Abstract
Butenolide is an environmentally friendly antifouling natural product, but its efficiency and mechanism in preventing biofilm formation have not been examined. Furthermore, controlling the release of butenolide from paints into seawater is technically challenging. A coating was developed by mixing butenolide with a biodegradable polymer, poly (ε-caprolactone)-based polyurethane, and a one-month in situ anti-biofilm test was conducted in a subtidal area. The constant release of butenolide from the surface suggested that its release was well controlled. Direct observation and confocal microscope investigation indicated that the coating was effective against both biofilm formation and attachment of large fouling organisms. Metatranscriptomic analysis of biofilm samples implied that the coating selectively inhibited the adhesion of microbes from a variety of phyla and targeted particular functional pathways including energy metabolism, drug transport and toxin release. These integrated analyses demonstrated the potential application of this butenolide/polymer coating as an anti-biofilm material.
Abbreviations:
- butenolide, 5-octylfuran-2(5H)-one
- DCOIT, 4,5-dichloro-2-n-octyl-4-isothiazolin-3-one
- DBTDL, dibutyltin dilaurate
- HPLC, high performance liquid chromatography
- CLSM, confocal laser scanning microscopy
- FITC, fluorescein isothiocyanate
- COGs, Clusters of Orthologous Groups
- PCA, principal component analysis
- ABC transporters, ATP-binding cassette transporters
- RTX, repeats-in-toxin
Introduction
In marine environments, biofouling refers to the formation of biofilms and the settlement of macrofouling species (such as molluscs, encrusting bryozoans and barnacles) on man-made surfaces (Bhosle et al. Citation1994; Lau et al. Citation2002; Dobretsov et al. Citation2006; Chen et al. Citation2013). Biofilms form when multiple species of bacteria, archaea and diatoms adhere to surfaces immersed in aqueous environments and secrete glue-like substances that can anchor the microbial cells. Biofilm formation, which also causes corrosion of materials, is one of the major sources of marine fouling (Flemming Citation1997). Moreover, there is evidence that the chemical and physical signals released by microbial biofilms may induce the larvae of macrofoulers to settle (Unabia and Hadfield Citation1999; Holm et al. Citation2000; Huang and Hadfield Citation2003). Therefore, the development of anti-biofilm coatings is a promising way to prevent marine biofouling.
Marine natural products provide a promising reservoir of useful compounds with many marine chemical molecules being reported as potential anti-biofilm agents (reviewed in Clare Citation1996; Fusetani Citation2004, Citation2011; Qian et al. Citation2009, Citation2015). For example, Salta et al. (Citation2013) identified toluene from Chondrus crispus, which prevents biofilm formation by the species Cobetia marina and Marinobacter hydrocarbonoclasticus. However, due to technical challenges, such as in situ control of the release rate of these compounds, few compounds have been developed into anti-biofilm agents in marine coatings. One promising solution to the release problem is to utilize polymers with a degradable backbone, which can generate a self-renewing surface in the marine environment and serve as the carrier of anti-biofilm compounds (Ma et al. Citation2013; Sisson et al. Citation2013; Babu et al. Citation2016).
The ecologically benign antifoulant, 5-octylfuran-2(5H)-one (butenolide) was reported previously (Xu et al. Citation2010). Butenolide prevents the settlement of major macrofoulers, such as barnacles and bryozoans, and has very low toxicity in its target organisms as indicated by the high pharmaceutical ratios (LC50/EC50) (Xu et al. Citation2010). Furthermore, the degradation kinetics of butenolide under various environmental conditions has shown it is rapidly degraded in natural seawater (Chen et al. Citation2015). Despite the accumulating evidence of the potential application for butenolide as a new antifouling (AF) agent, the effect of butenolide on biofilm formation has not been examined, especially with respect to release and performance in in situ marine environments. In the present study, a self-renewing system using the biodegradable polymer poly (ε-caprolactone)-based polyurethane was constructed to control the release of butenolide and a one-month in situ test was performed to assess its anti-biofilm performance. In parallel, the commercial anti-biofilm agent 4,5-dichloro-2-n-octyl-4-isothiazolin-3-one (DCOIT) was used for comparison. Metatranscriptomic analysis was integrated with the anti-biofilm behavior of butenolide to explore the underlying molecular mechanisms of action.
Materials and methods
Chemicals
Butenolide was synthesized by Shanghai Medicilon Inc. (Shanghai, China). The structure is shown in Figure . DCOIT was obtained from The Dow Chemical Company (Midland, MI, USA). The molar masses and dispersity of the poly (ε-caprolactone)-based polyurethane used in the present study were 27,000 and 1.87, respectively. Poly (ε-caprolactone)-based polyurethane was prepared by polyaddition according to the steps described in a previous study (Ma et al. Citation2013), and a general experimental procedure was as follows: first, isophorone diisocyanate was allowed to react with poly (ε-caprolactone) diol at 70°C for 1 h in tetrahydrofuran under a nitrogen atmosphere, yielding a prepolymer. Subsequently, 1,4-butanediol and dibutyltin dilaurate (DBTDL) were added as the chain extender and catalyst, respectively, and the mixture was allowed to react at 80°C for 3 h. The product was precipitated into hexane twice, filtered, and dried under vacuum at 40°C for 24 h.
Coating preparation
The butenolide/polymer coating was prepared by mixing solutions. Typically, for the 5 wt% butenolide coating, poly (ε-caprolactone)-based polyurethane (0.95 g) and butenolide (0.05 g) were dissolved by vigorous stirring in xylene at 25°C. Then the solution was coated onto glass fiber reinforced epoxy resin panels (20 × 70 mm2) to form a film. Other coatings with different concentrations of butenolide (1 wt%, 2.5 wt% and 10 wt%) or DCOIT (5 wt%) were prepared following the same procedure. To make a control panel, poly (ε-caprolactone)-based polyurethane (1 g) was dissolved in xylene and coated onto the resin described above. After removal of volatile organic compounds by exposure at room temperature and atmospheric pressure for seven days, the panels were used for anti-biofilm assessment. The dry film thickness of each coating was 400 μm.
Release rate measurement
Butenolide concentrations were measured to determine its release rates by high performance liquid chromatography (HPLC) under static conditions. Typically, the panel coated with butenolide and polymer was immersed in a measuring container with 100 ml of sterilized artificial seawater. After 24 h immersion, 10 ml of seawater was taken from the measuring container and extracted three times using 10 ml of dichloromethane. After drying under nitrogen gas, the extract was resuspended in 100 μl of methanol and then subjected to HPLC analysis using a reversed-phase system (Waters 2695, Milford, MA, USA) with a phenomenex luna C18 column connected to a photodiode array detector with a detection wavelength of 210 nm. The peaks of butenolide were determined based on the retention time and amounts were calculated based on the established standard curves. Each treatment contained three independent replicates in three tanks, and each replicate contained one panel. Release rates (μg day−1 cm−2) of butenolide and DCOIT at six time points within 36 days were measured.
Biofilm development
Glass fiber reinforced epoxy resin panels with the above coatings were exposed at Port Shelter Bay, Hong Kong Water (22°20′16.7ʺN, 114°16′08.0ʺE) at the subtidal level in July 2015. After one month, the panels were transported back to the laboratory in a cooler with in situ seawater. The panels were washed twice using autoclaved and 0.22 μm-filtered seawater to remove loose particles and cells.
Microscopic observation
After recovery, the panels were immediately subjected to microscopic observation. Biofilms were monitored by confocal laser scanning microscopy (CLSM). Cells in biofilms were labeled with fluorescein isothiocyanate (FITC) (Sigma, Poole, UK), a dye that stains proteins. Observations were carried out using a Zeiss LSM 510 CLSM (Carl Zeiss, Jena, Germany) equipped with detectors for FITC. Images were generated using a 63 × 1.4 objective, followed by signal recording in the green channel (excitation 488 nm, emission 522/32 nm). The thickness of the biofilms was automatically calculated and exported by the attendant software of the CLSM. The substratum coverage of the biofilms was calculated using COMSTAT (Heydorn et al. Citation2000). Each treatment contained three independent replicates from three panels, and each replicate contained three observations.
RNA extraction and cDNA sequencing
After collection from the subtidal zone, the biofilms were scraped from the panels using autoclaved cotton tips and stored in RNAlater buffer (Invitrogen, Carlsbad, CA, USA) at −80°C before RNA extraction. RNA extraction was performed with the AllPrep DNA/RNA Mini Kit (Qiagen, Hilden, Germany) following the manufacturer’s instructions. The extracted RNA was subjected to DNase (Sigma-Aldrich, St. Louis, MO, USA) treatment to remove genomic DNA contamination. The RNA was then used as a template for whole-cDNA amplification using the Ovation Single-Cell RNA-Seq System, which enriches non-rRNA during cDNA synthesis using proprietary whole transcriptome primers targeting non-rRNA sequences in the transcriptome. The synthesized cDNA was sequenced using an Illumina HiSeq 2500 platform (Wuqing, Tianjin, China) after construction of 350 bp insert libraries in Novogene Bioinformatics Technology (Beijing, China). Two replicates were performed for each treatment, and each replicate contained biofilms from ten panels which were pooled together for RNA extraction.
Metatranscriptomic analysis
Metatranscriptomic analysis was performed using the method described in a previous study (Zhang et al. Citation2016). The quality of the cDNA sequences was controlled using the next-generation sequencing QC toolkit (Patel and Jain Citation2012) before being assembled by SPAdes Genome Assembler 3.6.1 (Bankevich et al. Citation2012) on a local server. The parameters used for assembly included ‘K values 21, 31, 41, 51, 61, 71, and 81’ and ‘–careful’. The open reading frames of the functional genes were predicted using Prodigal (Hyatt et al. Citation2010) and then annotated using BLASTX against the Clusters of Orthologous Groups (COGs) (Galperin et al. Citation2015) database. The reads were mapped to the genes using Bowtie 2 (Langmead and Salzberg Citation2012). The taxonomic structure of the biofilms was obtained by importing the BLASTX results into MEGAN 6.0 (Huson et al. Citation2016). The expression abundance for a single gene is the number of transcripts mapped to this gene and then normalized to the gene length, while expression abundance for one COG is the summary of expression abundances of genes in this COG category.
Statistical analysis
To compare biofilm characteristics (substratum coverage and average thickness) on different panels, the Student’s t-test was used to determine the significant differences. To compare the phylum, genus and COG abundance of different biofilm groups, one-way ANOVA was employed using values from two biological biofilm replicates as queries. At the phylum level, 16 microbial phyla with an abundance > 1% in at least one biofilm were used as input. At the genus level, 25 genera with an abundance > 1% in at least one biofilm were used as input. For functional comparison, 416 COGs with an abundance > 0.1% in at least one biofilm were used as input. The statistical analyses were run on proportion data. Prior to the Student’s t-test and the ANOVA test, a Shapiro–Wilk test and Levene’s test were carried out using SPSSv13.0 software (SPSS Inc, Chicago, IL, USA) to test the normality and the homogeneity of the data, respectively. The similarities of the functional community structures were assessed using the principal component analysis (PCA) tool implemented in the software package PAST (Hammer et al. Citation2001).
Data deposition
The metatranscriptomic data have been submitted to the Sequence Read Archive database under accession numbers PRJNA387511 and have been released.
Results and discussion
Coating toxicity
The content of Sn (indicating the concentration of DBTDL) in the butenolide and DCOIT coatings was < 0.005%. However, because the catalyst DBTDL used in the polymer synthesis is considered to be a biocide, the potential toxicity of the DBTDL in the polymers was evaluated. The polymers after and before purification containing different concentrations of DBTDL (Sn% = 0.005% and 0.037%, respectively) were included for evaluation. Biofilm formation of two marine bacterial strains isolated from subtidal biofilms, species of the Sphingomonadaceae and Pseudoalteromonas sp., on the two polymers was observed using CLSM. No significant difference in substratum coverage and average thickness could be detected between biofilms on the polymers containing different concentrations of DBTDL (Figure A, B and Table ). The toxicity of DBTDL was further evaluated by observing bacterial growth in medium with or without polymers (Figure C and D). Similarly, no inhibitive effect could be detected for the polymers on the growth of the two bacterial strains. These results suggested that the remaining DBTDL in the polymers should have no impact on the anti-biofilm assay of the butenolide coatings.
Figure 2. Toxicity evaluation of DBTDL by using CLSM observation of biofilm formation and growth curve analysis. Two marine bacterial strains, Sphingomonadaceae sp. and Pseudoalteromonas sp., were used for analysis. Two polymers with different concentrations of DBTDL were included for comparison: polymer 1, polymer containing 0.2 wt% DBTDL and then precipitated twice (Sn%=0.005%); polymer 2, polymer containing 0.2 wt% DBTDL and without being precipitated (Sn%=0.037%). Biofilms of the Sphingomonadaceae sp. (A) and Pseudoalteromonas sp. (B) were allowed to form on different polymers coated on glass slides for 8 h at 22°C, and then observed by using CLSM. The growth curve analyses of the Sphingomonadaceae sp. (C) and Pseudoalteromonas sp. (D) were performed in LB medium containing polymers with different concentrations of DBTDL for 18 h at 22°C.
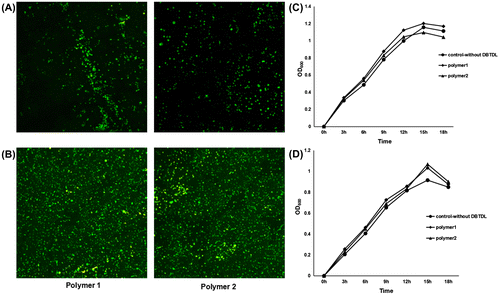
Table 1. Biofilm formation by two marine bacterial strains on polymers with different concentrations of DBTDL.
Release rate of butenolide from coatings
Figure A shows the release rates of butenolide from coatings with different concentrations. For each concentration, the release rates at different time points after the panel was immersed in seawater were monitored. As a result, the release rate varied with the concentration of butenolide: the higher the concentration, the faster it was released into the seawater. For concentrations of 5 wt% and 10 wt%, the release rates were > 12 μg day−1 cm−2. For concentrations of 1 wt% and 2.5 wt%, the release rates were < 10 μg day−1 cm−2. However, at a given concentration, only a slight decrease could be observed over time. These results suggest that butenolide is released constantly from the coating for at least one month, and the release rate can be changed by adjusting the concentration.
Figure 3. Release of butenolide (A) and DCOIT (B) from the coatings into seawater. Coatings containing four different concentrations (1, 2.5, 5, and 10 wt%) of butenolide (Bu) and DCOIT (Dc) were studied, while experiments were performed at six time points spanning 36 days.
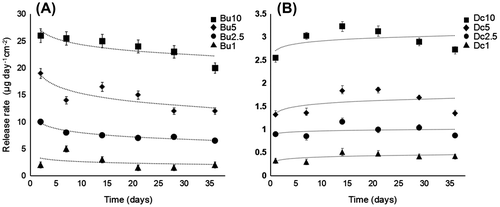
As a biocompatible and biodegradable polymer, poly (ε-caprolactone)-based polyurethane is suitable for sustained delivery of bioactive compounds over a period of one year (Sisson et al. Citation2013). Slow degradation of poly (ε-caprolactone)-based polyurethane without generation of an acidic environment, as well as its ability to blend with other agents make this polymer applicable for long term drug release (Sisson et al. Citation2013). Consistent with these previously reported characteristics of poly (ε-caprolactone), the matrix formed by this polymer provided a controllable release of butenolide into seawater. The poly (ε-caprolactone)-based polyurethane is hydrophobic, and water cannot easily diffuse into the polymer. Thus, the release of butenolide is largely determined by the erosion of the polymer matrix coming from the degradation of polymer.
For comparison, the release rates of DCOIT were also measured (Figure B). Overall, DCOIT showed a lower release rate than butenolide. At an early stage (< 15 days), the release rate of DCOIT tended to increase whereas that of butenolide has slightly decreased. Such differences are attributable to different states of DCOIT (solid) and buternolide (liquid and easy to be dissolved in water).
The anti-biofilm effect of the butenolide/polymer coating
The anti-biofilm effectiveness of the coating was visualized by direct imaging (Figure ) and CLSM analysis (Figure ). The results showed almost no biofilm attached to the panels of 10 wt% butenolide and only a small amount of biofilm on the panels with 2.5 and 5 wt% butenolide. Thick biofilms were found on the panels with 0 and 1 wt% butenolide and a few bacterial cells had attached to the surfaces with 5 wt% DCOIT. In addition to the microbial films, a reduction of macroorganisms attached to the panels with 5 and 10 wt% butenolide, (and DCOIT) was observed in comparison with other panels. Based on their appearance, most of the macroorganisms attached to the panels with 0, 1 and 2.5 wt% butenolide were of the polychaete Hydroides elegans, a fouling organism widely distributed in the coastal areas of Hong Kong Water. It has been reported that biofilm plays an important role in the settlement of H. elegans (Unabia and Hadfield Citation1999; Lau et al. Citation2002; Chung et al. Citation2010). Thus, the inhibition of attachment of these macroorganisms to the panels by butenolide could also be due to the inhibition of biofilm formation, in addition to the direct function of butenolide in preventing larval settlement (Xu et al. Citation2010; Zhang et al. Citation2012).
Figure 4. The AF performance of surfaces coated with poly (ε-caprolactone)-based polyurethane mixed with butenolide at different concentrations (1, 2.5, 5 and 10 wt%). Polyurethane coated surfaces either without compounds (Con) or with 5 wt% of DCOIT (Dc) were also included for analyses. The anti-biofilm test was performed in a subtidal environment for 36 days, and then observed by direct imaging.
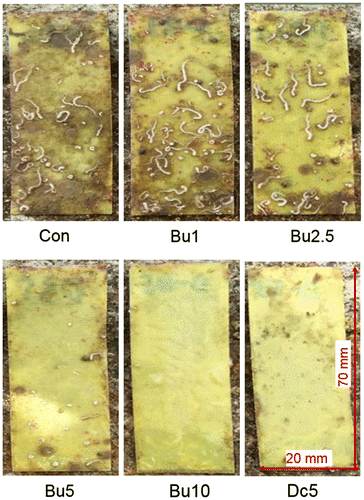
Figure 5. Microscopic observation of the biofilms developed on different coatings. The anti-biofilm experiment and the abbreviations are described in the caption to Figure 4.
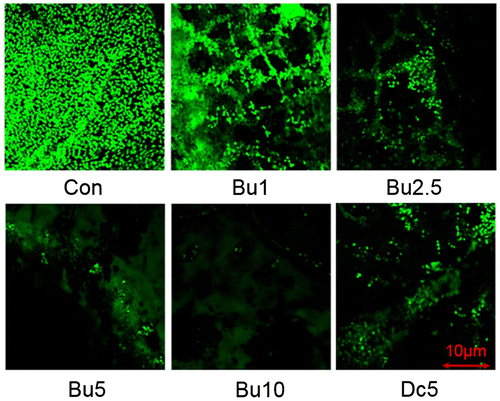
The results of CLSM (Figure ) were consistent with the direct observations. The average coverage of the biofilms measured on the basis of the CLSM images (Table ) for the control biofilms was 92.36%, whereas the average coverage for the biofilms on 5 and 10 wt% butenolide was < 15%. Similarly, the average thickness of the biofilms on 5 and 10 wt% butenolide was significantly less than that of the control group (Student’s t-test, p < 0.01). Taken together, the butenolide coatings showed a good anti-biofilm performance.
Table 2. CLSM-based characteristics of biofilms on different coatings deployed in the subtidal zone. The substratum coverage and the average thickness were calculated based on the CLSM images of three biological replicates. Butenolide (Bu): 1, 2.5, 5 and 10 wt%; DCOIT (Dc): 5 wt%.
Taxonomy dependence of the anti-biofilm effect
To understand the molecular mechanisms of how butenolide prevents biofilm development and the interaction between this chemical and microbes, three types of biofilms, including biofilms developed on 5 wt% butebolide, 5 wt% DCOIT and the control panel (only coated with the polymer) were selected for metatranscriptomic analysis. Metatranscriptomics can reveal both the taxonomic composition and active functions of complex microbial communities. A total of 10.76 Gb of metatranscriptomic sequences were generated (two biological replicates for each type of biofilm and in total six metatranscriptomes), and the sequence information is summarized in Table . It was hypothesized that, given the different anti-biofilm properties of the three types of panels, variances in the biofilm compositions should be observed. MEGAN analysis was performed to determine the biofilm community structures, which were first observed at the phylum level (Proteobacteria were classified down to classes). As a result, 16 microbial taxa were detected with an abundance > 1% in at least one biofilm (Figure ). Statistical analysis suggested seven of the 16 groups with significant changes in relative abundance (one-way ANOVA running on proportion data, p < 0.01 and the F values and p values for the comparisons at phylum level are given), including Bacteroidetes/Chlorobi (F = 83.01, p = 0.002), Chloroflexi (F = 47.21, p = 0.005), Gammaproteobacteria (F = 54.80, p = 0.004), Cyanobacteria (F = 345.96, p = 0.000), Actinobacteria (F = 33.28, p = 0.009), Chlamydiae/Verrucomicrobia (F = 60.06, p = 0.004), and Fibrobacteres/Acidobacteria (F = 650.25, p = 0). The microbes were then classified down to the genus level. In total 25 genera were identified with an abundance > 1% in at least one biofilm (Figure ) and 13 showed significant changes (one-way ANOVA, p < 0.01) between the three biofilm groups, including Bacteriovorax, Alteromonas, Rhodobacteraceae, Acetobacteraceae, Azospirillum, Thalassospira, Alphaproteobacterium HIMB59, Gilvimarinus, Parvibaculum, Cycloclasticus, Beggiatoa, Phaeospirillum and Bacillus.
Table 3. The sequence profile of the metatranscriptomic paired-end reads sequenced using the Illumina 2500 platform. Reads from the two ends were assembled together. Two biological replicates (for example, Bu5-1 and Bu5-2) were sequenced for each type of biofilm.
Figure 6. Comparison of the microbial community structures of different biofilm groups based on metatranscriptomic analysis. The structure was visualized at the phylum level, while Proteobacteria are further classified into the classes. The relative abundance of a certain taxon was calculated based on the number of reads mapped to transcripts classified to this taxon. The abbreviations of the biofilm names are shown in Figure 4 and each sample has two biological replicates (–1 and –2).
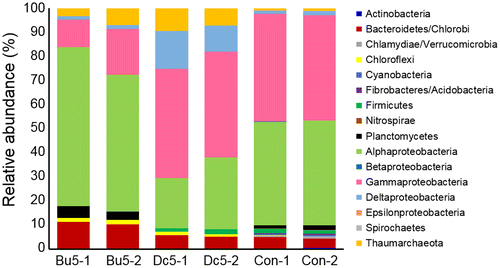
Figure 7. Microbial community structures of different biofilm groups at the genus level. The relative abundance of a certain genus was calculated based on the number of reads mapped to transcripts classified to this genus. The abbreviations of the biofilm names are described in Figure 4 and each sample has two biological replicates (–1 and –2).
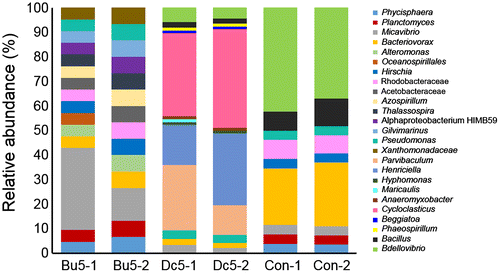
These results showed different community structures between the butenolide-treated and the DCOIT-treated biofilms, suggesting that the two anti-biofilm compounds had distinct effects. These data are consistent with previous findings that the community structure of biofilms in marine environments depends on surface characteristics (Zhang et al. Citation2014, Citation2015). For instance, in a cold seep brine pool, the relative abundance of Firmicutes was found to be higher in the biofilms developed on aluminum and polytetrafluoroethylene than that in the biofilms on titanium, stainless steel, polyetheretherketone and polyvinyl chloride (Zhang et al. Citation2014). One possible explanation is the selection of microbial inhabitants of the water during biofilm assembly, a process called ‘species sorting’ (Zhang et al. Citation2014). In addition, a long-term investigation revealed the influence of biocide coatings on taxonomic structure of marine biofilms (Muthukrishnan et al. Citation2014). More specifically, results of the present study are consistent with findings in recent studies of marine biofilm communities colonizing antifouling coatings, where Proteobacteria and Bacteroidetes were most affected by biocide coatings (Yang et al. Citation2016; Briand et al. Citation2017). Therefore, the enrichment of particular microbes in each biofilm group (such as Cycloclasticus enriched in the DCOIT-treated biofilms) implies that the compounds are less effective for some microbial taxa than for others during biofilm assembly.
In addition, it is worthwhile noting that the taxonomic composition is similar to that observed in a previous study (Chung et al. Citation2010), where biofilms were developed at the same location in Hong Kong Water. In the study by Chung et al. (Citation2010), the subtidal biofilms were also dominated by Actinobacteria, Bacteroidetes and Proteobacteria, while the Proteobacteria were composed of Alpha, Beta, Gamma, Delta and Epsilon groups. One exception was the Thaumarchaeota, which accounted for 2–8% in the biofilms in the present study, but were absent in the study of Chung et al. (Citation2010). One explanation is that the technique used by Chung et al. (Citation2010) in community profiling (denaturing gradient gel electrophoresis) did not provide as much in-depth analysis of the microbial community as revealed by high-throughput sequence technique. Members of the Thaumarchaeota have been reported to be dominant in the microbial mats (can generally be seen as ‘larger biofilms’) in Shark Bay, Australia (Wong et al. Citation2017), and thus members of the Thaumarchaeota could also be inhabitants of the subtidal biofilms.
Butenolide targets particular functional pathways in the microbes
To further explore the mechanisms that control the interactions between butenolide and biofilms, a functional comparison was conducted to illustrate the variation in biofilms on the surfaces of different coatings. After annotation using the COG database, 416 COGs were identified with an abundance > 0.1% in at least one biofilm, and these COGs were distributed in 21 different functional categories (Figure ), suggesting high functional diversity of the biofilms.
Figure 8. Functional classification of the 416 COGs identified with an abundance > 0.1% in at least one metatranscriptome. COG categories: C, energy production and conversion; D, cell cycle control and mitosis; E, amino acid metabolism and transport; F, nucleotide metabolism and transport; G, carbohydrate metabolism and transport; H, coenzyme metabolism; I, lipid metabolism; J, translation; K, transcription; L, replication and repair; M, cell wall/membrane/envelop biogenesis; N, cell motility; O, post-translational modification, protein turnover, chaperone functions; P, inorganic ion transport and metabolism; Q, secondary structure; R, general functional prediction only; S, function unknown; T, signal transduction; U, intracellular trafficking and secretion; V, defense mechanisms; Z, cytoskeleton. The abbreviations of the biofilm names are shown in Figure 4.
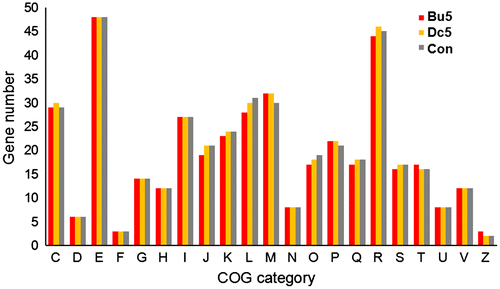
To reveal the taxonomic structural similarity between the biofilms, PCA based on the relative COG abundance was performed for the six metatranscriptomes. As a result (Figure ), the three groups of biofilms (biofilms on 5 wt% butebolide, 5 wt% DCOIT, and the control panels coated only with the polymer) were clearly separated by PC1 and PC2, explaining 64.1% and 14.5% of the variance, respectively. Moreover, PC1 suggested that the extent of similarity (indicated by how far away the samples were from each other on the PCA plot) between the butenolide-treated biofilms and the control biofilms was smaller than that between the DCOIT-treated biofilms and the control biofilms. Indeed, based on the Bray–Curtis distance, the similarity between butenolide-treated and control group was 0.506, whereas the similarity between DCOIT-treated and control group was 0.711 (average value of the two replicates). The PCA results revealed functional variation in the biofilms after butenolide treatment. To further illustrate the functional changes, statistical analysis (one-way ANOVA, p < 0.01) was employed, which revealed that, of the 416 COGs, 38 showed significantly different expression levels (p < 0.01) between the three biofilm groups. The 38 COGs could be classified into three major categories: energy production, inorganic ion transport and defense (Figure ), and their functions are discussed as follows.
Figure 9. Similarity of biofilm communities based on the 416 COG functions identified with an abundance > 0.1% in at least one metatranscriptome, as illustrated by PCA. The abbreviations of the biofilm names are described in Figure 4 and each sample has two biological replicates (–1 and –2). The eigenvalues (percentage variance for the two principle components) and COGs that contribute most heavily to the variations are indicated in the figure. COG3209, Rhs family protein; COG0843, heme/copper-type cytochrome/quinol oxidases; COG2801, transposase and inactivated derivatives; COG0732, HsdS restriction endonuclease S subunits; COG2931, RTX toxins and related Ca2+-binding proteins; COG1960, acyl-coenzyme A dehydrogenases.
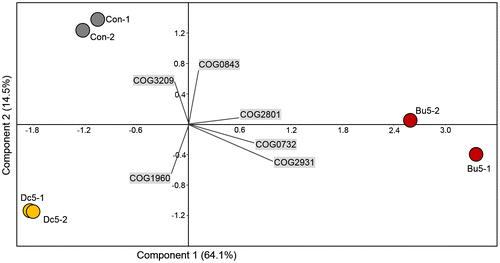
Figure 10. COGs with significantly different expression levels between the biofilms. The abbreviations of the biofilm names are shown in Figure 4 and each sample has two biological replicates. The relative expression abundance for one COG is the summary of expression abundances of genes (calculated based on the number of reads mapped to one gene and the gene length) in this COG category. One-way ANOVA was used to identify COGs that had changed significantly between Bu5, Dc5 and Con (p < 0.01).
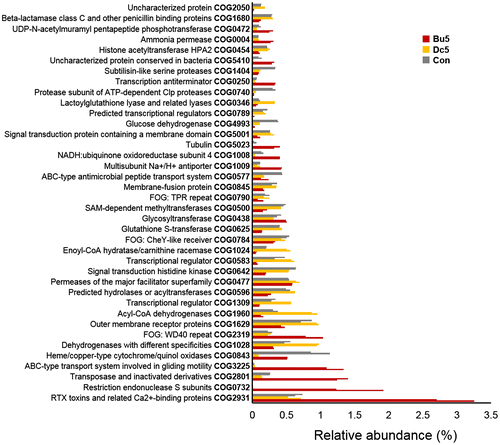
Firstly, comparing the DCOIT-treated biofilms and the control biofilms, genes involved in energy production, including enoyl-coenzyme A hydratase/carnithine racemase (COG1024) and acyl-coenzyme A dehydrogenases (COG1960) had lower abundance in the butenolide treated biofilms, suggesting inhibition of these genes by butenolide. In particular, acyl-coenzyme A dehydrogenase is an enzyme which generates energy by catalyzing the β-oxidation of long chain fatty acids (McAndrew et al. Citation2008). It has been demonstrated that butenolide binds to very long chain acyl-coenzyme A dehydrogenase, actin, and glutathione S-transferases in the barnacle Balanus (=Amphibalanus) amphitrite, and based on the result of in vitro molecular assay using purified proteins and compounds, butenolide binds to the succinyl-coenzyme A synthetase β subunit in the marine bacterium Vibrio sp. UST020129-010 to inhibit its growth (Zhang et al. Citation2012). These results imply that butenolide inhibits marine biofilm formation by altering the primary metabolism in microbes. It is worthwhile mentioning that some biocides also affect energy metabolism in bacteria. For example, tetrakis (hydroxymethyl) phosphonium sulfate has been shown to affect energy metabolic pathways in sulfate-reducing bacteria (Lee et al. Citation2010).
Secondly, genes encoding ATP-binding cassette (ABC) transporters (COG3225) were enriched in the butenolide treated biofilms. The COG3225 ABC transport family is related to cell motility and export of chemicals or proteins to the periplasm or extracellular space (Hunnicutt et al. Citation2002). It has been reported that ABC transporters play roles in drug resistance by pumping a variety of drugs out of cells at the expense of ATP hydrolysis (Choi Citation2005). For a certain bacterium, multiple drug transport systems are used to evade the toxic effects of antibiotics and other drugs (van-Veen et al. Citation1998), and a wide variety of structurally unrelated compounds are actively excreted from bacterial cells by the drug transport mechanism (Paulsen et al. Citation1996; Lewis et al. Citation1997). Thus, ABC-type multidrug transporters could be induced by butenolide, hinting at the role of multidrug transporters in resistance to antifoulants. However, it remains unclear whether specific transporters and protein-compound interactions are responsible for efflux of butenolide from bacterial cells and detoxification.
Thirdly, repeats-in-toxin (RTX) toxins (COG2931), first classified as ‘hemolysins’ (Goebel and Hedgpeth Citation1982), were significantly overexpressed in butenolide-treated biofilms, suggesting their roles as possible molecular targets of butenolide. RTX toxins have been shown to be involved in important cellular processes inducing necrosis, apoptosis and inflammation (Frey and Kuhnert Citation2002); they induce the production of inflammatory mediators in vivo while performing a cytotoxic activity in eukaryotic cells (Czuprynski and Welch Citation1995; Welch Citation2001). Thus, due to stimulation from butenolide, a higher expression level of RTX toxins can result in eukaryotes being attacked more strongly, thereby inhibiting the adherence of eukaryotes to the surfaces.
Concluding remarks
Butenolide is a promising antifoulant that inhibits larval settlement. In the present study, the anti-biofilm effect was shown through in situ experiments using a controlled release system. Because the attachment and metamorphosis of many fouling organisms rely highly on biofilms, the inhibition of biofilm formation is likely to be an important cause of its anti-biofouling mechanism. The anti-biofilm performance of the butenolide/polymer coating can be attributed to both the constant release of butenolide, which was higher than DCOIT at the same concentration, and its direct interactions with microbes from a variety of phyla. Furthermore, the potential targets and mechanism of action of butenolide in the microbes may involve energy metabolism, drug transport and toxin production. The butenolide/polymer coating is an environmental friendly and effective anti-biofilm material, and current efforts are focused on developing it into a commercial product.
Disclosure statement
The authors declare that there is no conflict of interest.
Funding
This work was supported by a NSFC grant (#41576140) to PY Qian, and COMRRDA17SC01 project.
References
- Babu SS, Mathew S, Kalarikkal N, Thomas S, Radhakrishnan EK. 2016. Antimicrobial, antibiofilm, and microbial barrier properties of poly (ε-caprolactone)/cloisite 30B thin films. 3. Biotech. 6:249.
- Bankevich A, Nurk S, Antipov D, Gurevich AA, Dvorkin M, Kulikov AS, Lesin VM, Nikolenko SI, Pham S, Prjibelski AD, Pyshkin AV, et al. 2012. SPAdes: a new genome assembly algorithm and its applications to single-cell sequencing. J Comput Biol. 19:455–477. doi: 10.1089/cmb.2012.002110.1089/cmb.2012.0021
- Bhosle NB, Evans LV, Edyvean RGJ. 1994. The effect of cathodic polarisation on monosaccharides of Amphora coffeaeformis, a marine fouling diatom. Biofouling. 8:71–79. doi: 10.1080/0892701940937826210.1080/08927019409378262
- Briand JF, Barani A, Garnier C, Réhel K, Urvois F, LePoupon C, Bouchez A, Debroas D, Bressy C. 2017. Spatio-temporal variations of marine biofilm communities colonizing artificial substrata including antifouling coatings in contrasted French coastal environments. Microb Ecol. 74:585–598.
- Chen X, Suwarno SR, Chong TH, McDougald D, Kjelleberg S, Cohen Y, Fane AG, Rice SA. 2013. Dynamics of biofilm formation under different nutrient levels and the effect on biofouling of a reverse osmosis membrane system. Biofouling. 29:319–330. doi: 10.1080/08927014.2013.77214110.1080/08927014.2013.772141
- Chen L, Xu Y, Wang W, Qian PY. 2015. Degradation kinetics of a potent antifouling agent, butenolide, under various environmental conditions. Chemosphere. 119:1075–1083. doi: 10.1016/j.chemosphere.2014.09.05610.1016/j.chemosphere.2014.09.056
- Choi CH. 2005. ABC transporters as multidrug resistance mechanisms and the development of chemosensitizers for their reversal. Cancer Cell Int. 5:30. doi: 10.1186/1475-2867-5-3010.1186/1475-2867-5-30
- Chung HC, Lee OO, Huang YL, Mok SY, Kolter R, Qian PY. 2010. Bacterial community succession and chemical profiles of subtidal biofilms in relation to larval settlement of the polychaete Hydroides elegans. ISME J. 4:817–828. doi: 10.1038/ismej.2009.15710.1038/ismej.2009.157
- Clare AS. 1996. Marine natural product antifoulants: status and potential. Biofouling. 9:211–229. doi: 10.1080/0892701960937830410.1080/08927019609378304
- Czuprynski CJ, Welch RA. 1995. Biological effects of RTX toxins: the possible role of lipopolysaccharide. Trends Microbiol. 3:480–483. doi: 10.1016/S0966-842X(00)89016-210.1016/S0966-842X(00)89016-2
- Dobretsov S, Dahms HU, Qian PY. 2006. Inhibition of biofouling by marine microorganisms and their metabolites. Biofouling. 22:43–54. doi: 10.1080/0892701050050478410.1080/08927010500504784
- Flemming HC. 1997. Reverse osmosis membrane biofouling. Exp Therm Fluid Sci. 14:382–391. doi: 10.1016/S0894-1777(96)00140-910.1016/S0894-1777(96)00140-9
- Frey J, Kuhnert P. 2002. RTX toxins in Pasteurellaceae. Int J Med Microbiol. 292:149–158. doi: 10.1078/1438-4221-0020010.1078/1438-4221-00200
- Fusetani N. 2004. Biofouling and antifouling. Nat Prod Rep. 21:94–104. doi: 10.1039/b302231p10.1039/b302231p
- Fusetani N. 2011. Antifouling marine natural products. Nat Prod Rep. 28:400. doi: 10.1039/C0NP00034E10.1039/C0NP00034E
- Galperin MY, Makarova KS, Wolf YI, Koonin EV. 2015. Expanded microbial genome coverage and improved protein family annotation in the COG database. Nucleic Acids Res. 43:261–269. doi: 10.1093/nar/gku122310.1093/nar/gku1223
- Goebel W, Hedgpeth J. 1982. Cloning and functional characterization of the plasmid-encoded hemolysin determinant of Escherichia coli. J Bacteriol. 151:1290–1298.
- Heydorn A, Nielsen AT, Hentzer M, Sternberg C, Givskov M, Ersbøll BK, Molin S. 2000. Quantification of biofilm structures by the novel computer program COMSTAT. Microbiology. 146:2395–2407. doi: 10.1099/00221287-146-10-239510.1099/00221287-146-10-2395
- Hammer Ø, Harper DAT, Ryan PD. 2001. PAST: paleontological statistics software package for education and data analysis. Palaeontol Electron. 4:9.
- Holm ER, Nedved BT, Phillips N, Deangelsis KL, Hadfield MG, Smith CM. 2000. Temporal and spatial variation in the fouling of silicone coatings in Pearl harbor. Biofouling. 15:95–107. doi: 10.1080/0892701000938630110.1080/08927010009386301
- Huang S, Hadfield MG. 2003. Composition and density of bacterial biofilms determine larval settlement of the polychaete Hydroides elegans. Mar Eco Prog Ser. 260:161–172. doi: 10.3354/meps26016110.3354/meps260161
- Hunnicutt DW, Kempf MJ, McBride MJ. 2002. Mutations in Flavobacterium johnsoniae gldF and gldG disrupt gliding motility and interfere with membrane localization of GldA. J Bacteriol. 184:2370–2378. doi: 10.1128/JB.184.9.2370-2378.200210.1128/JB.184.9.2370-2378.2002
- Huson DH, Beier S, Flade I, Górska A, El-Hadidi M, Mitra S, Ruscheweyh HJ, Tappu R. 2016. MEGAN community edition-interactive exploration and analysis of large-scale microbiome sequencing data. PLoS Comput Biol. 12:e100495.
- Hyatt D, Chen GL, LoCascio PF, Land ML, Larimer FW, Hauser LJ. 2010. Prodigal: prokaryotic gene recognition and translation initiation site identification. BMC Bioinformatics. 11:119. doi: 10.1186/1471-2105-11-11910.1186/1471-2105-11-119
- Langmead B, Salzberg SL. 2012. Fast gapped-read alignment with Bowtie 2. Nature Methods. 9:357–359. doi: 10.1038/nmeth.192310.1038/nmeth.1923
- Lau SCK, Mak KKW, Chen F, Qian PY. 2002. Bioactivity of bacterial strains from marine biofilms in Hong Kong waters for the induction of larval settlement in the marine polychaete Hydroides elegans. Mar Ecol Prog Ser. 226:301–310. doi: 10.3354/meps22630110.3354/meps226301
- Lee MHP, Caffrey SM, Voordouw JK, Voordouw G. 2010. Effects of biocides on gene expression in the sulfate-reducing bacterium Desulfovibrio vulgaris Hildenborough. Appl Microbiol Biotechnol. 87:1109–1118. doi: 10.1007/s00253-010-2596-110.1007/s00253-010-2596-1
- Lewis K, Hooper DC, Ouellette M. 1997. Multidrug resistance pumps provide broad defense. ASM News. 63:605–610.
- Ma C, Xu L, Xu W, Zhang G. 2013. Degradable polyurethane for marine anti-biofouling. J Mater Chem B. 1:3099–3106. doi: 10.1039/c3tb20454e10.1039/c3tb20454e
- McAndrew RP, Wang Y, Mohsen AW, He M, Vockley J, Kim JJ, Ryan P. 2008. Structural basis for substrate fatty acyl chain specificity: crystal structure of human very-long-chain acyl-CoA dehydrogenase. J Biol Chem. 283:9435–9443. doi: 10.1074/jbc.M70913520010.1074/jbc.M709135200
- Muthukrishnan T, Abed RM, Dobretsov S, Kidd B, Finnie AA. 2014. Long-term microfouling on commercial biocidal fouling control coatings. Biofouling. 30:1155–1164. doi: 10.1080/08927014.2014.97295110.1080/08927014.2014.972951
- Patel RK, Jain M. 2012. NGS QC Toolkit: a toolkit for quality control of next generation sequencing data. PLoS ONE. 7:e30619. doi: 10.1371/journal.pone.003061910.1371/journal.pone.0030619
- Paulsen IT, Brown MH, Skurray RA. 1996. Proton-dependent multidrug efflux systems. Microbiol Rev. 60:575–608.
- Qian PY, Xu Y, Fusetani N. 2009. Natural products as antifouling compounds: recent progress and future perspectives. Biofouling. 26:223–234. doi: 10.1080/0892701090347081510.1080/08927010903470815
- Qian PY, Li Z, Xu Y, Li Y, Fusetani N. 2015. Mini-review: marine natural products and their synthetic analogs as antifouling compounds: 2009–2014. Biofouling. 31:101–122. doi: 10.1080/08927014.2014.99722610.1080/08927014.2014.997226
- Salta M, Wharton JA, Dennington SP, Stoodley P, Stokes KR. 2013. Anti-biofilm performance of three natural products against initial bacterial attachment. Int J Mol Sci. 14:21757–21780. doi: 10.3390/ijms14112175710.3390/ijms141121757
- Sisson AL, Ekinci D, Lendlein A. 2013. The contemporary role of ε-caprolactone chemistry to create advanced polymer architectures. Polymer. 54:4333–4350. doi: 10.1016/j.polymer.2013.04.04510.1016/j.polymer.2013.04.045
- Unabia CRC, Hadfield MG. 1999. Role of bacteria in larval settlement and metamorphosis of the polychaete Hydroides elegans. Mar Biol. 133:55–64. doi: 10.1007/s00227005044210.1007/s002270050442
- van-Veen HW, Callaghan R, Soceneantu L, Sardini A, Konings WN, Higgins CF. 1998. A bacterial antibiotic-resistance gene that complements the human multidrug-resistance P-glycoprotein gene. Nature. 391:291–295. doi: 10.1038/3466910.1038/34669
- Welch RA. 2001. RTX toxin structure and function: a story of numerous anomalies and few analogies in toxin biology. Curr Top Microbiol Immunol. 257:85–111.
- Wong HL, Visscher PT, White RA III, Smith DL, Patterson MM, Burns BP. 2017. Dynamics of archaea at fine spatial scales in Shark Bay mat microbiomes. Sci Rep. 7:46160. doi: 10.1038/srep4616010.1038/srep46160
- Xu Y, He H, Schulz S, Liu X, Fusetani N, Xiong H, Xiao X, Qian PY. 2010. Potent antifouling compounds produced by marine Streptomyces. Bioresour Technol. 101:1331–1336. doi: 10.1016/j.biortech.2009.09.04610.1016/j.biortech.2009.09.046
- Yang JL, Li YF, Guo XP, Liang X, Xu YF, Ding DW, Bao WY, Dobretsov S. 2016. The effect of carbon nanotubes and titanium dioxide incorporated in PDMS on biofilm community composition and subsequent mussel plantigrade settlement. Biofouling. 32:763–777. doi: 10.1080/08927014.2016.119721010.1080/08927014.2016.1197210
- Zhang W, Ding W, Yang B, Tian R, Gu S, Luo H, Qian PY. 2016. Genomic and transcriptomic evidence for carbohydrate consumption among microorganisms in a cold seep brine pool. Front Microbiol. 7:1825.
- Zhang W, Wang Y, Bougouffa S, Tian R, Cao H, Li Y, Cai L, Wong YH, Zhang G, Zhou G, et al. 2015. Synchronized dynamics of bacterial niche-specific functions during biofilm development in a cold seep brine pool. Environ Microbiol. 17:4089–4104. doi: 10.1111/1462-2920.1297810.1111/1462-2920.12978
- Zhang W, Wang Y, Tian RM, Bougouffa S, Yang B, Cao HL, Zhang G, Wong YH, Xu W, Batang Z, et al. 2014. Species sorting during biofilm assembly by artificial substrates deployed in a cold seep system. Sci Rep. 4:6647.
- Zhang YF, Zhang H, He L, Liu C, Xu Y, Qian PY. 2012. Butenolide inhibits marine fouling by altering the primary metabolism of three target organisms. ACS Chem Boil. 7:1049–1058. doi: 10.1021/cb200545s10.1021/cb200545s