, Molino P, Crawford SA, Teng R, Spurck T, Wetherbee R. 2004. The glucans extracted with warm water from diatoms are mainly derived from intracellular chrysolaminaran and not extracellular polysaccharides. Eur J Phycol. 39:117–128.10.1080/0967026042000201885
- Clavero E, Hernandez-Marine M, Grimalt JO, Garcia-Pichel F. 2000. Salinity tolerance of diatoms from thalassic hypersaline environments. J Phycol. 36:1021–1034.10.1046/j.1529-8817.2000.99177.x
- Davis JS. 1972. Survival records in the algae, and the survival role of certain algal pigments, fat and mucilaginous substances. Biologist. 54:52–93.
- de Brouwer JFC, Stal LJ. 2002. Daily fluctuations of exopolymers in cultures of the benthic diatoms Cylindrotheca closterium and Nitzschia sp. (Bacillariophyceae). J Phycol. 38:464–472.10.1046/j.1529-8817.2002.01164.x
- De Kievit TR. 2009. Quorum sensing in Pseudomonas aeruginosa biofilms. Environ Microbiol. 11:279–288.10.1111/emi.2009.11.issue-2
- Decho A. 1994. Molecular-scale events influencing the macroscale cohesiveness of exopolymers. In: Krumbein WE, Paterson DM, Stal LJ, editors. Biostabilization of sediment. BIS Verlag: Oldenburg; p. 135–148.
- Decho AW. 1990. Microbial exopolymer secretions in ocean environments – their role (s) in food webs and marine processes. Oceanogr Mar Biol. 28:73–153.
- Dobretsov S, Abed RM, Teplitski M. 2013. Mini-review: Inhibition of biofouling by marine microorganisms. Biofouling. 29:423–441.10.1080/08927014.2013.776042
- Dudman WF. 1977. The role of surface polysaccharides in natural environments. In: Sutherland IW, editor. Surface carbohydrates of the prokaryotic cell. London: Accademic Press; p. 357–414.
- Ewert M, Deming JW. 2013. Sea ice microorganisms: environmental constraints and extracellular responses. Biology. 2:603–628.10.3390/biology2020603
- Garvey M, Moriceau B, Passow U. 2007. Applicability of the FDA assay to determine the viability of marine phytoplankton under different environmental conditions. Mar Ecol Prog Ser. 352:17–26.10.3354/meps07134
- Gleitz M, Thomas DN. 1993. Variation in phytoplankton standing stock, chemical composition and physiology during sea-ice formation in the southeastern Weddell Sea, Antarctica. J Exp Mar Biol Ecol. 173:211–230.10.1016/0022-0981(93)90054-R
- Gotelli NJ. 1995. A primer of ecology. Sunderland (MA): Sinauer Associates.
- Guillard RRL. 1975. Culture of phytoplankton for feeding marine invertebrates. In: Smith WL, Chanley MH, editors. Culture of marine invertebrate animals. New York (NY): Plenum Press; p. 26–60.
- Hart T, Lynch J, Chamberlain A. 2001. Anion exclusion in microbial and soil polysaccharides. Biol Fertility Soils. 34:201–209.10.1007/s003740100400
- Herbst DB, Blinn DW. 1998. Experimental mesocosm studies of salinity effects on the benthic algal community of a saline lake. J Phycol. 34:772–778.10.1046/j.1529-8817.1998.340772.x
- Hoagland KD, Rosowski JR, Gretz MR, Roemer SC. 1993. Diatom extracellular polymeric substances: function, fine structure, chemistry, and physiology. J Phycol. 29:537–566.10.1111/jpy.1993.29.issue-5
- Hostetter HP, Hoshaw RW. 1970. Environmental factors affecting resistance to desiccation in the diatom Stauroneis anceps. Am J Bot. 57:512–518.10.2307/2441048
- Khandeparker RDS, Bhosle NB. 2001. Extracellular polymeric substances of the marine fouling diatom Amphora rostrata Wm. Sm. Biofouling. 17:117–127.10.1080/08927010109378471
- Krell A, Beszteri B, Dieckmann G, Glöckner G, Valentin K, Mock T. 2008. A new class of ice-binding proteins discovered in a salt-stress-induced cDNA library of the psychrophilic diatom Fragilariopsis cylindrus (Bacillariophyceae). Eur J Phycol. 43:423–433.10.1080/09670260802348615
- Krell A, Funck D, Plettner I, John U, Dieckmann G. 2007. Regulation of proline metabolism under salt stress in the psychrophilic diatom Fragilariopsis cylindrus (Bacillariophyceae). J Phycol. 43:753–762.10.1111/jpy.2007.43.issue-4
- Krembs C, Deming J. 2008. The role of exopolymers in microbial adaptation to sea ice. In: Margesin R, Schinner F, Marx J-C, Gerday C, editors. Psychrophiles: from biodiversity to biotechnology. Berlin: Springer-Verlag; p. 247–264.
- Krembs C, Eicken H, Deming JW. 2011. Exopolymer alteration of physical properties of sea ice and implications for ice habitability and biogeochemistry in a warmer Arctic. P Natl Acad Sci USA. 108:3653–3658.10.1073/pnas.1100701108
- Lan S, Zhang Q, Wu L, Liu Y, Zhang D, Hu C. 2014. Artificially accelerating the reversal of desertification: cyanobacterial inoculation facilitates the succession of vegetation communities. Environ Sci Technol. 48:307–315.10.1021/es403785j
- McKew BA, Taylor JD, McGenity TJ, Underwood GJC. 2011. Resilience of benthic biofilm communities from a temperate salt marsh to desiccation and rewetting. ISME J. 5:30–41.10.1038/ismej.2010.91
- Mieszkin S, Callow ME, Callow JA. 2013. Interactions between microbial biofilms and marine fouling algae: a mini review. Biofouling. 29:1097–1113.10.1080/08927014.2013.828712
- Mishra A, Jha B. 2009. Isolation and characterization of extracellular polymeric substances from micro-algae Dunaliella salina under salt stress. Bioresour Technol. 100:3382–3386.10.1016/j.biortech.2009.02.006
- Molino PJ, Wetherbee R. 2008. The biology of biofouling diatoms and their role in the development of microbial slimes. Biofouling. 24:365–379.10.1080/08927010802254583
- Najdek M, Blazina M, Djakovac T, Kraus R. 2005. The role of the diatom Cylindrotheca closterium in a mucilage event in the northern Adriatic Sea: coupling with high salinity water intrusions. J Plankton Res. 27:851–862.10.1093/plankt/fbi057
- Nedwell DB, Jickells TD, Trimmer M, Sanders R. 1999. Nutrients in estuaries. Adv Ecol Res. 29:43–92.10.1016/S0065-2504(08)60191-9
- Nilsson C, Sundbäck K. 1996. Amino acid uptake in natural microphytobenthic assemblages studied by microautoradiography. Hydrobiologia. 332:119–129.10.1007/BF00016691
- Oxborough K, Hanlon ARM, Underwood GJC, Baker NR. 2000. In vivo estimation of the photosystem II photochemical efficiency of individual microphytobenthic cells using high-resolution imaging of chlorophyll a fluorescence. Limnol Oceanogr. 45:1420–1425.10.4319/lo.2000.45.6.1420
- Passow U, Alldredge AL. 1995. A dye-binding assay for the spectrophotometric measurement of transparent exopolymer particles (TEP). Limnol Oceanogr. 40:1326–1335.10.4319/lo.1995.40.7.1326
- Paterson D. 1997. Biological mediation of sediment erodibility: ecology and physical dynamics. In: Burt N, Parker R, Watts J, editors. Cohesive sediments. Chichester: Wiley; p. 215–229.
- Poulsen N, Kröger N, Harrington MJ, Brunner E, Paasch S, Buhmann MT. 2014. Isolation and biochemical characterization of underwater adhesives from diatoms. Biofouling. 30:513–523.10.1080/08927014.2014.895895
- Roberson EB, Firestone MK. 1992. Relationship between desiccation and exopolysaccharide production in a soil Pseudomonas sp. Appl Environ Microbiol. 58:1284–1291.
- Rothrock MJ, Garcia-Pichel F. 2005. Microbial diversity of benthic mats along a tidal desiccation gradient. Environ Microbiol. 7:593–601.10.1111/emi.2005.7.issue-4
- Sauer J, Wenderoth K, Maier UG, Rhiel E. 2002. Effects of salinity, light and time on the vertical migration of diatom assemblages. Diatom Res. 17:189–203.10.1080/0269249X.2002.9705538
- Schobert B. 1980. Proline catabolism, relaxation of osmotic strain and membrane permeability in the diatom Phaeodactylum tricornutum. Physiol Plant. 50:37–42.10.1111/ppl.1980.50.issue-1
- Shi H, Lee B, Wu SJ, Zhu JK. 2002. Overexpression of a plasma membrane Na+/H+ antiporter gene improves salt tolerance in Arabidopsis thaliana. Nat Biotechnol. 21:81–85.10.1038/nbt766
- Smith DJ, Underwood GJ. 2000. The production of extracellular carbohydrates by estuarine benthic diatoms: the effects of growth phase and light and dark treatment. J Phycol. 36:321–333.
- Staats N, De Winder B, Stal LJ, Mur LR. 1999. Isolation and characterization of extracellular polysaccharides from the epipelic diatoms Cylindrotheca closterium and Navicula salinarum. Eur J Phycol. 34:161–169.10.1080/09670269910001736212
- Staats N, Stal LJ, Mur LR. 2000. Exopolysaccharide production by the epipelic diatom Cylindrotheca closterium: effects of nutrient conditions. J Exp Mar Biol Ecol. 249:13–27.10.1016/S0022-0981(00)00166-0
- Sutherland IW. 2001. Biofilm exopolysaccharides: a strong and sticky framework. Microbiology. 147:3–9.
- Taylor JD, McKew BA, Kuhl A, McGenity TJ, Underwood GJ. 2013. Microphytobenthic extracellular polymeric substances (EPS) in intertidal sediments fuel both generalist and specialist EPS-degrading bacteria. Limnol Oceanogr. 58:1463–1480.
- Tonn SJ, Gander JE. 1979. Biosynthesis of polysaccharides by prokaryotes. Annu Rev Microbiol. 33:169–199.10.1146/annurev.mi.33.100179.001125
- Tuchman NC, Schollett MA, Rier ST, Geddes P. 2006. Differential heterotrophic utilization of organic compounds by diatoms and bacteria under light and dark conditions. Hydrobiologia. 561:167–177.
- Underwood G, Kromkamp J. 1999. Primary production by phytoplankton and microphytobenthos in estuaries. Adv Ecol Res. 29:93–153.10.1016/S0065-2504(08)60192-0
- Underwood GJC. 1997. Microalgal colonization in a saltmarsh restoration scheme. Estuar Coast Shelf Sci. 44:471–481.10.1006/ecss.1996.0138
- Underwood GJC, Aslam SN, Michel C, Niemi A, Norman L, Meiners KM, Laybourn-Parry J, Paterson H, Thomas DN. 2013. Broad-scale predictability of carbohydrates and exopolymers in Antarctic and Arctic sea ice. P Natl Acad Sci USA. 110:15734–15739.10.1073/pnas.1302870110
- Underwood GJC, Boulcott M, Raines CA, Waldron K. 2004. Environmental effects on exopolymer production by marine benthic diatoms: dynamics, changes in composition and pathways of production. J Phycol. 40:293–304.10.1111/j.1529-8817.2004.03076.x
- Underwood GJC, Paterson DM. 2003. The importance of extracellular carbohydrate production by marine epipelic diatoms. Adv Bot Res. 40:184–241.
- Van Bergeijk SA, Van der Zee C, Stal LJ. 2003. Uptake and excretion of dimethylsulphoniopropionate is driven by salinity changes in the marine benthic diatom Cylindrotheca closterium. Eur J Phycol. 38:341–349.10.1080/09670260310001612600
- Veldhuis MJW, Kraay GW, Timmermans KR. 2001. Cell death in phytoplankton: correlation between changes in membrane permeability, photosynthetic activity, pigmentation and growth. Eur J Phycol. 36:167–177.10.1080/09670260110001735318
- Waring J, Underwood GJC, Baker NR. 2006. Impact of elevated UV-B radiation on photosynthetic electron transport, primary productivity and carbon allocation in estuarine epipelic diatoms. Plant Cell Environ. 29:521–534.10.1111/pce.2006.29.issue-4
- Webb JS, Thompson LS, James S, Charlton T, Tolker-Nielsen T, Koch B, Givskov M, Kjelleberg S. 2003. Cell death in Pseudomonas aeruginosa biofilm development. J Bacteriol. 185:4585–4592.10.1128/JB.185.15.4585-4592.2003
- Wolfstein K, Stal LJ. 2002. Production of extracellular polymeric substances (EPS) by benthic diatoms: effect of irradiance and temperature. Mar Ecol Prog Ser. 236:13–22.10.3354/meps236013
- Yallop ML, Anesio AM, Perkins RG, Cook J, Telling J, Fagan D, MacFarlane J, Stibal M, Barker G, Bellas C. 2012. Photophysiology and albedo-changing potential of the ice algal community on the surface of the Greenland ice sheet. ISME J. 6:2302–2313.10.1038/ismej.2012.107
- Yallop ML, Paterson DM, Wellsbury P. 2000. Interrelationships between rates of microbial production, exopolymer production, microbial biomass, and sediment stability in biofilms of intertidal sediments. Microb Ecol. 39:116–127.10.1007/s002489900186
- Yancey PH, Clark ME, Hand SC, Bowlus RD, Somero GN. 1982. Living with water stress: evolution of osmolyte systems. Science. 217:1214–1222.10.1126/science.7112124
- Zargiel KA, Coogan JS, Swain GW. 2011. Diatom community structure on commercially available ship hull coatings. Biofouling. 27:955–965.10.1080/08927014.2011.618268
- Zargiel KA, Swain GW. 2014. Static vs dynamic settlement and adhesion of diatoms to ship hull coatings. Biofouling. 30:115–129.10.1080/08927014.2013.847927