Abstract
Biofilm formation and detachment in drinking water distribution systems (DWDS) can lead to several operational issues. Here, an alternative biofilm control strategy of limiting bacterial adhesion by application of a poly(N-isopropylmethacrylamide)-based nanogel coating on DWDS pipe walls was investigated. The nanogel coatings were successfully deposited on surfaces of four polymeric pipe materials commonly applied in DWDS construction. Nanogel-coated and non-coated pipe materials were characterized in terms of their surface hydrophilicity and roughness. Four DWDS relevant bacterial strains, representing Sphingomonas and Pseudomonas, were used to evaluate the anti-adhesive performance of the coating in 4 h adhesion and 24 h biofilm assays. The presence of the nanogel coating resulted in adhesion reduction up to 97%, and biofilm reduction up to 98%, compared to non-coated surfaces. These promising results motivate further investigation of nanogel coatings as a strategy for biofilm prevention in DWDS.
Graphical Abstract

Introduction
The purpose of drinking water distribution systems (DWDS) is to safeguard the transportation of potable water from a treatment plant to a consumer, and it is crucial that the water delivered to a tap is of high quality, both chemically and biologically. Nevertheless, this quality often deteriorates during the lengthy distribution process (Liu et al. Citation2017). Next to pipe corrosion and scales formation, biofilm development on DWDS pipe walls is one of the main causes of quality degradation (Liu et al. Citation2013; Makris et al. Citation2014). Biofilms are microbial aggregates formed on a surface that consist of cells embedded in a matrix of extracellular polymeric substances (EPS) secreted by the microbial community itself (Flemming et al. Citation2016). Their presence in DWDS leads to several operational issues. For instance, a biofilm adhering on the pipe walls can impact distribution process efficiency in the long term by pipe damage through microbially induced corrosion of iron-based pipes (Wang et al. Citation2015) or by increasing the flow resistance (Cowle et al. Citation2014). Moreover, the detachment of biofilm clusters into the water flow can lead to downstream deterioration of water aesthetics (turbidity, colour, smell) (Husband and Boxall Citation2011), and pose as a health hazard as biofilms were found to harbour pathogens (Wingender and Flemming Citation2011).
Globally, the biofilm control approach in DWDS often involves disinfection strategies via chlorination and chloramination (Al Abri et al. Citation2019; Li et al. Citation2021). However, chlorine is incapable of complete inhibition of biofilm growth, affecting only the periphery of the EPS matrix, and does not target microorganisms within the biofilm (Liu et al. Citation2016). Chlorine has limited penetration within the biofilm matrix due to its reaction with organic and inorganic compounds (Schwering et al. Citation2013); this reaction with organics also produces harmful disinfection by-products, which are suspected human carcinogens (Le Roux et al. Citation2017). Water companies that resigned from chlorination, focus on limiting the organic matter and nutrients essential for bacterial growth through improved water treatment (Park et al. Citation2021). Nevertheless, it has been reported that oligotrophic bacteria are still found to accumulate and form biofilms even when little to no molecules, neither organic nor inorganic, are present in the water stream (Kulakov et al. Citation2002). Common cleaning practices, such as periodic pipe flushing with increased water velocity, also do not completely remove the biofilm since they were found to mobilize and remove only loose biofilm clusters (Douterelo et al. Citation2013).
In this study, an alternative strategy to limit microbial adhesion to pipe materials utilized in DWDS with an anti-adhesive coating that targets biofilm formation in its onset was proposed. Antifouling solutions are already a recognized strategy in preventing biofilm development in numerous industrial applications, including those in membrane technologies (Zhao et al. Citation2021), the marine (Gu et al. Citation2020), and the biomedical field (Busscher et al. Citation2012). Such application offers an interesting alternative to DWDS biofilm control approaches, especially in DWDS biofilm hotspots, that are more prone to biofilm formation (higher temperatures, long stagnation periods, dead-ends) (Ling et al. Citation2018; Simunič et al. Citation2020).
Nanogels are nanosized hydrogel particles made of cross-linked swellable polymer networks with an ability to absorb and retain high volumes of water, without dissolving in aqueous media (Peppas and Hoffman Citation2020). When deposited on a surface in a form of a coating, in an aqueous environment, nanogel particles create a hydration layer, which targets biofilm formation in its early stages by creating a physical and energetical barrier for initial adhesion of bacteria (Chen et al. Citation2010, Keskin et al. Citation2019). Nowadays, nanogel coatings are gaining great attention to kill bacteria and to limit bacterial adhesion on biomaterial surfaces in order to avoid biomaterial-associated infections (Zu et al. Citation2020, Keskin et al. Citation2021). Nanogel coatings have also been used in the marine industry to prevent biofouling and the costly consequences for marine equipment (Chen et al. Citation2016). Proposed nanogel coating deposition on various surfaces is a universal, very simple and time-efficient method based on the electrostatic interactions between the solid surface and the nanogels, which can also be applied to water distribution pipe materials (Keskin et al. Citation2019).
While the nanogel coatings have been tested against adhesion of proteins (Cross et al. Citation2016), mammalian cells (Schmidt et al. Citation2010), and clinical bacterial strains (Keskin et al. Citation2019, Citation2020), at this moment, this is the first study that investigates their influence on initial adhesion and biofilm formation by environmentally relevant bacterial strains. DWDS conditions vary considerably from those in clinical settings, including oligotrophic environment, exposure to high water flows, and spatial-temporal fluctuations in environmental and engineering parameters throughout the distribution process (Potgieter et al. Citation2018). Such conditions promote development of microbial communities equipped with survival strategies and biofilm forming behaviours different from those exhibited by the clinically relevant strains. Therefore, the effect of the coating on DWDS-representative bacterial strains cannot be predicted from the results obtained by studies performed within biomedical settings. In this work, the poly(N-isopropylmethacrylamide)-based nanogel coating was deposited on a selection of polymeric pipe surfaces to validate the applicability of the coating on DWDS relevant materials. The nanogel coating was tested against the initial adhesion and the biofilm formation by four bacterial strains representing Sphingomonas and Pseudomonas genera commonly identified in DWDS biofilms. Bacterial adhesion was assessed by flowing bacterial suspensions in a parallel-plate flow chamber and by enumerating attached bacteria after 4 h. Moreover, the anti-biofilm performance of the coating was examined in a multi-well plate assay for 24 h on polyvinyl chloride (PVC) and high-density polyethylene (HDPE), materials commonly utilized in the construction of new DWDS.
Materials and methods
Nanogels synthesis, nanogel coating deposition and characterization
Reagents
The monomer N-isopropylmethacrylamide (97%, NIPMAM), the cross-linker N,N′-methylene bis(acrylamide) (99%, BIS), the surfactant sodium dodecyl sulfate (SDS), the initiator ammonium persulfate (98% APS) and polyethyleneimine (PEI, branched, Mw 25,000 g/mol) were purchased from Sigma-Aldrich, the Netherlands. N-Isopropylmethacrylamide was recrystallized from hexane; all other chemicals were used as received without purification. Ultrapure water (18.2 MΩ, arium 611 DI water purification system; Sartorius AG, Germany) was used in nanogels synthesis and coatings preparation.
Synthesis of P(NIPMAM) nanogel
Synthesis of the poly(N-isopropylmethacrylamide) (P(NIPMAM)) nanogel was performed as described previously by Keskin et al. (Citation2019), and the most optimal composition, µGel5, was selected for this study. Briefly, in a three-necked 250 mL flask equipped with a flat anchor-shaped mechanical stirrer, a reflux condenser, and a nitrogen in- and outlet, 1812 mg (4.8 mmol, 95 mol %) of NIPMAM (NIPMAM previously crystallized), 117 mg (0.25 mmol, 5 mol %) of BIS, 30 mg (0.7 mM) of SDS were dissolved in 135 mL of water, and the reaction mixture was degassed with N2 for 1 h and heated till 70 °C. The reaction was initiated by injecting a degassed initiator solution of 33 mg (0.05 mmol) APS in 15 mL water into the reaction mixture and the reaction was continued for another 4 h at 70 °C and 300 rpm under N2 atmosphere. After 4 h, the reaction mixture was cooled to room temperature (RT) and stirred overnight. The nanogel dispersion was purified by ultracentrifugation (3 times at 179,200 g) followed by decantation and dispersion in water and the P(NIPMAM) nanogel was freeze-dried for the preparation of the coatings.
Dynamic light scattering (DLS) and ζ-potential measurement
The hydrodynamic diameter (dh) and particle size distribution of the nanogels were measured using a Zetasizer Nano-ZS (Malvern Instruments, UK). Temperature-dependent measurements were recorded at a fixed scattering angle of 173 degrees and a wavelength λ = 633 nm of the laser beam while the temperature was varied in the range of 2 to 60 °C at 2 °C intervals and with a measurement time of 10 s and 11 runs, performed in triplicate. For data evaluation, the cumulant fit analysis was used and the hydrodynamic dh was calculated by use of the Stokes − Einstein equation. ζ-potential measurements were performed in disposable capillary cells (Malvern, DTS1070) in water at an angle of 17 degrees and a laser beam wavelength of λ = 633 nm. The ζ-potential was calculated from the electrophoretic mobility by use of the Smoluchowski equation.
Transmission electron microscopy (TEM)
To evaluate the size and the morphology of the nanogel particles, TEM analysis was performed using a Philips CM12 microscope operating at an accelerating voltage of 120 kV and coupled to a 4 k CCD camera. The nanogel particles were negatively stained with 2% uranyl acetate that were prepared by drop-casting of a nanogel suspension (0.2 mg/mL) in water onto a carbon coated copper grid.
Surface preparation and nanogel coating deposition
To test the nanogel coating applicability on pipe materials, the coating was deposited on coupons (8 mm x 8 mm) cut from polymeric pipes used in DWDS, such as high-density polyethylene PE100 (HDPE-1, HDPE-2) and unplasticized polyvinyl chloride (PVC-1, PVC-2). Additionally, nanogel coating was deposited on transparent polyvinyl chloride slides (transparent PVC; Eriks, the Netherlands) of 76 mm x 26 mm x 1 mm and used in a parallel-plate flow chamber for bacterial adhesion experiments, and on PVC-1 and HDPE-1 coupons of 25 mm x 25 mm for biofilm growth assay. The coating procedure has been described previously (Keskin et al. Citation2019) and was implemented here with slight modifications. Briefly, prior to coating, transparent PVC and pipe materials were rinsed with ethanol (70%) and water, sonicated for 10 min (Transsonic TP690, Salm en Kipp B.V., the Netherlands) in water and air-dried at RT. Plasma oxidation was performed for 5 min (at 100 mTorr and 0.2 mbar, on Plasma Active Flecto 10 USB, Plasma Technology GmbH, Germany) after which the solid surfaces were immersed in PEI solution (1.5 mg/mL, 0.15 wt%, pH 7) for 20 min and afterward rinsed five times with water. After drying at RT, nanogel suspension (5 mg/mL, 0.5 wt%) was sprayed onto the PEI modified surfaces until the nanogel solution was uniformly distributed. The coated surfaces were air dried first at RT followed by overnight drying in the oven at 50 °C. To remove the non-bound fraction of nanogel particles, the solid surfaces with the dried nanogel layer were washed three times by immersing in water for 2 h each time.
Atomic force microscopy (AFM) and average roughness measurements
The surface topographies of nanogel coatings on transparent PVC, HDPE-1, HDPE-2, PVC-1, and PVC-2 were visualized using AFM (Dimension Icon, Bruker, MA, USA) in contact mode with silicon nitride DNP-10 probes (spring constant k = 0.06 N/m, frequency f0 = 18 kHz) in a dry and wet state. Average roughness (RA) was calculated based on AFM images of non-coated and coated samples using NanoScope Analysis 2.0 image processing software (Bruker) on micrographs of 5 × 5 µm. RA measurements were performed on two different locations of three replicates per tested surface. To compare the roughness of non-coated and nanogel-coated pipe materials, Student’s t-test was used.
Water contact angle measurements
The wettability of non-coated and nanogel-coated surfaces was assessed by measuring advancing type water contact angles at RT using the sessile drop technique. Ultrapure water droplets of 1.5 µL were applied on the solid surfaces with and without a nanogel coating and measured with a contour analysis system (OCA35, DataPhysics Instruments, Germany). The measurements were performed on three different locations of three replicates per tested surface. All the samples were hydrated overnight before the measurement.
Nanogel coating stability
To test the stability of the nanogel coating, three coated and non-coated coupons (8 mm x 8 mm) of the four pipe materials were placed in a 24-well plate (Corning, NY, USA), with sterilized tap water and agitated on an orbital shaker (KS 260 basic, IKA, Germany) at 240 rpm at RT for 30 days. The presence of the nanogel coating, prior and after the stability test, was determined using AFM. Water, in which the coupons were immersed during the stability test, was analysed for the concentration of organic carbon using Liquid Chromatography-Organic Carbon Detection (LC-OCD) method to evaluate potential effect of the nanogel coating on the water quality. LC-OCD was performed using Model 8 (DOC-LABOR, Germany) and data analysis was performed with DOC-LABOR software (ChromLog version). To compare the organic carbon concentrations in water in contact with non-coated and nanogel-coated pipe materials, Student’s t-test was used.
Bacterial adhesion and biofilm formation experiments
Bacterial strains
Four bacterial strains belonging to Sphingomonas and Pseudomonas, genera commonly identified in DWDS biofilms communities (Douterelo et al. Citation2014; Liu et al. Citation2014; Citation2017), were selected for this study. Sphingomonas Sph5 and Sph10 were isolated from fouled spiral wound membranes and are part of the Wetsus institute collection (De Vries et al. Citation2019). Pseudomonas aeruginosa (DSM 50071) and Pseudomonas extremorientalis (DSM 15824) were purchased from the German Collection of Microorganisms and Cell Cultures GmbH (DSMZ, Germany). The bacteria were first grown on R2A agar (BD Difco, NJ, USA) plates at 30 °C over 72 h from frozen Viabank beads stock (Medical Wire, UK) that was stored at −80 °C. The plates were stored at 4 °C and were renewed every two weeks.
Parallel plate flow chamber experiments
For preparation of bacterial cultures, one colony was used to inoculate 10 mL R2A liquid growth medium (Dinkelberg Analytics GmbH, Germany) and grown overnight (18 h) at 30 °C under agitation (150 rpm). 0.5 mL of this pre-culture was used to inoculate 100 mL R2A medium , and the main culture was allowed to grow for 24 h. Bacteria were harvested by centrifugation (5000 g, 5 min, 10 °C) and washed three times with synthetic tap water (STW; 100 mg/L NaHCO3, 13 mg/L MgSO4·7 H2O, 0.7 mg/L K2HPO4, 0.3 mg/L KH2PO4, 0.01 mg/L (NH4)2SO4, 0.01 mg/L NaCl, 0.001 mg/L FeSO4·7 H2O, 1 mg/L NaNO3, 27 mg/L CaSO4, pH = 7.50 ± 0.05 (Gomes et al. Citation2019)) and sonicated on ice for 30 s at 30 W (Vibra Cell VCX130; Sonics and Materials Inc., CT, USA) to break bacterial aggregates. To prepare a bacterial suspension needed for the adhesion experiments, bacteria were counted using a Bürker-Türk counting chamber (Marienfeld, Germany) and the bacterial suspension was diluted to 3 x 108 bacteria/mL in STW.
Bacterial adhesion on nanogel-coated and non-coated transparent PVC was determined by triplicate experiments using a parallel plate flow chamber set-up (Figure S1) by flowing a bacterial suspension (3 x 108 bacteria/mL) at RT for 4 h at a shear rate of 10 s−1, as described previously (Busscher and Van der Mei Citation2006). The parallel plate flow chamber was mounted on a phase-contrast microscope BH-2 (Olympus, Japan), equipped with a 40x long-distance objective (Olympus ULWD-CD Plan 40 PL) (Figure S2). Prior to the experiment, the system was filled with STW to remove air bubbles. Transparent PVC was used as an alternative for non-transparent pipe materials to allow real-time observation of bacterial adhesion. The transparent PVC slide with or without a nanogel coating was mounted as a bottom plate in the parallel-plate flow chamber from which live images were acquired. After 4 h, images were taken on ten different locations on each transparent PVC slide. To enhance the signal-to-noise ratio and to eradicate flowing bacteria from the analysis, live images were generated after summation of 15 consecutive images (1 s intervals). The adhered bacteria were counted and expressed per cm2 using ImageJ Fiji software (https://imagej.net/software/fiji/) (Schindelin et al. Citation2012), automatically, or manually with the Cell Counter plugin, when the distinction of a single bacterium was not possible otherwise. As Sphingomonas Sph10 adhered as aggregates, the number of aggregates was determined based on the images obtained after 4 h adhesion. A minimum of three bacterial cells clumped together was defined as an aggregate. Similar to single cell quantification, the number of Sph10 aggregates was evaluated after three independent assays, each time using images collected from ten different locations on a coated and non-coated surface. To compare the number of adhered bacteria on non-coated and nanogel-coated transparent PVC, Student’s t-test was used.
Biofilm formation on DWDS materials
Biofilm formation was performed in 6-well plates (Falcon, Corning, NY, USA) on nanogel-coated and bare PVC-1 and HDPE-1 coupons, as they represented distinct surface properties in terms of hydrophilicity and roughness ( and ). Before the experiment, PVC-1 and HDPE-1 coupons (25 mm x 25 mm) were washed by rubbing with a commercial dish soap (Dreft, Procter & Gamble, OH, USA) solution, immersed in 70% ethanol for 10 min, rinsed with sterile ultrapure water and air dried in sterile environment at RT. Nanogel-coated coupons were immersed in 70% ethanol for 10 min, rinsed with sterile ultrapure water and air dried in sterile environment at RT. For the bacterial pre-culture, one colony was used to inoculate 50 mL R2A medium and grown for 24 h at 30 °C under agitation (150 rpm). The bacterial pre-cultures were diluted in R2A medium to obtain an optical density at 600 nm of 0.1 and distributed by 6 mL in each well in sterile environment, together with the coupons. The controls included bacterial suspensions without any coupon, material coupons in R2A medium, and R2A medium only. The plates were incubated for 24, 48 and 72 h in 30 °C under agitation (100 rpm). Since no relevant differences in bacterial growth and biofilm formation were observed between the three incubation times (Figure S3), all the experiments were run for 24 h before biofilm analysis. For each strain, three biological replicates were performed.
Figure 3. (A) Phase contrast microscope images of bacteria adhered to the surface of non-coated transparent PVC (top row) and nanogel-coated transparent PVC (bottom row) in a parallel-plate flow chamber after 4 h. Scale bars are 20 µm. (B) Number of adhered bacteria in the parallel-plate flow chamber after 4 h on non-coated and nanogel-coated transparent PVC. The values are averages of experiments performed on three separately coated surfaces with separately prepared bacterial cultures.
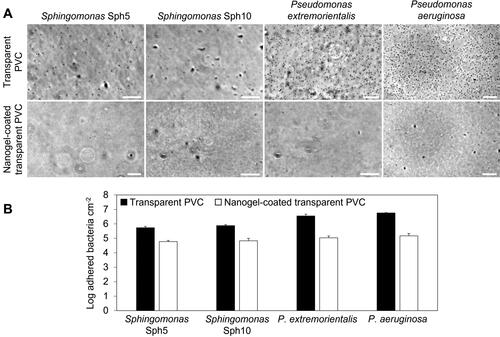
DAPI staining and fluorescence microscopy
To prepare the samples for the analysis, the coupons were removed from the wells and washed five times with phosphate-buffered saline (PBS; PanReac AppliChem, Germany) to remove non-adhered bacteria. Then, the coupons were immersed for 10 min in 4′,6-diamidino-2-phenylindole (DAPI, 1 µg/mL in PBS; Thermo Fisher Scientific, MA, USA) and washed in PBS again to remove excess staining. The samples were observed under an epi-fluorescence microscope BX43 (Olympus, Japan) equipped with an X-Cite 120Q UV-lamp (Excelitas Technologies, Canada). The images of adhered biomass were acquired with an Olympus DP80 camera and cellSense imaging software (Evident, Germany). Nine images per coupon were collected at 1000x magnification and the adhered bacteria were quantified and expressed as DAPI-positive area coverage (%) using ImageJ Fiji software. To compare the biofilms formed on non-coated and nanogel-coated pipe materials, Student’s t-test was used.
Results and discussion
Nanogels characterization
The synthesis of P(NIPMAM) nanogels was done by a precipitation polymerization method described earlier (Schmidt et al. Citation2010). To evaluate the nanogels stability in a range of temperatures, their behaviour was investigated by DLS in water (). Upon increasing the temperature, the nanogel hydrodynamic diameter (dh) decreased, since the volume phase transition temperature of the P(NIPMAM) nanogels is around 44 °C. The measured dh of the nanogel at 30 °C was 572 ± 5 nm, which is comparable with previously reported values (Keskin et al. Citation2019). Synthetized nanogels exhibited spherical shapes with an average diameter of 340 ± 23 nm according to TEM results (), which is lower than the diameter found by DLS analysis, since TEM is done in a dry state. Finally, the measured ζ-potential was −9.5 ± 0.1 mV, showing the net negative charge of the nanogel. Such negative charge of the nanogel particles potentially adds to the anti-adhesive performance of the coating, as negatively charged surfaces are known to repel bacteria through electrostatic repulsion, as bacterial cell walls are usually negatively charged themselves (Rzhepishevska et al. Citation2013).
Nanogel coating on polymeric pipe materials
Nanogel deposition was carried out on transparent PVC, and two brands of HDPE and PVC pipe materials, which were selected as surfaces of interest as they are a common selection for construction of new DWDS networks, due to their low price, light weight, and being non-corrosive (Wang et al. Citation2022). The polymeric surfaces were coated with P(NIPMAM) nanogel particles via spray-deposition and immobilization on a surface through electrostatic interactions with positively charged PEI as an anchoring polymer. Despite variations in topographies, AFM analysis confirmed that the nanogel coating procedure was successful on all selected pipe materials, and nanogel particles were tightly packed on their surfaces (), with only minor imperfections, where nanogel particles were missing (Figure S4). The thickness of the coating has been previously measured as the height of nanogel particles deposited on a surface by Keskin et al. (Citation2019), with a result of 30 ± 4 nm in a dry state and 57 ± 8 nm in a hydrated state. Hydrophilicity of the coated and uncoated polymeric samples was assessed here by water contact angle measurement (). The water contact angles were similar on all uncoated samples and pointing to a hydrophobic surface (89 ± 6 degrees), while the presence of the nanogel coating increased the hydrophilicity of pipe materials, as evaluated after overnight hydration, resulting in an average decrease of 44 ± 5 degrees in the water contact angles. Hydrophilicity is directly connected to surface free energy of a substratum, which is an important indicator in predicting bacterial adhesion. The higher the surface free energy, the more hydrophilic the surface will be, which at the molecular level will increase energetical demand for an adhering bacterium to replace water molecules bound with that surface (Carniello et al. Citation2018). Therefore, theoretically, the more hydrophilic the surface, the more energetically unfavorable the conditions will be for a bacterium to adhere. Moreover, in aqueous solutions, the nanogel coating absorbs and immobilizes water molecules, creating a hydration layer that weakens the interactions between bacterial cell wall and substratum, limiting the transition from reversible to irreversible adhesion, and thereby hinders biofilm formation (Chen et al. Citation2010; Carniello et al. Citation2018; Liu et al. Citation2021). Generally, the presence of the nanogel coating creates not only an energetical, but also a physical barrier between bacterial cells and the surface. Another important surface feature in terms of supporting biofilm formation is surface roughness. Rougher surfaces provide increased surface area available for bacterial adhesion and bacteria on such surfaces are shielded from the shear stresses and turbulences of bulk flow, which makes them more resistant to detachment (Cowle et al. Citation2014). In this study, the average surface roughness RA was measured using AFM. The selected pipe materials varied significantly, with transparent PVC (RA DRY = 4 ± 1 nm), HDPE-1 (16 ± 13 nm) and PVC-2 (14 ± 4 nm) being smoother, and HDPE-2 (32 ± 10 nm) and PVC-1 (61 ± 33 nm) rougher (). The presence of the nanogel coating had no significant influence (p > 0.05) on pipe materials roughness, neither in a dry nor a wet state, potentially due to its thin film nature.
Figure 4. (A) Micrographs of Sphingomonas Sph5 and Sphingomonas Sph10 adhered on non-coated (left column) and nanogel-coated transparent PVC (right column) after 4 h adhesion. Black arrows indicate Sph10 aggregates. Scale bars are 20 µm. (B) Number of Sphingomonas Sph10 aggregates adhered to the surface of non-coated and nanogel-coated transparent PVC after 4 h adhesion. The values are averages of experiments performed on three separately coated surfaces with separately prepared bacterial cultures. Note, no aggregates were observed for Sph5.
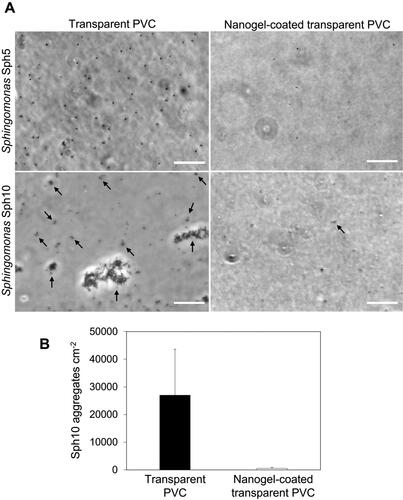
Table 1. Water contact angles of pipe materials without and with the nanogel coating.
Table 2. Average roughness (RA) of pipe materials without and with the nanogel coating in a hydrated (RA WET) and a dry (RA DRY) state.
The long-term stability of the coatings on HDPE-1, HDPE-2, PVC-1 and PVC-2 was evaluated after 30 days immersion in sterilized tap water by AFM analysis, which confirmed that the coating remained on all the surfaces, as shown in Figure S5. Additionally, the water in which the coupons were immersed did not show any significant (p > 0.05) increase in organic carbon concentrations (Figure S6). While 30 days are not yet representative of the real operational periods of DWDS pipework, as the pipework is installed with a purpose to serve for decades (Renaud et al. Citation2014), it provides an indication that the proposed protocol produces a coating which is structurally and chemically stable, and remains on the surface for an extended period of time. Moreover, the conditions that were found to negatively affect the nanogels stability, such as high temperature (above 45 °C), high salt concentration, and low pH (below 2.3) (Farooqi et al. Citation2017), are far from the average DWDS conditions. Temperatures in DWDS usually do not exceed 25 °C (Blokker and Pieterse-Quirijns Citation2013), and drinking water is of neutral pH and low salt content, making nanogel coatings suitable for potential long-term applications.
Figure 5. (A) Representative DAPI stained biomass images of Sphingomonas and Pseudomonas strains adhered on non-coated and nanogel-coated PVC-1 and HDPE-1 after 24 h. Scale bars are 10 µm. (B) Quantified area coverage by DAPI stained biomass of Sphingomonas and Pseudomonas strains adhered on non-coated and nanogel-coated PVC-1 and HDPE-1 after 24 h. The values are averages of experiments performed on six separately prepared non-coated and nanogel-coated surfaces with three separately prepared bacterial cultures. Asterisks indicate significance (*p < 0.05, **p < 0.01).
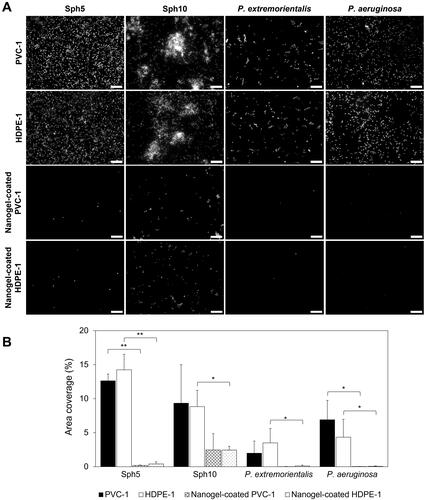
Bacterial adhesion on nanogel-coated and non-coated transparent PVC
The effectiveness of nanogel coatings in reducing bacterial adhesion on non-coated and nanogel-coated transparent PVC in a parallel-plate flow chamber was evaluated after 4 h for Sphingomonas Sph5 and Sph10, P. extremorientalis and P. aeruginosa. The bacterial strains were selected as representative genera for the initial adhesion tests since they are commonly found in DWDS biofilm communities (Hong et al. Citation2010; Douterelo et al. Citation2014; Liu et al. Citation2017; Chan et al. Citation2019). Sphingomonas spp. have been identified as primary surface colonizers and dominating species in many fouling phenomena, including those on spiral wound membranes (Bereschenko et al. Citation2010), and in membrane bioreactors (Huang et al. Citation2008). Moreover, Sphingomonas spp. are able to persist in oligotrophic environments (Vancanneyt et al. Citation2001; Ohta et al. Citation2004), and to resist chlorine (Sun et al. Citation2013), which makes them suitable candidates to proliferate in DWDS conditions. Presence and potential growth of P. aeruginosa, an opportunistic pathogen, is of special concern in DWDS (Bressler et al. Citation2009). P. extremorientalis was selected as a representative Pseudomonas specie since it was first isolated from a water reservoir (Ivanova et al. Citation2002).
The number of bacteria adhering on the nanogel-coated transparent PVC decreased compared to non-coated PVC (). The presence of the coating significantly (p < 0.005) reduced the number of adhered bacteria per unit area by approximately 1 to 1.5 log, namely by 88 ± 4%, 91 ± 3%, 97 ± 1% and 97 ± 1%, for Sph5, Sph10, P. extremorientalis and P. aeruginosa, respectively (), confirming the effectiveness of the coating in changing the surface properties. After 4 h, Pseudomonas strains adhered to the non-coated transparent PVC surface in significantly higher numbers than Sphingomonas strains (p < 0.05) ().
The same P(NIPMAM) nanogel coating also reduced adhesion of clinically relevant bacteria of Staphylococcus aureus by up to 99% in comparison to non-coated glass (Keskin et al. Citation2019). The lower efficiency against adhesion of Sphingomonas and Pseudomonas assessed in this study could possibly be a result of morphological outer layer differences between the used strains and S. aureus. Both Sphingomonas and Pseudomonas possess surface appendages (as also shown by SEM analysis, Figure S7), such as flagella, which is important in DWDS context, since motility is essential for nutrients scavenging in oligotrophic conditions (Du et al. Citation2020). Flagellum and other cell appendages (i.e. pili) can also serve as tethers piercing the energy barrier (Wang et al. Citation2011) and facilitate adhesion to those limited adhesion sites between the deposited nanogel particles or spaces formed due to occasional coating imperfections (Figure S4). In comparison, S. aureus, as a non-flagellated bacterium (Pollitt et al. Citation2015), solely depends on adhesion sites that are big enough to support the cell body attachment.
It is worth to notice that, despite representing the same genus, Sphingomonas Sph5 and Sph10 exhibited different adhesion behaviours. On non-coated transparent PVC, Sph5 adhered uniformly as single cells, and Sph10 as aggregates spontaneously pre-formed in a liquid phase (). This behaviour is in agreement with the initial characterization of the isolate provided by De Vries et al. (Citation2019), where Sph10 showed auto-aggregation of almost 100% of the cells in a liquid culture after 24 h. The nanogel coating significantly (p < 0.05) reduced the number of adhered Sph10 aggregates by 97 ± 2%, compared to bare transparent PVC (). Moreover, the aggregates detected on coated surfaces consisted of no more than four bacterial cells, while those attached on non-coated transparent PVC were multicellular clusters in sizes of up to 30 μm in diameter (). This might indicate that while the coating might provide occasional adhesion sites to support (tethered) attachment of single cells, as observed for Sph5 and Pseudomonas strains, these sites are not big enough to hold big multicellular clusters under the applied flow conditions. It is important in the context of the discussed future coating application, as microbial aggregates play a considerable role in initial biofilm development stages in natural environments, and their adhesion can initiate biofilm formation in a more rapid way than adhesion of single cells (Kragh et al. Citation2016).
Biofilm formation on nanogel-coated and non-coated polymeric pipe materials
The performance of the nanogel coating was further evaluated against the selected bacterial strains in conditions favourable for biofilm formation in 6-well plates. Conditions in the plate assay were similar to those in DWDS sections with low hydrodynamic stress such as dead-ends, corners, valves or areas with low water consumption, which are also prone to increased nutrients levels in water due to stagnation, accumulation of sediments, and pipe damage (Fernandes et al. Citation2019). After 24 h biofilm formation, fluorescence microscopy images showed differences in bacterial distribution on the surface between the strains (). Similarly as in the initial adhesion experiments, the biofilm forming tendencies varied between the four strains. Sph5 and P. aeruginosa single cells were homogenously distributed, Sph10 formed extensive multilayer bacterial aggregates, and P. extremorientalis formed small monolayer clusters on the surface. Nanogel coating significantly reduced the number of adhered bacteria, as visible in , and as confirmed by the quantification of attached biomass determined by DAPI staining in . For Sph5, P. extremorientalis and P.aeruginosa the presence of the coating led to significant (p < 0.05) reduction in adhered biomass of average 98 ± 1%. For Sph10 the reduction was lower, as it reached 65 ± 38% (p > 0.05) and 70 ± 13% (p < 0.05) for PVC-1 and HDPE-1, respectively. However, while Sph10 was present on non-coated surfaces as three-dimensional bacterial aggregates with a diameter up to 30 µm, on nanogel-coated surfaces, bacteria were mainly present as single cells or small two-dimensional clusters (). It is expected that for Sph10 the biomass adhered to the non-coated pipe material surfaces, and thereby also the anti-adhesive efficiency of the coating, were underestimated due to the bacterial aggregates. Epi-fluorescence microscopy provides only a two-dimensional plane imaging, which is not taking the three dimensional nature of aggregates into account (Costa et al. Citation2013).
Aspects of nanogel coatings as an alternative biofilm control strategy for DWDS
The purpose of this study was to introduce the concept of P(NIPMAM)-based nanogel coating application as an alternative control strategy for biofilm formation and regrowth in water distribution and storage systems via limiting the microbial attachment to the surfaces. As presented, the nanogel coating showed promising results in limiting bacterial adhesion and biofilm formation, and appears to be a versatile solution to be applied on various materials utilized in DWDS. Moreover, in line with the proposed application area, nanogel-based coatings present numerous advantages. As being soft deformable colloidal nanoparticles, nanogels can adhere to the surface and create a homogeneous thin coating. Compared to other chemical coating procedures that require grafting or covalent binding (Schmidt et al. Citation2010), nanogel coating deposition presented in this work depends on physical adsorption, and nanogels can be easily applied to a surface by spraying. Spray-deposition creates a considerable advantage in the manufacturing process, especially when applied on oddly shaped substrata, such as inner surface of a pipe. The polyacrylamides used to synthetize the nanogels are generally considered non-toxic/non-hazardous (Andersen Citation2005), they are commonly selected for biomedical applications, due to their biocompatibility (Naha et al. Citation2010; Guo et al. Citation2017; Capella et al. Citation2019), and are also utilized as a coagulating agent in water treatment (Ma et al. Citation2019; Long et al. Citation2020). Also, if DWDS sections are considered to be the future coating target, despite significantly larger scale and surface area to coat, the nanogel coating showed to be effective as a thin monolayer, which volume-wise requires only little amounts of nanogel particles per surface unit. For instance, to coat 100 m of a 110 mm diameter pipe, only 1.1 g of P(NIPMAM) nanogels would be needed. Considering that residual concentrations of acrylamide in its polymerized form range from <0.01 to 0.1% (Andersen Citation2005) and that big volumes of water are distributed daily, any potential sudden or gradual erosion of the coating components will not result in acrylamide concentration exceeding the guideline value of 0.1 µg/L (Council Directive 98/83/EC Citation1998). Together with the high efficiency in limiting bacterial adhesion, nanogel coatings present an interesting alternative for biofilm control in DWDS, with a potential focus on biofilm hotspots characterized by low hydrodynamic stress, long stagnation periods and higher nutrients concentrations.
Until now, the potential of nanogel coatings to limit bacterial adhesion has been investigated in the biomedical field, where they are proposed as an anti-biofilm strategy for in-body devices and have been tested against adhesion of medically relevant strains, such as S. aureus. This study showed that the nanogel coating can have a much broader application, and it can be successfully applied to a variety of polymeric pipe materials, such as PVC or HDPE, that are commonly used for the construction of new DWDS pipelines. Obtained results show promise for the potential application of P(NIPMAM)-based nanogel coating against adhesion of drinking water bacteria. However, translation of the technology from biomedical application to DWDS circumstances will require further investigation, with a focus on long-term stability, especially in DWDS hydraulic conditions, robustness, antifouling efficiency against complex, multi-species microbial communities, and any potential long-term exposure health implications.
Supplemental Material
Download PDF (1.3 MB)Acknowledgments
The authors thank the participants of the research theme ‘Biofilms’ for the help, fruitful discussions and financial support. The authors want to thank Clio Siebenmorgen for TEM imaging of nanogel particles.
Disclosure statement
P.v.R. is also a co-founder, scientific advisor, and shareholder in BiomACS BV, a biomedical-oriented screening company. The authors declare no other conflict of interest.
Additional information
Funding
References
- Al Abri M, Al-Ghafri B, Bora T, Dobretsov S, Dutta J, Castelleto S, Rosa L, Borettu A. 2019. Chlorination disadvantages and alternative routes for biofouling control in reverse osmosis desalination. Npj Clean Water. 2:2. doi:10.1038/s41545-018-0024-8
- Andersen FA. 2005. Amended final report on the safety assessment of polyacrylamide and acrylamide residues in cosmetics. Int J Toxicol. 24 Suppl:21–50. doi:10.1080/10915810590953842
- Bereschenko LA, Stams AJM, Euverink GJW, Van Loosdrecht MCM. 2010. Biofilm formation on reverse osmosis membranes is initiated and dominated by Sphingomonas spp. Appl Environ Microbiol. 76:2623–2632. doi:10.1128/AEM.01998-09
- Blokker EJM, Pieterse-Quirijns EJ. 2013. Modeling temperature in the drinking water distribution system. J Am Water Works Assoc. 105: E19–E28. doi:10.5942/jawwa.2013.105.0011
- Bressler D, Balzer M, Dannehl A, Flemming HC, Wingender J. 2009. Persistence of Pseudomonas aeruginosa in drinking-water biofilms on elastomeric material. Water Supply. 9:81–87. doi:10.2166/ws.2009.026
- Busscher HJ, Van der Mei HC. 2006. Microbial adhesion in flow displacement systems. Clin Microbiol Rev. 19:127–141. doi:10.1128/CMR.19.1.127-141.2006
- Busscher HJ, Van der Mei HC, Subbiahdoss G, Jutte PC, Van den Dungen JJ, Zaat SA, Schultz MJ, Grainger DW. 2012. Biomaterial-associated infection: locating the finish line in the race for the surface. Sci Transl Med. 4:153rv10. doi:10.1126/scitranslmed.3004528
- Capella V, Rivero RE, Liaudat AC, Ibarra LE, Roma DA, Alustiza F, Mañas F, Barbero CA, Bosch P, Rivarola CR, et al. 2019. Cytotoxicity and bioadhesive properties of poly-N-isopropylacrylamide hydrogel. Heliyon. 5:e01474. doi:10.1016/j.heliyon.2019.e01474
- Carniello V, Peterson BW, Van der Mei HC, Busscher HJ. 2018. Physico-chemistry from initial bacterial adhesion to surface-programmed biofilm growth. Adv Colloid Interface Sci. 261:1–14. doi:10.1016/j.cis.2018.10.005
- Chan S, Pullerits K, Keucken A, Persson KM, Paul CJ, Rådström P. 2019. Bacterial release from pipe biofilm in a full-scale drinking water distribution system. Npj Biofilms Microbiomes. 5:1–8. doi:10.1038/s41522-019-0082-9
- Chen K, Zhou S, Wu L. 2016. Self-healing underwater superoleophobic and antibiofouling coatings based on the assembly of hierarchical microgel spheres. ACS Nano. 10:1386–1394. doi:10.1021/acsnano.5b06816
- Chen S, Li L, Zhao C, Zheng J. 2010. Surface hydration: principles and applications toward low-fouling/nonfouling biomaterials. Polymer. 51:5283–5293. doi:10.1016/j.polymer.2010.08.022
- Costa JC, Mesquita DP, Amaral AL, Alves MM, Ferreira EC. 2013. Quantitative image analysis for the characterization of microbial aggregates in biological wastewater treatment: a review. Environ Sci Pollut Res. 20:5887–5912. doi:10.1007/s11356-013-1824-5
- Council Directive 98/83/EC of 3 November 1998 on the Quality of water intended for human consumption 1998. OJ L 330, 5.12, 32–35.
- Cowle MW, Babatunde AO, Rauen WB, Bockelmann-Evans BN, Barton AF. 2014. Biofilm development in water distribution and drainage systems: dynamics and implications for hydraulic efficiency. Environ Technol Rev. 3:31–47. doi:10.1080/09593330.2014.923517
- Cross MC, Toomey RG, Gallant ND. 2016. Protein-surface interactions on stimuli-responsive polymeric biomaterials. Biomed Mater. 11. doi:10.1088/1748-6041/11/2/022002
- De Vries HJ, Beyer F, Jarzembowska M, Lipińska J, Van den Brink P, Zwijnenburg A, Timmers PHA, Stams AJM, Plugge CM. 2019. Isolation and characterization of Sphingomonadaceae from fouled membranes. Npj Biofilms Microbiomes. 5:1–9. doi:10.1038/s41522-018-0074-1
- Douterelo I, Husband S, Boxall JB. 2014. The bacteriological composition of biomass recovered by flushing an operational drinking water distribution system. Water Res. 54:100–114. doi:10.1016/j.watres.2014.01.049
- Douterelo I, Sharpe RL, Boxall JB. 2013. Influence of hydraulic regimes on bacterial community structure and composition in an experimental drinking water distribution system. Water Res. 47:503–516. doi:10.1016/j.watres.2012.09.053
- Du B, Gu Y, Chen G, Wang G, Liu L. 2020. Flagellar motility mediates early-stage biofilm formation in oligotrophic aquatic environment. Ecotoxicol Environ Saf. 194. doi:10.1016/j.ecoenv.2020.110340
- Farooqi ZH, Khan HU, Shah SM, Siddiq M. 2017. Stability of poly (N-isopropylacrylamide-co-acrylic acid) polymer microgels under various conditions of temperature, pH and salt concentration. Arab J Chem. 10:329–335. doi:10.1016/j.arabjc.2013.07.031
- Fernandes S, Simões LC, Lima N, Simões M. 2019. Adhesion of filamentous fungi isolated from drinking water under different process conditions. Water Res. 164:114951. doi:10.1016/j.watres.2019.114951
- Flemming HC, Wingender J, Szewzyk U, Steinberg P, Rice SA, Kjelleberg S. 2016. Biofilms: an emergent form of bacterial life. Nat Rev Microbiol. 14:563–575. doi:10.1038/nrmicro.2016.94
- Gomes IB, Simões LC, Simões M. 2019. The role of surface copper content on biofilm formation by drinking water bacteria. RSC Adv. 9:32184–32196. doi:10.1039/C9RA05880J
- Gu Y, Yu L, Mou J, Wu D, Xu M, Zhou P, Ren Y. 2020. Research strategies to develop environmentally friendly marine antifouling coatings. Mar Drugs. 18:371. doi:10.3390/md18070371
- Guo Z, Li S, Wang C, Xu J, Kirk B, Wu J, Liu Z, Xue W. 2017. Biocompatibility and cellular uptake mechanisms of poly(N-isopropylacrylamide) in different cells. J Bioact Compat Polym. 32:17–31. doi:10.1177/0883911516648969
- Hong PY, Hwang C, Ling F, Andersen GL, LeChevallier MW, Liu W-T. 2010. Pyrosequencing analysis of bacterial biofilm communities in water meters of a drinking water distribution system. Appl Environ Microbiol. 76:5631–5635. doi:10.1128/AEM.00281-10
- Huang LN, De Wever H, Diels L. 2008. Diverse and distinct bacterial communities induced biofilm fouling in membrane bioreactors operated under different conditions. Environ Sci Technol. 42:8360–8366. doi:10.1021/es801283q
- Husband PS, Boxall JB. 2011. Asset deterioration and discolouration in water distribution systems. Water Res. 45:113–124. doi:10.1016/j.watres.2010.08.021
- Ivanova EP, Gorshkova NM, Sawabe T, Hayashi K, Kalinovskaya NI, Lysenko AM, Zhukova NV, Nicolau DV, Kuznetsova TA, Mikhailov VV, et al. 2002. Pseudomonas extremorientalis sp. nov., isolated from a drinking water reservoir. Int J Syst Evol Microbiol. 52:2113–2120. doi:10.1099/00207713-52-6-2113
- Keskin D, Mergel O, Van der Mei HC, Busscher HJ, Van Rijn P. 2019. Inhibiting bacterial adhesion by mechanically modulated microgel coatings. Biomacromolecules. 20:243–253. doi:10.1021/acs.biomac.8b01378
- Keskin D, Tromp L, Mergel O, Zu G, Warszawik E, Van der Mei HC, Van Rijn P. 2020. Highly efficient antimicrobial and antifouling surface coatings with triclosan-loaded nanogels. ACS Appl Mater Interfaces. 12:57721–57731. doi:10.1021/acsami.0c18172
- Keskin D, Zu G, Forson AM, Tromp L, Sjollema J, Van Rijn P. 2021. Nanogels: a novel approach in antimicrobial delivery systems and antimicrobial coatings. Bioact Mater. 6:3634–3657. doi:10.1016/j.bioactmat.2021.03.004
- Kragh KN, Hutchison JB, Melaugh G, Rodesney C, Roberts AEL, Irie Y, Jensen PØ, Diggle SP, Allen RJ, Gordon V, et al. 2016. Role of multicellular aggregates in biofilm formation. mBio. 7:e00237-16. doi:10.1128/mBio.00237-16
- Kulakov LA, McAlister MB, Ogden KL, Larkin MJ, O'Hanlon JF. 2002. Analysis of bacteria contaminating ultrapure water in industrial systems. Appl Environ Microbiol. 68:1548–1555. doi:10.1128/AEM.68.4.1548-1555.2002
- Le Roux J, Plewa MJ, Wagner ED, Nihemaiti M, Dad A, Croué JP. 2017. Chloramination of wastewater effluent: toxicity and formation of disinfection byproducts. J Environ Sci (China). 58:135–145. doi:10.1016/j.jes.2017.04.022
- Li W, Li Y, Wang F, Chen S, Chen J. 2021. Flow cytometric assessment of the chlorine/chloramine efficacy of particle-associated bacteria in drinking water. Environ Technol. 0:1–9. doi:10.1080/09593330.2021.1918263
- Ling F, Whitaker R, LeChevallier MW, Liu WT. 2018. Drinking water microbiome assembly induced by water stagnation. ISME J. 12:1520–1531. doi:10.1038/s41396-018-0101-5
- Liu G, Tao Y, Zhang Y, Lut M, Knibbe WJ, Van der Wielen P, Liu W, Medema G, Van der Meer W. 2017. Hotspots for selected metal elements and microbes accumulation and the corresponding water quality deterioration potential in an unchlorinated drinking water distribution system. Water Res. 124:435–445. doi:10.1016/j.watres.2017.08.002
- Liu G, Verberk JQJC, Van Dijk JC. 2013. Bacteriology of drinking water distribution systems: an integral and multidimensional review. Appl Microbiol Biotechnol. 97:9265–9276. doi:10.1007/s00253-013-5217-y
- Liu J, Qu S, Suo Z, Yang W. 2021. Functional hydrogel coatings. Natl. Sci. 8:. doi:10.1093/nsr/nwaa254
- Liu R, Zhu J, Yu Z, Joshi D, Zhang H, Lin W, Yang M. 2014. Molecular analysis of long-term biofilm formation on PVC and cast iron surfaces in drinking water distribution system. J Environ Sci (China). 26:865–874. doi:10.1016/S1001-0742(13)60481-7
- Liu S, Gunawan C, Barraud N, Rice SA, Harry EJ, Amal R. 2016. Understanding, monitoring, and controlling biofilm growth in drinking water distribution systems. Environ Sci Technol. 50:8954–8976. doi:10.1021/acs.est.6b00835
- Long Y, You X, Chen Y, Hong H, Liao BQ, Lin H. 2020. Filtration behaviors and fouling mechanisms of ultrafiltration process with polyacrylamide flocculation for water treatment. Sci Total Environ. 703:135540. doi:10.1016/j.scitotenv.2019.135540
- Ma J, Wang R, Wang X, Zhang H, Zhu B, Lian L, Lou D. 2019. Drinking water treatment by stepwise flocculation using polysilicate aluminum magnesium and cationic polyacrylamide. J Environ Chem Eng. 7:103049. doi:10.1016/j.jece.2019.103049
- Makris KC, Andra SS, Botsaris G. 2014. Pipe scales and biofilms in drinking-water distribution systems: undermining finished water quality. Crit Rev Environ Sci Technol. 44:1477–1523. doi:10.1080/10643389.2013.790746
- Naha PC, Bhattacharya K, Tenuta T, Dawson KA, Lynch I, Gracia A, Lyng FM, Byrne HJ. 2010. Intracellular localisation, geno- and cytotoxic response of poly-N-isopropylacrylamide (PNIPAM) nanoparticles to human keratinocyte (HaCaT) and colon cells (SW 480). Toxicol Lett. 198:134–143. doi:10.1016/j.toxlet.2010.06.011
- Ohta H, Hattori R, Ushiba Y, Mitsui H, Ito M, Watanabe H, Tonosaki A, Hattori T. 2004. Sphingomonas oligophenolica sp. nov., a halo- and organo-sensitive oligotrophic bacterium from paddy soil that degrades phenolic acids at low concentrations. Int J Syst Evol Microbiol. 54:2185–2190. doi:10.1099/ijs.0.02959-0
- Park J, Noh JH, Duong TH, Chung SY, Son H, Prest E, Oh S, Maeng SK. 2021. Occurrences and changes in bacterial growth-promoting nutrients in drinking water from source to tap: a review. Environ Sci: Water Res Technol. 7:2206–2222. doi:10.1039/D1EW00514F
- Peppas NA, Hoffman AS. 2020. Hydrogels. In: Wagner WR, Sakiyama-Elbert SE, Zhang G, Yaszemski MJ, editors. Biomaterials science. 4th ed. Cambridge, MA: Academic Press; p. 153–166. doi:10.1016/B978-0-12-816137-1.00014-3
- Pollitt EJG, Crusz SA, Diggle SP. 2015. Staphylococcus aureus forms spreading dendrites that have characteristics of active motility. Sci Rep. 5:17698. doi:10.1038/srep17698
- Potgieter S, Pinto A, Sigudu M, Du Preez H, Ncube E, Venter S. 2018. Long-term spatial and temporal microbial community dynamics in a large-scale drinking water distribution system with multiple disinfectant regimes. Water Res. 139:406–419. doi:10.1016/j.watres.2018.03.077
- Renaud E, Bremond B, Le Gat Y. 2014. Water pipes: why ‘lifetime’ is not an adequate concept on which to base pipe renewal strategies. Water Pract Technol. 9:307–315. doi:10.2166/wpt.2014.032
- Rzhepishevska O, Hakobyan S, Ruhal R, Gautrot J, Barbero D, Ramstedt M. 2013. The surface charge of anti-bacterial coatings alters motility and biofilm architecture. Biomater Sci. 1:589–602. doi:10.1039/C3BM00197K
- Schindelin J, Arganda-Carreras I, Frise E, Kaynig V, Longair M, Pietzsch T, Preibisch S, Rueden C, Saalfeld S, Schmid B, et al. 2012. Fiji: an open-source platform for biological-image analysis. Nat Methods. 9:676–682. doi:10.1038/nmeth.2019
- Schmidt S, Zeiser M, Hellweg T, Duschl C, Fery A, Möhwald H. 2010. Adhesion and mechanical properties of PNIPAM microgel films and their potential use as switchable cell culture substrates. Adv Funct Mater. 20:3235–3243. doi:10.1002/adfm.201000730
- Schwering M, Song J, Louie M, Turner RJ, Ceri H. 2013. Multi-species biofilms defined from drinking water microorganisms provide increased protection against chlorine disinfection. Biofouling. 29:917–928. doi:10.1080/08927014.2013.816298
- Simunič U, Pipp P, Dular M, Stopar D. 2020. The limitations of hydrodynamic removal of biofilms from the dead-ends in a model drinking water distribution system. Water Res. 178:115838. doi:10.1016/j.watres.2020.115838
- Sun W, Liu W, Cui L, Zhang M, Wang B. 2013. Characterization and identification of a chlorine-resistant bacterium, Sphingomonas TS001, from a model drinking water distribution system. Sci Total Environ. 458–460:169–175. doi:10.1016/j.scitotenv.2013.04.030
- Vancanneyt M, Schut F, Snauwaert C, Goris J, Swings J, Gottschal JC. 2001. Sphingomonas alaskensis sp. nov., a dominant bacterium from a marine oligotrophic environment. Int J Syst Evol Microbiol. 51:73–79. doi:10.1099/00207713-51-1-73
- Wang H, Hu C, Han L, Yang M. 2015. Effects of microbial cycling of Fe(II)/Fe(III) and Fe/N on cast iron corrosion in simulated drinking water distribution systems. Corros Sci. 100:599–606. doi:10.1016/j.corsci.2015.08.037
- Wang H, Sodagari M, Chen Y, He X, Newby BZ, Ju LK. 2011. Initial bacterial attachment in slow flowing systems: effects of cell and substrate surface properties. Biointerfaces. 87:415–422. doi:10.1016/j.colsurfb.2011.05.053
- Wang H, Yu P, Schwarz C, Zhang B, Huo L, Shi B, Alvarez PJJ. 2022. Phthalate esters released from plastics promote biofilm formation and chlorine resistance. Environ Sci Technol. 56:1081–1090. doi:10.1021/acs.est.1c04857.34991317
- Wingender J, Flemming HC. 2011. Biofilms in drinking water and their role as reservoir for pathogens. Int J Hyg Environ Health. 214:417–423. doi:10.1016/j.ijheh.2011.05.009
- Zhao S, Liao Z, Fane A, Li J, Tang C, Zheng C, Lin J, Kong L. 2021. Engineering antifouling reverse osmosis membranes: a review. Desalination. 499:114857. doi:10.1016/j.desal.2020.114857
- Zu G, Steinmüller M, Keskin D, van der Mei HC, Mergel O, van Rijn P. 2020. Antimicrobial nanogels with nanoinjection capabilities for delivery of the hydrophobic antibacterial agent triclosan. ACS Appl Polym Mater. 2:5779–5789. doi:10.1021/acsapm.0c01031