Abstract
Candida albicans is a leading cause of catheter-associated urinary tract infections and elimination of these biofilm-based infections without antifungal agents would constitute a significant medical advance. A novel urinary catheter prototype that utilizes on-demand surface deformation is effective at eliminating bacterial biofilms and here the broader applicability of this prototype to remove fungal biofilms has been demonstrated. C. albicans biofilms were debonded from prototypes by selectively inflating four additional intralumens surrounding the main lumen of the catheters to provide the necessary surface strain to remove the adhered biofilm. Deformable catheters eliminated significantly more biofilm than the controls (>90% eliminated vs 10% control; p < 0.001). Mechanical testing revealed that fungal biofilms have an elastic modulus of 45 ± 6.7 kPa with a fracture energy of 0.4–2 J m−2. This study underscores the potential of mechanical disruption as a materials design strategy to combat fungal device-associated infections.
Introduction
With over 1 million cases diagnosed annually in the USA, catheter-associated urinary tract infections (CAUTIs) remain the most common type of hospital-acquired infection (Haley et al. Citation1981; Hachem et al. Citation2009; Siddiq and Darouiche Citation2012). Among these, Candida albicans is the leading cause of fungal urinary tract infections and accounts for up to 25% of all CAUTIs in patients with a catheter beyond seven days (Pierce Citation2005; Raman et al. Citation2016). CAUTI pathogenesis begins with colonization of microbes on the catheter surface followed by proliferation and the development of a biofilm (Hawser and Douglas Citation1994; Ramage et al. Citation2006). C. albicans biofilms consist of a complex network of budding yeast and intertwined hyphae protected by an extracellular matrix scaffold composed of proteins, polysaccharides, lipids, and nucleic acids (Pierce et al. Citation2017). Eradication of fungal biofilm-related infections requires removal of the infected device as these dense biofilms are resistant to anti-fungal therapy and can evade clearance by the host immune system (Ramage et al. Citation2006, Citation2014). Strategies to combat CAUTIs, including the use of urinary catheters impregnated with either silver or antimicrobial agents, have shown mixed outcomes and have been associated with unintended tissue toxicity (Riley et al. Citation1995; Darouiche et al. Citation1997; Sampath et al. Citation2001; Gaonkar et al. Citation2003; Fisher et al. Citation2015).
In this study, C. albicans biofilms were actively removed from a urinary catheter prototype that uses on-demand surface deformation technology (Levering et al. Citation2014, Citation2016). Controlled deformation of silicone elastomers has been shown to be effective for detaching both micro- and macrofouling organisms in a variety of industrial applications (Shivapooja et al. Citation2013, Citation2015, Citation2016). Built using extrusion and replica molding, these catheters enable pressure-driven, regioselective actuation of four additional lumens that inflate into the main lumen of the catheter to mechanically disrupt adherent biofilms. These catheters and earlier model prototypes have been previously shown to successfully remove mono-microbial and mixed Gram-negative bacterial biofilms (Levering et al. Citation2014, Citation2016). Additional proof-of-concept testing using fungal biofilms was conducted to demonstrate two key points regarding the utility of this technology: (1) it is pathogen-independent and therefore applicable to biofilms formed by other common urinary pathogens beyond Gram-negative organisms; and (2) in addition to bacterial biofilms, this catheter prototype is also able to remove the structurally robust and macroscopic biofilms formed by C. albicans that are increasingly encountered clinically (Selek et al. Citation2016). Collectively, these results not only demonstrate the utility of this catheter prototype to remove fungal and bacterial biofilms without the use of antimicrobial agents, but further highlight the generalizability of this technology to remove a broad array of biofilms in both biomedical and industrial applications.
Materials and methods
Preparation of prototypes
Catheters were prepared as previously described (Levering et al. Citation2016). Briefly, extruded silicone catheter shaft components (Vesta Inc., Lanham, MD, USA) were generated according to provided specifications using Dow Corning two-part, platinum-catalyzed Class VI silicone feedstock. Silicone feedstock was used to achieve 35 durometer multi-lumen silicone main shafts and the 65 durometer silicone sheath (all durometers defined per the type A scale). The sheath was slip-fitted over the main shaft using isopropyl alcohol. The inflation lumens were then sealed using Sil-Poxy® silicone adhesive (Smooth-on Inc., Macungie, PA, USA). Long holes (~2 mm) were made in the outer walls of the inflation lumen ~1 cm from the designated hub end of the shaft. Hub manifolds were prepared by pouring silicone (Dragon Skin 0020®, Smooth-On Inc., Macungie, PA, USA) into a mold prepared by a 3-D printer as described previously (Levering et al. Citation2014). Once cured, the hubs were removed from the molds and then pierced and fitted with a male Tuohy-Borst connector to be used for inflation of the intralumens. The hubs were fitted over the designated end of the shaft and the hub was carefully glued in place without occluding the holes in the inflation lumens. This permitted the simultaneous on-demand inflation of all four lumens via the Tuohy-Borst connector. The prototypes were sterilized with ethanol before use.
Fungal strain and culture media
C. albicans (SC5314) was thawed from frozen stock and grown overnight at 37°C on yeast extract peptone dextrose (YPD) agar plates (Becton Dickenson, Sparks, MD, USA). Plates were stored at 4°C and used for up to four weeks. The artificial urine medium (AUM) formulation was prepared as previously described and composed of CaCl2 (0.65 g l−1), MgCl2 (0.65 g l−1), NaCl (4.6 g l−1), Na2SO4 (2.3 g l−1), Na3-citrate (0.65 g l−1), Na2-oxalate (0.02 g l−1), KH2PO4 (2.8 g l−1), KCl (1.6 g l−1), NH4Cl (1.0 g l−1), urea (25.0 g v), creatinine (1.1 g l−1), 5% yeast nitrogen base medium (v v–1), and 2% (w v–1) glucose (Uppuluri et al. Citation2009). The artificial urine medium was then supplemented with YPD and the pH was adjusted to 6.0. The AUM solution was sterile filtered (0.2 μm) prior to use. Colonies of C. albicans were inoculated into 3 ml of AUM and grown overnight at 37°C on an orbital shaker at 225 rpm. One ml of the overnight culture was transferred to a 50 ml flask with AUM and grown at 37°C on a shaker at 225 rpm for 8 h prior to inoculation into artificial bladders containing catheter prototypes.
Fungal biofilm growth in catheter prototypes
Biofilms were grown on the main drainage lumen of catheter prototypes using an established continuous flow method in a vertical orientation (Stickler et al. Citation1999), but modified to accommodate a manifold of four 50 ml artificial bladders (Levering et al. Citation2016). All experimental components were autoclaved sterilized or rinsed with ethanol prior to use. The artificial bladders and prototypes were maintained at 37°C in a mini-incubator outfitted with an additional insulated cover to allow for input and output ports (Figure ). The bladders each held up to a 30 ml reservoir of infected medium that would overflow into the distal tip of the catheter prototype and then drip-feed through the main drainage lumen of the prototypes as fresh medium was added to the bladder system by a peristaltic pump. The system was primed with AUM and then inoculated with 10 ml of 8 h cultures of C. albicans. The experimental setup was left to incubate for 1.5 h in the absence of flow to allow attachment and infection of the C. albicans cultures to the bladders and catheters. The flow was then restarted at a rate of 0.5 ml min−1 (within range of normal urine production rates; Burnier et al. Citation1993; Zanchi et al. Citation2004) until a mucoid biofilm was clearly visible on the walls of the prototype, or a system blockage occurred. A typical duration for preparing fungal biofilms in catheters was 42–48 h.
Figure 1. Schematic of the experimental apparatus. A continuous supply of artificial urine medium (AUM) is provided to an artificial bladder using a peristaltic pump (flow rate = 0.5 mm min–1). The top portion of the catheter is inserted into an artificial bladder while the bottom portion is connected to tubing that drains into a waste container. The entire apparatus is enclosed in a modified incubator at 37°C. Arrows symbolize the direction of medium flow.
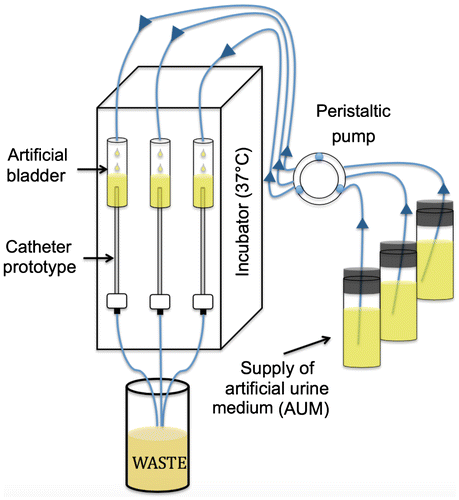
Actuation testing
Following biofilm growth, the prototypes were then gently removed from the artificial bladders and suspended vertically. AUM was introduced into the upper end of the catheter at a flow rate of 2 ml min−1 for 2 min using a syringe pump. Samples designated as controls only underwent a rinse with AUM and did not undergo actuation. Samples designated to undergo actuation were rapidly inflated to a pressure of 80 kPa (to achieve ~35% strain) and then deflated 10 times. Each inflate/deflate cycle lasted ~1–2 s, and the start of the first inflation cycle occurred ~20 s into the 2 min rinse. Actuation parameters were chosen based on previous studies that demonstrated that these settings resulted in the removal of adhered crystalline bacterial biofilms (Levering et al. Citation2014, Citation2016). Inflation was conducted pneumatically by delivering a pre-determined volume of air in a syringe to the inflation hub. Catheters were weighed before biofilm growth, before the rinse, after the rinse, and after actuation in order to assess the weight of biofilm grown and removed. The effluent from each rinse and following actuation was collected and weighed. The effluents were collected and centrifuged for 5 min at 3,500 g. The liquid supernatant was removed, and the remaining biofilm pellet was weighed. For control catheters, the percentage of biofilm removed was obtained by dividing the biofilm pellet collected after the rinse by the total weight of the biofilm immediately following growth. For actuated catheters, the percentage of biofilm removed was determined by dividing the weight of isolated pellet by the weight of the biofilm immediately following growth.
Samples generated for visual analysis were dissected into sections to obtain cross-sectional views of the main lumen along the entire shaft of the catheter. Additional pieces were stained with 0.01% (w v–1) crystal violet in water for 10 min and rinsed twice with deionized water before catheters were dissected to obtain both cross sectional and longitudinal views. Stained sections were optically photographed for comparison.
Scanning electron microscopy (SEM) imaging
Samples generated for SEM images were dissected into sections to obtain cross-sectional images and superficial images of the fungal biofilms. Biofilm samples were fixed in a solution of 2% glutaraldehyde (Electron Microscopy Sciences, Hatfield, PA, USA) in phosphate buffered saline (PBS) for 2 h at room temperature. Samples were rinsed twice with PBS, dehydrated through a series of ethanol washes (30% for 10 min, 50% for 10 min, 70% for 10 min, 90% for 10 min, and 100% for 10 min), and dried in a critical point desiccator. The samples were subsequently coated with 40% gold–60% palladium and imaged with a SEM (FEI XL30) in high-vacuum mode at 5 kV.
Mechanical testing
Tensile testing
Biofilms used for tensile testing were manually extracted from catheters following the growth period and stored in PBS at 4°C for up to 72 h prior to testing. Samples were warmed to room temperature in PBS and then loaded vertically between the two fixtures of Micro-strain Analyzer (MSA, TA Instruments RSA III, New Castle, DE, USA), which is suitable for soft materials. Biofilm samples were tested in air at room temperature, and only those samples with a length-to-width ratio > 1:3 were used for testing. The mounted biofilm samples were stretched at a strain rate of 0.001 s−1 for 100 s and the stress was concurrently recorded. Measurements were performed once on each sample to avoid any structural distortion that could confound measurements on repeat testing. The elastic modulus (E) of biofilms was extracted by measuring the slope of the linear region of the obtained stress (σ)–strain (ɛ) curves according to Hooke’s law. This approach has been previously used to calculate E from compression testing of biofilms of C. albicans mutants (Paramonova et al. Citation2009).
Fungal biofilm toughness was evaluated by determining the fracture energy, which is measured with a two-step approach under identical conditions to the aforementioned procedure. In the first step, a small notch was made at the center of the mounted biofilm edge to create a defect, which would initiate the crack propagation when the biofilm failed during the loading process. The critical strain at which the biofilm failed, ɛc, could be identified from the onset of the steep drop of stress on the σ–ɛ curves. In the second step, similarly prepared biofilms without a notch were mounted to the MSA and stretched to obtain stress–stretch () curves, where
. The area under the
curves, until the critical stretch
(=1+ɛc), was integrated to determine the fracture energy (Pharr et al. Citation2012; Sun et al. Citation2012).
Adhesion testing using Atomic Force Microscopy
Biofilms generated for adhesion measurements were manually extracted from catheters and stored in PBS at 4°C for up to 72 h prior to testing. Samples were then mounted onto a clean and stiff surface to provide adequate support (crystalline silicon wafer pieces), hydrated with fresh AUM, and tested at room temperature.
Atomic Force Microscopy (AFM) force–distance curves can show the interaction forces between the AFM tip and a sample before, during, and after contact by approaching and retracting the AFM tip to the sample. To measure the adhesion forces between silicone and biofilm, the contact angle of water on the inner surface of the catheter was measured and then gold-coated colloidal AFM tips were chemically treated to reach the same wettability. The adhesion energy was then derived from curves generated by indenting and retracting the modified AFM probes into fungal biofilms (Figure S1). Calibrated silicon nitride cantilever probes (spring constant k = 0.01 N m−1; Novascan, Ames, IA, USA) with gold-coated borosilicate glass spheres (D = 12 μm) were used in all experiments. The contact angle of water measured on the inner surface of the catheter was 87 ± 3°. A 1:1 mixture of hydrophilic (11-mercapto-1-undecanol; Sigma Aldrich, St Louis, MO, USA) was used and hydrophobic (1-undecanethiol; Sigma Aldrich) thiol-terminated alkanethiols to replicate this contact angle on the gold-coated AFM probes. Briefly, the functionalized probes were rinsed with ethanol, plasma-ashed for 2 min, and immersed in the alkanethiol mixture for 45 min. Following incubation, the probes were removed from the solution and immersed first in ethanol, then in deionized water, and then finally left to air dry. The contact angle on the modified probes was measured to be 85 ± 5°, which is in line with previously reported values (Callow et al. Citation2000) and matches that of the catheter material. The probes were used within 3 h of preparation to prevent degradation of the self-assembled monolayer (SAM) surface.
Adhesion energies were measured from the pull-off forces using AFM (MFP-3D, Asylum Research, Santa Barbara, CA, USA). Prior to the experiments, the cantilever sensitivity on a clean silicon surface was determined by comparing the z-piezoelectric actuator motion during contact with respect to the photodiode signal. For adhesion measurements, the probes were immersed in AUM and then brought in contact with the mounted biofilm samples. Adhesion curves were obtained at an approach and retraction speed of 800 nm s−1 after first allowing the AFM probe to contact the surface with a force of 1 nN and then withdrawing the probe. All force–distance curves were obtained in contact mode at room temperature in a solution of AUM. Five locations on two independent biofilm samples were tested (n = 5). Thirty force–distance curves were obtained for each location, and for each location, the first 10 force curves were selected for analysis. The pull-off forces are measured from peak negative force in the withdraw curves. The work of adhesion, ie the energy required per unit area to separate two bodies in contact, is calculated by determining the appropriate contact model and the pull-off force and work of adhesion relation. The Asylum MFP-3D software AFM (MFP-3D, Asylum Research, Santa Barbara, CA, USA) and a custom script in MATLAB (The MathWorks, Natick, MA, USA) were used to determine the dimensionless elasticity parameter and the work of adhesion (Cappella and Dietler Citation1999).
Statistical analysis
Statistical analysis was performed using GraphPad Prism 7 (La Jolla, CA, USA) software. Group means were compared by two-tailed, t-tests with Welch’s correction to account for potentially unequal variances. Data are presented as means ± standard deviation (SD) in the graphs, where ‘***’ denotes p < 0.001.
Results and discussion
Visual and microscopic characterization of C. albicans biofilms
The formation of mature C. albicans biofilms along the length of the catheter lumen was observed visually and microscopically. Figure A shows typical biofilm formation on catheter samples compared to negative control catheters following growth for 42–48 h. Mucoid C. albicans biofilms were detected on the sample catheters as early as 18–24 h following incubation and reached an average thickness of ~0.6 mm at the end of the growth period. Negative control catheters incubated under identical experimental conditions, but lacking the addition of C. albicans, showed no significant microbial contamination by visual inspection. Biofilm formation along the length of the main lumen was also found to be largely uniform as shown by cross-sectional imaging (Figure B). In many cases, biofilms that were manually extracted from catheter prototypes yielded a macroscopic tubular structure. These intact biofilms could be handled using blunt forceps or by hand (Figure S2).
Figure 2. Representative optical images of C. albicans biofilms grown in catheter prototypes compared to a negative control (A). Cross sectional optical images of biofilm growth within the main lumen at different regions along the length of the catheter prototype (B). Scale bars = 1 cm (upper) and 1 mm (lower).

SEM imaging was used to determine the structural and morphological characteristics of the resulting C. albicans biofilms (Figure ). Images revealed that C. albicans formed complex and heterogeneous biofilms, findings that are in agreement with prior studies (Chandra et al. Citation2001; Ramage et al. Citation2008; Mukherjee et al. Citation2009). Under low magnification, biofilms appear highly dense with filamentous elements woven into a 3-D structure that retains the geometry of the main catheter lumen. Images of the inner surface of the biofilm taken at increasing levels of magnification further reveal the entangled network of hyphal and pseudo-hyphal elements throughout the entire thickness of the biofilm. The majority of the biofilm consisted of the filamentous form of the fungus; the yeast form was less apparent. At the higher levels of magnification, extracellular polymeric substance (EPS) can be seen to connect the hyphal elements.
Mechanical characterization of C. albicans biofilms
Catheter prototypes were previously designed so that their inflated walls achieved sufficient substratum strains to debond crystalline bacterial biofilms. Prior experiments showed that debonding occurred at a critical strain value between 15 and 25% (Levering et al. Citation2014, Citation2016). Thus, to ensure for adequate biofilm removal, catheters were made to exceed 30% strain in the luminal surface when subjected to pressure. Since the catheter prototypes were designed specifically for bacterial biofilms, additional mechanical testing was performed here to characterize the adhesive and cohesive failure of C. albicans biofilms. This analysis for fungal biofilms is important for both the validation of this catheter technology and for future strategies that utilize mechanical mismatch of the underlying surface and the biofilm to drive removal.
Tensile testing
Tensile testing was employed to determine the bulk mechanical properties of intact C. albicans biofilms that formed in the catheter prototypes. Suitable biofilm samples (length to width ratio > 1:3, sample length 10 mm) were extracted manually from four different catheters and used for experiments (Figure S2). Figure shows a representative stress–strain plot from tensile tests on C. albicans biofilms. The stress–strain behavior is similar to that of an elastic material at low strain (<10%) (Wang et al. Citation2002; Choi and Rogers Citation2003), with an initial linear region until ~8–10% strain and a stress peak followed by a negative slope as a crack initiated in the film and then the film failed (Figure S3). The elastic modulus, E, obtained from all tested samples was 45 ± 6.7 kPa. The critical stretches were found to be 1.10 ± 0.02 and the fracture energy of the biofilms was determined to be 0.4–2 J m–2.
Figure 4. Tensile testing of intact C. albicans biofilms formed in catheter prototypes. (A) Biofilms were manually extracted from catheter prototypes and mounted in a microstrain analyzer for tensile testing. (B) Representative stress–strain plot resulting from tensile testing of intact biofilms.
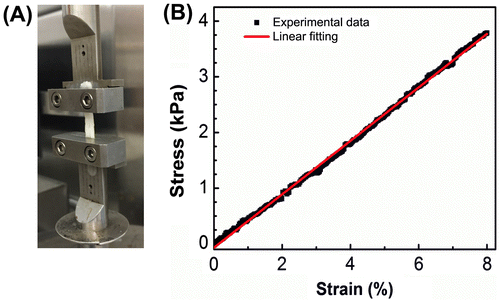
While the moduli and yield strengths found in this study are within the wide range reported for biofilms (Bol et al. Citation2013), further comparative analysis is limited given the paucity of experimental mechanical data available for fungal biofilms. Previously reported values for the compression moduli of biofilms of C. albicans mutants were ~20–300 Pa, of Candida tropicalis were 40 Pa, and of Candida parapsilosis were 30 Pa (Paramonova et al. Citation2009). It is noted, however, that variations in the elastic moduli values across different studies likely result from differences in the growth conditions (eg temperature), and other factors including shear stress and surface characteristics of the substratum known to affect the extent of microbial adhesion and biofilm formation (Stoodley et al. Citation2002; Garrett et al. Citation2008).
Adhesion testing using AFM
The adhesion force (pull-off force) measured between the SAM-coated AFM probes and the fungal biofilm was 1.95 nN ± 0.57 nN and the average distance required to pull the probe off the biofilm substratum was 0.46 ± 0.14 μm. The dimensionless elasticity parameter of the modified tip–biofilm contact varies between 0.25 and 1.5, which means the observed force–distance curves can be best explained by the Maugis contact model (Maugis Citation1992). This model assumes a constant adhesion force ring around the circular contact area and requires numerical and analytical solutions to determine the contact parameters. To reduce the complexity of this calculation, the relationship between the work of adhesion and the pull-off force was determined from previously computed tables for the Maugis model (Cappella et al. 1999; Zhao et al. Citation2003). The work of adhesion for the five experimental datasets in this study was 6.05 ± 1.35 × 10–5 J m–2, and these values are within the range reported from AFM adhesion studies on tissues and biofilms (Lau et al. Citation2009). This large variation in adhesion energy density is attributed to the underlying structural heterogeneity of the biofilm. Mechanisms driving structural heterogeneity in fungal biofilms are multifactorial, with contributing factors including the simultaneous presence of multiple cell morphologies (yeast, pseudo-hyphae, hyphae) as well as differences in extracellular DNA content (Bonhomme and d’Enfert Citation2013; Rajendran et al. Citation2014). The implications of microscale heterogeneity in the extracellular scaffold of biofilms was recently investigated in Pseudomonas aeruginosa and was found to have major effects on the local adhesion behavior of cells in response to a mechanical insult (Jang et al. Citation2017).
It is furthermore noted that the adhesion energy density was an order of magnitude higher for SAM-coated probes compared to non-functionalized borosilicate glass colloidal probes (data not shown). This observation illustrates that the energy of the catheter surface will likely have an important effect on the adhesion energy between the biofilm and the catheter (Baier et al. Citation1968; Absolom et al. Citation1983; Van Oss et al. Citation1986, Citation1987, Citation1988). Interestingly, the adhesion energy density obtained in the present experiments is significantly lower than that previously reported for bacterial biofilms grown on a flat silicone surface (Chen et al. Citation1998; Levering et al. Citation2014, Citation2016). This difference in adhesion energy may be due to a variety of reasons including: (1) differences in the methodology of the adhesion test, and (2) inherent differences in the adhesion of fungal biofilms grown in a catheter compared to bacterial biofilms grown on planar silicone substrata.
Debonding C. albicans biofilms using catheter prototypes
When actuated, all catheter prototypes showed visually obvious and thorough removal of the biofilm compared to control catheters (Videos S1, S2). Biofilm removal was associated with the presence of significant amount of debris in the effluent. An average of 0.75 g of biofilm was removed from the actuated prototypes, compared to 0.065 g in the control prototypes which only underwent a rinse. This suggests that >90% of the biofilm mass was removed upon actuation of the catheters (Figure ).
Figure 5. Catheter prototypes debond C. albicans biofilms. Representative cross sectional (A) and longitudinal (B) optical images of control (not inflated, left) and debonded (inflated, right) biofilms in the main lumen of the catheter prototypes. Biofilms were stained with crystal violet to enhance visualization. Scale bars = 2 mm (A) and 5 mm (B). (C) Percentage biofilm mass removed from the control and inflated catheters (N = 4). ‘***’ indicates p < 0.001.
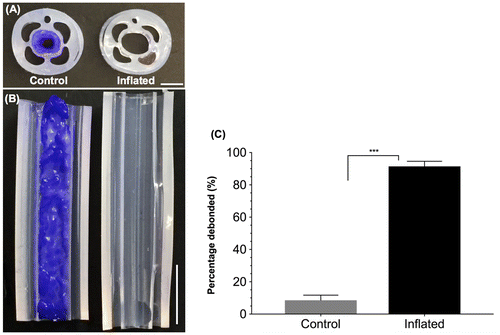
To further visualize biofilm removal, both control and actuated catheter prototypes were sectioned and stained with crystal violet to visualize the biofilms (Figure ). For actuated catheters, no significant amount of residual straining was observed, suggesting that the biofilm has been completely debonded from the catheter lumen surface. The surface of actuated catheters was further imaged using SEM, and no appreciable amount of residual biofilm was detected (Figure S3). In contrast, for control catheters, crystal violet straining suggested that the biofilm was present throughout the catheter lumen.
Furthermore, in contrast to previous experiments involving bacterial biofilms, no significant fungal biofilm residual was detected along the area of the intraluminal surface of the catheter overlying the wall between the intra-wall inflation lumens. This region undergoes minimal tensile strain during the actuation process, which allowed for the accumulation of a residual bacterial biofilm (Levering et al. Citation2016). This modest improvement in debonding is likely facilitated by the smaller adhesion energy between fungal biofilms and the catheter surface compared to bacterial biofilms. The length of the debonded fungal biofilm was often greater than that of previously examined bacterial biofilms, and in some cases, >10 cm for fungal biofilms of an average thickness of ~0.6mm. The energy release rate (G) of the fungal biofilm was calculated using the equations developed in previous studies for high L/H (length/thickness) biofilms: G/μfH = ((1 + ε)2+(1 + ε)−2 – 2)/2 (Levering et al. Citation2014, Citation2016). In this equation, μf is the shear modulus and can be calculated by using the measured E in the tensile experiments and Poisson’s ratio (assumed to be ~0.49 for almost incompressible biofilms) (Mahmoud et al. Citation2014). The resulting shear modulus was 15.1 ± 2.2 kPa. When a 35% strain is applied to the luminal surface during actuation, the resulting energy release rate is 1.68 ± 0.15 J m–2 which lies within the range of the experimentally determined fracture energy of 0.4–2.0 J m–2. Since a number of the debonded biofilm fragments were significantly longer relative to their thickness, it is concluded that the adhesion energy was lower than the fracture energy and energy release rate. It is clear that overcoming the adhesive bond strength between the biofilm and the catheter and not the cohesive failure of the biofilm itself drives the delamination of the biofilms from actuated catheter surfaces. This also supports the conclusions drawn regarding the adhesion energy difference between fungal and bacterial biofilms, ie if the adhesion energy of the fungal biofilms were as high as that of the bacterial biofilms, the films would first break into small pieces before debonding.
Conclusions
This study demonstrates the highly effective removal (almost total removal when assessed visually and with SEM and >90% by weight) of adhered C. albicans biofilms from novel catheter prototypes through the application of pressure-driven surface actuation and is the first demonstration of fungal biofilm removal in any milieu using this technique. Importantly, this technology is pathogen-independent and does not require the use of antimicrobials, two features that are critical given that a significant number of CAUTIs are poly-microbial and consist of antibiotic resistant organisms. Future work will involve in vivo models as well as performance testing after multiple rounds of use. Design of biomaterials that utilize mechanical disruption represent an innovative and effective approach to biofilm removal from catheters and other elastomeric substrata. Finally, this study further supports the effectiveness of surface deformation-based approaches to manage biofouling in number of scientific areas ranging from industrial to biomedical applications.
Funding
This research was supported by the National Institutes of Health to SAM [5T32-AI052080–12], JRP [R01AI073896 and R01AI093257], and VGF [2K24-AI093969, 2R01-AI068804, and R01-HL119648] and the National Science Foundation through the Research Triangle MRSEC to QT [DMR-1121107]. The SEM and MSA work was performed in part at the Duke University Shared Materials Instrumentation Facility (SMIF), a member of the North Carolina Research Triangle Nanotechnology Network (RTNN), which is supported by the National Science Foundation (ECCS 1542015) as part of the National Nanotechnology Coordinated Infrastructure (NNCI).
Disclosure statement
VL is a scientific founder of Biofilmless, Inc. and receives equity compensation to serve on Portela Soni Medical’s Scientific Advisory Board.
Supplemental data
The supplemental material for this paper is available online at https://doi.org/10.1080/08927014.2018.1474461
Supplemental Material
Download Zip (327.8 MB)Acknowledgements
The authors thank Michelle Gignac Plue for her assistance with the SEM images.
References
- Absolom DR, Lamberti FV, Policova Z, Zingg W, van Oss CJ, Neumann AW. 1983. Surface thermodynamics of bacterial adhesion. Appl Environ Microbiol. 46:90–97.
- Baier RE, Shafrin EG, Zisman WA. 1968. Adhesion: mechanisms that assist or impede it. Science. 162:1360–1368. doi: 10.1126/science.162.3860.1360.10.1126/science.162.3860.1360
- Bol M, Ehret AE, Bolea Albero A, Hellriegel J, Krull R. 2013. Recent advances in mechanical characterisation of biofilm and their significance for material modelling. Crit Rev Biotechnol. 33:145–171. doi: 10.3109/07388551.2012.679250.10.3109/07388551.2012.679250
- Bonhomme J, d’Enfert C. 2013. Candida albicans biofilms: building a heterogeneous, drug-tolerant environment. Curr Opin Microbiol. 16:398–403. doi: 10.1016/j.mib.2013.03.007.10.1016/j.mib.2013.03.007
- Burnier M, Rutschmann B, Nussberger J, Versaggi J, Shahinfar S, Waeber B, Brunner HR. 1993. Salt-dependent renal effects of an angiotensin II antagonist in healthy subjects. Hypertension. 22:339–347. doi: 10.1161/01.HYP.22.3.339.10.1161/01.HYP.22.3.339
- Callow ME, Callow JA, Ista LK, Coleman SE, Nolasco AC, López GP. 2000. Use of self-assembled monolayers of different wettabilities to study surface selection and primary adhesion processes of green algal (Enteromorpha) zoospores. Appl Environ Microbiol. 66:3249–3254. doi: 10.1128/AEM.66.8.3249-3254.2000.10.1128/AEM.66.8.3249-3254.2000
- Cappella B, Dietler G. 1999. Force-distance curves by atomic force microscopy. Surf Sci Rep. 34:1–104. doi: 10.1016/S0167-5729(99)00003-5.10.1016/S0167-5729(99)00003-5
- Chandra J, Kuhn DM, Mukherjee PK, Hoyer LL, McCormick T, Ghannoum MA. 2001. Biofilm formation by the fungal pathogen Candida albicans: development, architecture, and drug resistance. J Bacteriol. 183:5385–5394. doi: 10.1128/JB.183.18.5385-5394.2001.10.1128/JB.183.18.5385-5394.2001
- Chen MJ, Zhang Z, Bott TR. 1998. Direct measurement of the adhesive strength of biofilms in pipes by micromanipulation. Biotechnol Tech. 12:875–880. doi: 10.1023/A:1008805326385.10.1023/A:1008805326385
- Choi KM, Rogers JA. 2003. A photocurable poly(dimethylsiloxane) chemistry designed for soft lithographic molding and printing in the nanometer regime. J Am Chem Soc. 125:4060–4061. doi: 10.1021/ja029973k.10.1021/ja029973k
- Darouiche RO, Safar H, Raad II. 1997. In vitro efficacy of antimicrobial-coated bladder catheters in inhibiting bacterial migration along catheter surface. J Infect Dis. 176:1109–1112. doi: 10.1086/jid.1997.176.issue-4.10.1086/jid.1997.176.issue-4
- Fisher LE, Hook AL, Ashraf W, Yousef A, Barrett DA, Scurr DJ, Chen X, Smith EF, Fay M, Parmenter CD, et al. 2015. Biomaterial modification of urinary catheters with antimicrobials to give long-term broadspectrum antibiofilm activity. J Controlled Release. 202:57–64. doi: 10.1016/j.jconrel.2015.01.037.10.1016/j.jconrel.2015.01.037
- Gaonkar TA, Sampath LA, Modak SM. 2003. Evaluation of the antimicrobial efficacy of urinary catheters impregnated with antiseptics in an in vitro urinary tract model. Infect Control Hosp Epidemiol. 24:506–513. doi: 10.1086/502241.10.1086/502241
- Garrett TR, Bhakoo M, Zhang Z. 2008. Bacterial adhesion and biofilms on surfaces. Prog Nat Sci. 18:1049–1056. doi: 10.1016/j.pnsc.2008.04.001.10.1016/j.pnsc.2008.04.001
- Hachem R, Reitzel R, Borne A, Jiang Y, Tinkey P, Uthamanthil R, Chandra J, Ghannoum M, Raad I. 2009. Novel antiseptic urinary catheters for prevention of urinary tract infections: correlation of in vivo and in vitro test results. Antimicrob Agents Chemother. 53:5145–5149. doi: 10.1128/AAC.00718-09.10.1128/AAC.00718-09
- Haley RW, Hooton TM, Culver DH, Stanley RC, Emori TG, Hardison CD, Quade D, Shachtman RH, Schaberg DR, Shah BV, et al. 1981. Nosocomial infections in U.S. hospitals, 1975-1976: estimated frequency by selected characteristics of patients. Am J Med. 70:947–959. doi: 10.1016/0002-9343(81)90561-1.10.1016/0002-9343(81)90561-1
- Hawser SP, Douglas LJ. 1994. Biofilm formation by Candida species on the surface of catheter materials in vitro. Infect Immun. 62:915–921.
- Jang H, Rusconi R, Stocker R. 2017. Biofilm disruption by an air bubble reveals heterogeneous age-dependent detachment patterns dictated by initial extracellular matrix distribution. NPJ Biofilms Microbiomes. 3:95.
- Lau PC, Dutcher JR, Beveridge TJ, Lam JS. 2009. Absolute quantitation of bacterial biofilm adhesion and viscoelasticity by microbead force spectroscopy. Biophys J. 96:2935–2948. doi: 10.1016/j.bpj.2008.12.3943.10.1016/j.bpj.2008.12.3943
- Levering V, Wang Q, Shivapooja P, Zhao X, López GP. 2014. Soft robotic concepts in catheter design: an on-demand fouling-release urinary catheter. Adv Healthc Mater. 3:1588–1596. doi: 10.1002/adhm.v3.10.10.1002/adhm.v3.10
- Levering V, Cao C, Shivapooja P, Levinson H, Zhao X, López GP. 2016. Urinary catheter capable of repeated on-demand removal of infectious biofilms via active deformation. Biomaterials. 77:77–86. doi: 10.1016/j.biomaterials.2015.10.070.10.1016/j.biomaterials.2015.10.070
- Mahmoud B, Zhe X, Leah G, Yingjie D, Hongbing L, Philippe Z, Majid M-J. 2014. Nanoindentation of Pseudomonas aeruginosa bacterial biofilm using atomic force microscopy. Mater Res Express. 1:045411.
- Maugis D. 1992. Adhesion of spheres: The JKR-DMT transition using a dugdale model. J Colloid Interface Sci. 150:243–269. doi: 10.1016/0021-9797(92)90285-T.10.1016/0021-9797(92)90285-T
- Mukherjee PK, Chand DV, Chandra J, Anderson JM, Ghannoum MA. 2009. Shear stress modulates the thickness and architecture of Candida albicans biofilms in a phase-dependent manner. Mycoses. 52:440–446. doi: 10.1111/myc.2009.52.issue-5.10.1111/myc.2009.52.issue-5
- Paramonova E, Krom BP, van der Mei HC, Busscher HJ, Sharma PK. 2009. Hyphal content determines the compression strength of Candida albicans biofilms. Microbiology. 155:1997–2003. doi: 10.1099/mic.0.021568-0.10.1099/mic.0.021568-0
- Pharr M, Sun J-Y, Suo Z. 2012. Rupture of a highly stretchable acrylic dielectric elastomer. J Appl Phys. 111:104114. doi: 10.1063/1.4721777.10.1063/1.4721777
- Pierce GE. 2005. Pseudomonas aeruginosa, Candida albicans, and device-related nosocomial infections: implications, trends, and potential approaches for control. J Ind Microbiol Biotechnol. 32:309–318. doi: 10.1007/s10295-005-0225-2.10.1007/s10295-005-0225-2
- Pierce CG, Vila T, Romo JA, Montelongo-Jauregui D, Wall G, Ramasubramanian A, Lopez-Ribot JL. 2017. The Candida albicans biofilm matrix: composition, structure and function. J Fungi (Basel). 3:14. doi: 10.3390/jof3010014.10.3390/jof3010014
- Rajendran R, Sherry L, Lappin DF, Nile CJ, Smith K, Williams C, Munro CA, Ramage G. 2014. Extracellular DNA release confers heterogeneity in Candida albicans biofilm formation. BMC Microbiol. 14:1.
- Ramage G, Martinez JP, Lopez-Ribot JL. 2006. Candida biofilms on implanted biomaterials: a clinically significant problem. FEMS Yeast Res. 6:979–986. doi: 10.1111/fyr.2006.6.issue-7.10.1111/fyr.2006.6.issue-7
- Ramage G, Robertson SN, Williams C. 2014. Strength in numbers: antifungal strategies against fungal biofilms. Int J Antimicrob Agents. 43:114–120. doi: 10.1016/j.ijantimicag.2013.10.023.10.1016/j.ijantimicag.2013.10.023
- Ramage G, Wickes BL, Lopez-Ribot JL. 2008. A seed and feed model for the formation of Candida albicans biofilms under flow conditions using an improved modified Robbins device. Rev Iberoam Micol. 25:37–40. doi: 10.1016/S1130-1406(08)70009-3.10.1016/S1130-1406(08)70009-3
- Raman N, Lee MR, Rodriguez Lopez Ade L, Palecek SP, Lynn DM. 2016. Antifungal activity of a beta-peptide in synthetic urine media: toward materials-based approaches to reducing catheter-associated urinary tract fungal infections. Acta Biomater. 43:240–250. doi: 10.1016/j.actbio.2016.07.016.10.1016/j.actbio.2016.07.016
- Riley DK, Classen DC, Stevens LE, Burke JP. 1995. A large randomized clinical trial of a silver-impregnated urinary catheter: lack of efficacy and staphylococcal superinfection. Am J Med. 98:349–356. doi: 10.1016/S0002-9343(99)80313-1.10.1016/S0002-9343(99)80313-1
- Sampath LA, Tambe SM, Modak SM. 2001. In vitro and in vivo efficacy of catheters impregnated with antiseptics or antibiotics: evaluation of the risk of bacterial resistance to the antimicrobials in the catheters. Infect Control Hosp Epidemiol. 22:640–646. doi: 10.1086/501836.10.1086/501836
- Selek MB, Kula Atik T, Bektore B, Atik B, Demir S, Baylan O, Ozyurt M. 2016. First report of macroscopic biofilm formation caused by Candida albicans on silver hydrogel-coated urinary catheters. Am J Infect Control. 44:1174–1175. doi: 10.1016/j.ajic.2016.03.042.10.1016/j.ajic.2016.03.042
- Shivapooja P, Wang Q, Orihuela B, Rittschof D, López GP, Zhao X. 2013. Bioinspired surfaces with dynamic topography for active control of biofouling. Adv Mater. 25:1430–1434. doi: 10.1002/adma.v25.10.10.1002/adma.v25.10
- Shivapooja P, Wang Q, Szott LM, Orihuela B, Rittschof D, Zhao X, López GP. 2015. Dynamic surface deformation of silicone elastomers for management of marine biofouling: laboratory and field studies using pneumatic actuation. Biofouling. 31:265–274. doi: 10.1080/08927014.2015.1035651.10.1080/08927014.2015.1035651
- Shivapooja P, Cao C, Orihuela B, Levering V, Zhao X, Rittschof D, López GP. 2016. Incorporation of silicone oil into elastomers enhances barnacle detachment by active surface strain. Biofouling. 32:1017–1028. doi: 10.1080/08927014.2016.1209186.10.1080/08927014.2016.1209186
- Siddiq DM, Darouiche RO. 2012. New strategies to prevent catheter-associated urinary tract infections. Nat Rev Urol. 9:305–314. doi: 10.1038/nrurol.2012.68.10.1038/nrurol.2012.68
- Stickler DJ, Morris NS, Winters C. 1999. Simple physical model to study formation and physiology of biofilms on urethral catheters. Methods Enzymol. 310:494–501. doi: 10.1016/S0076-6879(99)10037-5.10.1016/S0076-6879(99)10037-5
- Stoodley P, Cargo R, Rupp CJ, Wilson S, Klapper I. 2002. Biofilm material properties as related to shear-induced deformation and detachment phenomena. J Ind Microbiol Biotechnol. 29:361–367. doi: 10.1038/sj.jim.7000282.10.1038/sj.jim.7000282
- Sun JY, Zhao X, Illeperuma WR, Chaudhuri O, Oh KH, Mooney DJ, Vlassak JJ, Suo Z. 2012. Highly stretchable and tough hydrogels. Nature. 489:133–136. doi: 10.1038/nature11409.10.1038/nature11409
- Uppuluri P, Dinakaran H, Thomas DP, Chaturvedi AK, Lopez-Ribot JL. 2009. Characteristics of Candida albicans biofilms grown in a synthetic urine medium. J Clin Microbiol. 47:4078–4083. doi: 10.1128/JCM.01377-09.10.1128/JCM.01377-09
- Van Oss CJ, Chaudhury MK, Good RJ. 1987. Monopolar surfaces. Adv Colloid Interface Sci. 28:35–64. doi: 10.1016/0001-8686(87)80008-8.10.1016/0001-8686(87)80008-8
- Van Oss CJ, Good RJ, Chaudhury MK. 1986. The role of van der Waals forces and hydrogen bonds in “hydrophobic interactions” between biopolymers and low energy surfaces. J Colloid Interface Sci. 111:378–390. doi: 10.1016/0021-9797(86)90041-X.10.1016/0021-9797(86)90041-X
- Van Oss CJ, Good RJ, Chaudhury MK. 1988. Additive and nonadditive surface tension components and the interpretation of contact angles. Langmuir. 4:884–891. doi: 10.1021/la00082a018.10.1021/la00082a018
- Wang Y, Ameer GA, Sheppard BJ, Langer R. 2002. A tough biodegradable elastomer. Nat Biotechnol. 20:602–606. doi: 10.1038/nbt0602-602.10.1038/nbt0602-602
- Zanchi A, Chiolero A, Maillard M, Nussberger J, Brunner HR, Burnier M. 2004. Effects of the peroxisomal proliferator-activated receptor-gamma agonist pioglitazone on renal and hormonal responses to salt in healthy men. J Clin Endocrinol Metab. 89:1140–1145. doi: 10.1210/jc.2003-031526.10.1210/jc.2003-031526
- Zhao YP, Wang LS, Yu TX. 2003. Mechanics of adhesion in MEMS - a review. J Adhes Sci Technol. 17:519–546. doi: 10.1163/15685610360554393.10.1163/15685610360554393