Abstract
Objective: Septic patients are especially vulnerable to acute lung injury (ALI). Calycosin (CAL) has various promising pharmacological activities. This paper aims to expound on the role of CAL in mice with sepsis-induced ALI and the associated mechanisms.
Methods: Mouse models of sepsis-induced ALI were established using lipopolysaccharide (LPS). Pulmonary histopathological changes were observed by HE staining. Cell apoptosis was assessed by TUNEL staining. Pulmonary edema was evaluated by measuring wet/dry weight. Bronchoalveolar lavage fluid (BALF) was collected to count inflammatory cells. In vitro LPS models were established using MLE-12 cells. miR-375-3p expression was determined by RT-qPCR. Cell viability and apoptosis were assessed by MTT assay and flow cytometry. Levels of inflammatory cytokines were determined by ELISA. The target relationship between miR-375-3p and ROCK2 was analyzed by the dual-luciferase assay. ROCK2 protein level was determined by Western blot.
Results: miR-375-3p was weakly-expressed in mice with sepsis-induced ALI, and CAL treatment elevated miR-375-3p expression. CAL treatment mitigated pulmonary tissue damage and edema, decreased apoptosis and inflammatory cells, downregulated levels of pro-inflammatory cytokines, and upregulated levels of anti-inflammatory cytokines in mice with sepsis-induced ALI. CAL treatment increased MLE-12 cell viability and decreased apoptosis and inflammation in MLE-12 cells. Inhibition of miR-375-3p partially abrogated CAL-mediated protective action on MLE-12 cells. miR-375-3p attenuated LPS-induced MLE-12 cell injury by targeting ROCK2.
Conclusion: CAL upregulates miR-375-3p to target ROCK2, thus protecting against sepsis-induced ALI in mice.
Introduction
Acute lung injury (ALI) refers to a common critical disease that can further develop into acute respiratory distress syndrome (ARDS) and clinically manifests with progressive respiratory failure and refractory hypoxemia [Citation1]. ALI leads to severe lung tissue damage and even irreversible pulmonary damage in severe cases, which ultimately results in death, significant morbidity, and horrific mortality [Citation2, Citation3]. The incidence of ALI is striking as well, accounting for 10% of intensive care unit admissions and 4% of all hospital admissions [Citation4]. Clinically, ALI patients often manifest with cyanosis refractory to oxygen, tachypnea, diffuse alveolar infiltrates, and decreased lung compliance [Citation5]. Although a better pathological understanding of ALI has been reached, the supportive treatment together with a protective pulmonary mechanical ventilation strategy continues to be the only option showing a survival perspective [Citation6]. Hence, new therapies, therapeutic targets, and antibiotics are in urgent need to combat ALI.
Unlike the commonly used drugs such as ulinastatin, dexamethasone, prednisolone, and prednisone with potential side effects, natural compounds possess underlying benefits in ALI treatment [Citation7]. Natural products are crucial sources of drugs and drug components with advantages such as low toxicity and few side reactions in ALI treatment [Citation8]. Calycosin (CAL) is an isoflavone phytoestrogen obtained from Astralagus membranaceus and has anti-oxidant, anti-inflammation, anti-tumor, and neuroprotective activities [Citation9]. CAL has been shown to ameliorate airway epithelial cell injury induced by exposure to PM 2.5 by activating the AMPK pathway [Citation10]. Emerging evidence suggests that CAL exerts a therapeutic effect on ALI either by inhibiting the activation of mitochondrial ROS-mediated inflammasome or the activation of NLRP3 inflammasome and the HMGB1/MyD88/NF-κB pathway [Citation11, Citation12]. However, the molecular mechanism of CAL in ALI remains insufficiently understood.
microRNAs (miRNAs) refer to short RNA molecules containing 19–25 nucleotides that modulate the silencing of target genes at the post-transcriptional level [Citation13]. Several miRNAs act as biomarkers for ALI and ingredients with application values in biopharmaceuticals [Citation14]. For example, miR-27a can mitigate lipopolysaccharide (LPS)-induced ALI in mice by reducing inflammation and cell apoptosis through the TLR4/MyD88/NF-κB pathway [Citation15]. miR-34b-5p downregulation extenuates pulmonary inflammation and cell apoptosis in mice with LPS-induced ALI by targeting progranulin [Citation16]. Novel evidence suggests that miR-375-3p contributes to the alleviation of myocardial injury in septic rats [Citation17]. Moreover, miR-375-3p plays an important role in regulating the function of pulmonary microvascular endothelial cells to maintain normal gas exchange and is potentially involved in the progression of lung diseases [Citation18]. Hence, we assumed that CAL might ameliorate ALI in mice by manipulating miR-375-3p expression. Rho-associated coiled-coil-containing protein kinase 2 (ROCK2) is a key regulatory factor of immunoregulation and fibrosis, and the activation of the Rho/ROCK pathway leads to inflammatory responses and has been confirmed to play a critical role in ALI [Citation19]. Multiple studies have disclosed that silencing ROCK2 relieves LPS-triggered ALI [Citation20, Citation21]. Since the research on the interaction among CAL, miR-375-3p, and ROCK2 in LPS-induced ALI is rare, this article is devised to expound on the effects of CAL on LPS-induced ALI via miR-375-3p/ROCK2 and the associated mechanism, in anticipation of offering theoretical references and novel therapeutic strategies for managing ALI.
Materials and methods
Ethics statement
Animal-associated experimental procedures were approved by the Animal Ethics Committee of People’s Hospital Affiliated with the Fujian University of Chinese Medicine in compliance with the ARRIVE guidelines and relevant regulations and guidelines. All animals were given humane care.
Experimental animals
Seventy-two male C57BL/6N mice (18–22 g, 8–10 wk) (Charles River, Beijing, China) were raised in a specific pathogen-free room at 22 ± 2 °C with 50 ± 10% humidity and a 12-h light/dark cycle, and provided with ad libitum standard rodent chow and clean water.
Establishment of ALI mouse models and animal grouping
The ALI mouse models were established by LPS (#L2630, Sigma-Aldrich, St. Louis, MO, USA) and oleic acid (OA, #O1008, Sigma-Aldrich) [Citation22] treatment. The 4% OA solution was prepared using 0.1% bovine serum albumin. Mice were allocated at random to 6 groups (N = 12): 1) control group, 2) LPS group, 3) LPS + 12.5 mg/kg CAL (#B9938, purity > 98%, Sigma-Aldrich) group, 4) LPS + 25 mg/kg CAL group, 5) LPS + 50 mg/kg CAL group, and 6) LPS + 100 mg/kg CAL group. ALI was induced by intraperitoneally injecting mice with 10 mg/kg LPS and intraperitoneally injecting 2.6 μL/g OA into mice 30 min later. The 1 mg/mL mother solution was prepared by dissolving 1 mg CAL in 1 mL of normal saline and then diluted to gradient concentrations for subsequent experimentation. Mice in the CAL treatment groups were treated with CAL gavage at 0 and 6 h following LPS treatment. Mice in the control and LPS groups were treated with normal saline only. Mice were euthanized 12 h after LPS administration, and lung tissues were removed for further experimentation. The left lungs of 6 random mice from each group were used for histological staining, and the right lungs for measurement of wet-dry (W/D) weight ratio, the lung tissues of the remaining mice in each group were used to collect broncho-alveolar lavage fluid (BALF) for reverse transcription-quantitative polymerase chain reaction (RT-qPCR) and Western blot.
Hematoxylin-eosin (HE) staining
The lung tissues were fixed in 4% formaldehyde, embedded in paraffin, and cut into 5-μm sections. Subsequently, the sections were placed on slides and subjected to HE staining, followed by dehydration with ethanol of gradient concentrations, clearing with xylene, resin sealing, and observation using a microscope (200×, Olympus, Tokyo, Japan). The overall histopathological scores were graded as follows [Citation23]: 0, no lesion; 1, minimal lesion with less than 10% area involved; 2, mild lesion with 10%–30% area involved; 3, moderate lesion with 30%–50% area involved; 4, marked lesion with 50%–80% area involved; 5, severe lesion with more than 80% area involved.
Terminal deoxynucleotidyl transferase-mediated dUTP-biotin nick end labeling (TUNEL) assay
Cell apoptosis was assessed using TUNEL and 4’,6-diamidino-2-phenylindole (DAPI) staining solution (Thermo Fisher Scientific, Waltham, MA, USA). The nucleus was stained by DAPI to differentiate apoptotic and non-apoptotic cells. TUNEL and DAPI double staining can distinguish apoptotic cells. The lung tissue sections were fixed in 4% paraformaldehyde (PFA, Sigma-Aldrich) for 30 min at room temperature and incubated with 0.5% Triton X-100 for 10 min. Subsequently, lung tissue sections were added with TUNEL reaction mixture and incubated for 60 min at 37 °C. Finally, the sections were observed under a fluorescence microscope (Leica Microsystems, Wetzlar, Germany). Six random visual fields were selected from each slide to count the number of TUNEL-positive cells and to estimate the percentage.
Lung W/D weight ratio analysis
The right lower lung was removed for measurement of wet weight. Then, the lung was dried in a 72 °C oven for 48 h before measurement of dry weight. The W/D weight ratio was estimated to evaluate pulmonary edema.
BALF collection and analysis
The BALF was collected as previously described [Citation24]. First, 1 mL 1 × phosphate buffer saline was intratracheally administered and withdrawn 3 times independently. The inflammatory cells (macrophages, lymphocytes, and neutrophils) in BALF were centrifuged and resuspended, followed by counting using a hemocytometer (MC-6200VET, MAXCOM, Shenzhen, China). The supernatant was conserved at −80 °C prior to enzyme-linked immunosorbent assay (ELISA).
Cell culture and treatment
The murine alveolar epithelial MLE-12 cells (ATCC, Manassas, VA, USA) were maintained in RPMI-1640 medium containing 10% fetal bovine serum at 37 °C in 5% CO2 [Citation25].
The IC50 value of LPS on MLE-12 cells was 10.11 μg/mL by preliminary experiments, and therefore, MLE-12 cell injury was induced using 10 μg/mL LPS (Supplementary Figure 1). MLE-12 cells were assigned to the following groups based on different treatments: the blank group (without any treatment), LPS group (treated with 10 μg/mL LPS for 24 h [Citation11], LPS + CAL groups (treated with LPS for 24 h after the addition of 3.75, 7.5, 15, 30, or 50 μg/mL CAL), LPS + CAL + inhi NC group or the LPS + CAL + miR inhi group (treated with 30 μg/mL CAL and LPS for 24 h after 48 h of transfection with inhibitor NC or miR-375-3p inhibitor), LPS + CAL + mimic NC group, LPS + CAL + miR mimi group, or LPS + CAL + miR mimi + oe-NC (negative control) group, LPS + CAL + miR mimi + oe-Rock2 group (treated with LPS for 24 h after 48 h of transfection with mimic NC, miR-375-3p mimic and/or oe-NC, oe-Rock2). Cell transfection was carried out following the specifications of Lipofectamine 2000 (11668-019, Invitrogen, Carlsbad, CA, USA) at a final concentration of 100 nM. All plasmids were procured from GenePharma (Shanghai, China) [Citation26].
4,5-dimethylthiazol-2-yl-2,5-diphenyltetrazolium bromide (MTT) assay
Cell viability was assessed using the MTT kit (M1020, Solarbio, Beijing, China). The optical density (OD) at 450 nm was detected using a microplate reader (Bio-Rad 680, Bio-Rad, Hercules, CA, USA) following the specifications of the kit.
Flow cytometry
MLE-12 cell apoptosis was detected using a V-fluorescein isothiocyanate (V-FITC)/propidium iodide (PI) kit (Beyotime, Shanghai, China) as per the instructions. Annexin V-FITC-positive and PI-negative cells were considered at an early apoptotic phase, and Annexin V-FITC-positive and PI-positive cells were at a late apoptotic phase. The apoptotic rate was expressed as the percentage of cells at early/late apoptotic phases.
Dual-luciferase reporter assay
As previously described [Citation26, Citation27], luciferase reporter vectors were constructed. The Rock2 3’UTR full-length sequences containing miR-375-3p wild-type or mutant binding sites were cloned to pMIR-Report vectors (RiboBio, Guangzhou, China) to construct the wild-type and mutant plasmids Rock2-WT and Rock2-MUT. The luciferase reporter vectors were introduced to MLE-12 cells together with miR-375-3p mimic or mimic NC using Lipofectamine 2000 (Invitrogen). Following 48 h of transfection, luciferase activity was measured using the Dual-luciferase Reporter Gene Assay System (Promega, Madison, WI, USA).
ELISA
BALF and the supernatant of MLE-12 cells were collected after LPS treatment. The concentrations of tumor necrosis factor-α (TNF-α, PT512), IL (interleukin)-1β (PI301), IL-4 (PI612), and IL-10 (PI522) were measured using ELISA kits (Beyotime, Shanghai, China). The results were collected using a microplate reader (Bio-Rad).
RT-qPCR
Total RNA was extracted from mouse lung tissues or MLE-12 cells using the TRIzol kit (Invitrogen) and inversely transcribed into cDNA using the PrimeScript RT-PCR kit (Takara, Tokyo, Japan) following standard protocols. The mRNA and miRNA levels were measured according to the instructions of the SYBR Premix Ex TaqTM kit (Takara) on the ABI Prism 7500 System (ABI, Foster City, CA, USA). U6 was utilized as the internal reference for miR-375-3p. The gene primers are demonstrated in . The relative levels were analyzed using the 2-ΔΔct method.
Table 1. Primer sequences.
Western blot
MLE-12 cells and lung tissues were lysed using a protein lysis buffer (R0278, Sigma-Aldrich). Proteins in the cell lysate and lung tissues were quantitatively analyzed using a bicinchoninic acid protein assay kit (Beyotime). After separation by 10% SDS-PAGE, the proteins were transferred onto PVDF membranes. The membranes were blocked for 1 h in Tris-buffered Tween-20 (TBST, 20 mM Tris, 137 mM NaCl, 0.1% Tween-20) containing 5% nonfat milk, and subsequently incubated with primary antibodies ROCK2 (1:10000, ab125025, Abcam, Cambridge, MA, USA) and β-actin (internal control; 1:2000, ab8227, Abcam) overnight at 4 °C. After TBST washes, the membranes were incubated with goat anti-rabbit HRP-labeled secondary antibody (1:2000, ab6721, Abcam) for 2 h at room temperature and ultimately incubated with BeyoECL Plus (Beyotime). Gray values of each band were quantified using ImageJ v1.61 (NIH Image, Bethesda, MD, USA).
Statistical analysis
Statistical analysis and data plotting were processed using GraphPad Prism 8.01 (GraphPad Software, San Diego, CA, USA). Measurement data are given as mean ± standard deviation. Comparisons between groups were analyzed using an independent sample t test, and comparisons among groups were processed using a one-way analysis of variance, followed by Turkey’s test. The p values were obtained through two-sided tests, and p < 0.05 indicated significant statistical differences.
Results
CAL alleviates LPS-induced ALI by upregulating miR-375-3p
ALI was induced in mice using 10 mg/kg LPS. HE staining demonstrated that control mice showed no obvious lung tissue damage and normal alveolar structure, and LPS-treated mice manifested with thickened alveolar walls, large amounts of inflammatory cell infiltration, and pulmonary capillary congestion and bleeding (, p < 0.05). TUNEL staining and W/D measurement tests unraveled increased cell apoptosis and pulmonary edema in the LPS group (, p < 0.05). The results of blood cell counter and ELISA revealed that the number of inflammatory cells (macrophages, lymphocytes, and neutrophils) and the levels of inflammatory cytokines (TNF-α, IL-1β, IL-4, and IL-10) in BALF in the LPS group were significantly elevated compared to the control group (, p < 0.05). These results indicated the successful establishment of LPS-induced ALI mouse models. Then, ALI mice were treated with CAL at different doses. CAL treatment significantly mitigated lung injury, pulmonary edema, and cell apoptosis in mice, decreased the number of inflammatory cells and levels of TNF-α and IL-1β, and augmented levels of IL-4 and IL-10 (, p < 0.05). In ALI mice treated with low and medium doses of CAL, the higher the CAL dose, the stronger the mitigating action, while there was no distinct difference between the LPS + 100 mg/kg CAL and LPS + 50 mg/kg CAL groups. RT-qPCR revealed that miR-375-3p expression was decreased in the LPS group relative to that in the control group, and CAL elevated miR-375-3p expression (, p < 0.05). The above results illustrated that CAL ameliorates LPS-evoked ALI by upregulating miR-375-3p.
Figure 1. CAL alleviates LPS-induced ALI by upregulating miR-375-3p. (A) lung tissues stained by HE and histopathological scores estimated according to the severity of lung injury; (B) cell apoptosis assessed by TUNEL staining; (C) pulmonary edema assessed by W/D ratio; (D) number of inflammatory cells (macrophages, lymphocytes, and neutrophils) in BALF counted using a blood cell counter; (E) levels of TNF-α, IL-1β, IL-4 and IL-10 in BALF determined by ELISA; (F) miR-375-3p expression measured by RT-qPCR. N = 6. Data are shown as mean ± standard deviation. Data among groups were compared using a one-way analysis of variance, followed by Turkey’s test. nsp > 0.05, *p < 0.05, **p < 0.01, ***p < 0.001.
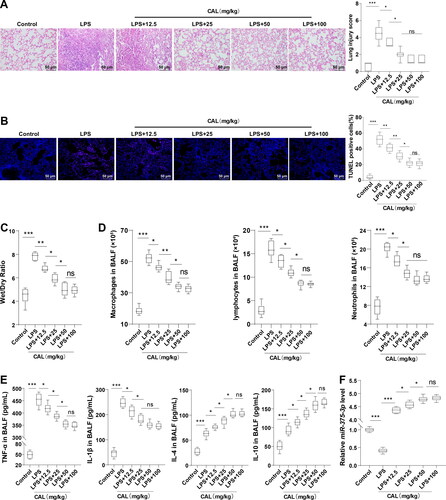
CAL extenuates LPS-evoked MLE-12 cell injury by upregulating miR-375-3p
Subsequently, murine alveolar epithelial MLE-12 cells were cultured in vitro and used to establish the cell models of LPS-induced ALI. MTT, flow cytometry, and ELISA exhibited that LPS reduced MLE-12 cell viability and increased apoptosis and elevated levels of TNF-α, IL-1β, IL-4, and IL-10 (, all p < 0.01), which indicated the successful establishment of LPS-induced MLE-12 cell injury model. MTT demonstrated that CAL at medium and low dose levels enhanced cell viability, 50 μg/mL CAL reduced cell viability relative to 30 μg/mL CAL (). Thus, 30 μg/mL CAL was used in subsequent experimentation. Afterward, CAL treatment strengthened the viability and decreased apoptosis of LPS-treated MLE-12 cells, decreased levels of TNF-α and IL-1β, and increased levels of IL-4 and IL-10 (, all p < 0.01). Thus, it could be inferred that CAL alleviated LPS-induced MLE-12 cell injury. In addition, RT-qPCR manifested a decrease in miR-375-3p expression in the LPS group relative to the blank group and an increase in miR-375-3p expression in LPS + CAL group compared to the LPS group (, all p < 0.001). These results unraveled that CAL alleviates LPS-induced MLE-12 cell injury by upregulating miR-375-3p.
Figure 2. CAL extenuates LPS-evoked MLE-12 cell injury by upregulating miR-375-3p. (A) the best dose level of CAL determined by MTT; (B) cell viability assessed by MTT; (C) cell apoptosis assessed by flow cytometry; (D) levels of TNF-α, IL-1β, IL-4, and IL-10 in MLE-12 cells determined by ELISA; (E) miR-375-3p expression measured by RT-qPCR. Data are shown as mean ± standard deviation. Cell experiments were repeated 3 times. Data among groups were compared using a one-way analysis of variance, followed by Turkey’s test. **p < 0.01, ***p < 0.001.
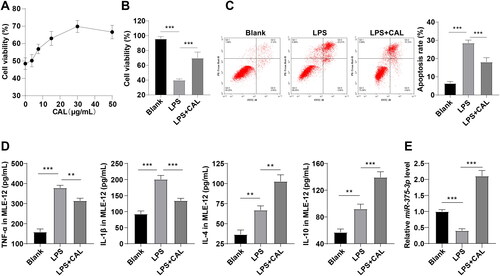
Inhibition of miR-375-3p partially negates CAL-mediated inhibition on LPS-induced MLE-12 cell injury
To ascertain the effects of miR-375-3p on LPS-triggered MLE-12 cell injury, LPS-treated cells were transfected with miR-375-3p inhibitor after CAL treatment, with cells transfected with inhi NC as controls. RT-qPCR validated the miR-375-3p transfection efficiency (, p < 0.001). MTT, flow cytometry, and ELISA illustrated no significant difference in cell viability, apoptosis, and levels of inflammatory cytokines between the LPS + CAL group and LPS + CAL + inhi NC group, while miR-375-3p inhibitor partially nullified CAL-exerted inhibitory effects on LPS-triggered MLE-12 cell injury relative to inhibitor NC (, all p < 0.01). Shortly, CAL suppresses LPS-induced MLE-12 cell injury by upregulating miR-375-3p.
Figure 3. Inhibition of miR-375-3p partially negates CAL-mediated inhibition on LPS-induced MLE-12 cell injury. (A) miR-375-3p transfection efficiency confirmed by RT-qPCR; (B) cell viability assessed by MTT; (C) cell apoptosis assessed by flow cytometry; (D) levels of TNF-α, IL-1β, IL-4, and IL-10 in MLE-12 cells determined by ELISA. Data are shown as mean ± standard deviation. Cell experiments were repeated 3 times. Data among groups were compared using a one-way analysis of variance, followed by Turkey’s test. **p < 0.01, ***p < 0.001.
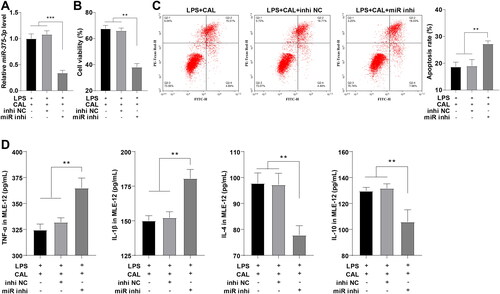
miR-375-3p ameliorates LPS-induced ALI by targeting ROCK2
To further investigate the potential mechanism of miR-375-3p in LPS-provoked MLE-12 cell injury, LPS-treated MLE-12 cells were transfected with miR-375-3p mimic, with cells transfected with mimic NC as controls. RT-qPCR confirmed the downregulation of miR-375-3p in the LPS group and upregulation of miR-375-3p after transfection with miR-375-3p mimic (, p < 0.01). There is emerging evidence that ROCK2 and ALI are associated, and silencing ROCK2 can mitigate LPS-induced ALI [Citation20, Citation21]. Western blot presented that ROCK2 expression was augmented in LPS-treated MLE-12 cells (, p < 0.001), and LPS upregulated ROCK2 expression in lung tissues of ALI mice, while CAL at low and medium dose levels partially abrogated the LPS-induced change (, p < 0.05). We thereby speculated that miR-375-3p might repress LPS-induced ALI by targeting ROCK2. The potential binding site of miR-375-3p and ROCK2 was predicted on the Starbase database. Dual-luciferase reporter assay elicited that luciferase activity declined after co-transfection with Rock2-WT and miR mimi, indicative of the binding between miR-375-3p and ROCK2 (). Furthermore, ROCK2 expression was diminished after overexpressing miR-375-3p (, p < 0.001). These results validated that miR-375-3p targets ROCK2. Moreover, LPS-induced MLE-12 cells exhibited decreased viability, increased apoptosis, elevated levels of TNF-α and IL-1β, and diminished levels of IL-4 and IL-10 after transfection with miR-375-3p mimic and oe-Rock2 (, p < 0.001). Conjointly, miR-375-3p targets ROCK2, thus extenuating LPS-induced ALI.
Figure 4. miR-375-3p ameliorates LPS-induced ALI by targeting ROCK2. (A) miR-375-3p transfection efficiency confirmed by RT-qPCR; (B) ROCK2 expression in MLE-12 cells determined by Western blot; (C) ROCK2 expression in lung tissues of mice determined by Western blot; (D) target relationship between miR-375-3p and ROCK2 confirmed by dual-luciferase reporter assay; (E) cell viability assessed by MTT; (F) cell apoptosis assessed by flow cytometry; (G) levels of TNF-α, IL-1β, IL-4, and IL-10 in MLE-12 cells determined by ELISA. Data are shown as mean ± standard deviation. Cell experiments were repeated 3 times. Data in figures (A-C) and (E-G) were analyzed using a one-way analysis of variance, followed by Turkey’s test. Data in Figure (D) were analyzed using t test. *p < 0.05, **p < 0.01, ***p < 0.001.
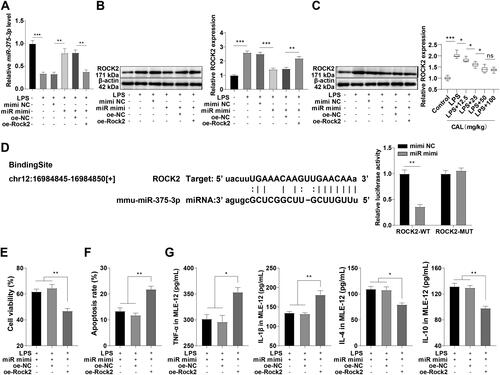
Discussion
ALI is a critical disease that predisposes to ARDS and is characterized by rapid onset and high death rates [Citation28]. Many Chinese medicines such as heat-clearing ones have been extensively used to treat ALI by suppressing inflammatory actions [Citation29]. CAL, an isoflavonoid, is a major active component of Astragalus membranaceus that has been widely used in the treatment of diabetes-induced renal inflammation [Citation30], myocardial infarction [Citation31], and lung cancer [Citation32]. miRNAs are tightly linked to ALI and miR-375-3p can relieve myocardial injury in septic mice and participate in the development of lung disease [Citation17, Citation18]. What’s more, ROCK2 knockdown can attenuate LPS-induced ALI [Citation20, Citation21]. Therefore, we suspected that CAL ameliorates LPS-induced ALI in mice by modulating the miR-375-3p/ROCK2 axis. The results of the present study evinced that CAL exerts protective effects on mice against LPS-stimulated ALI by upregulating miR-375-3p to target ROCK2.
The formation of alveolar edema, changes in the alveolar surface, and increases in inflammatory markers are specific signs of ALI [Citation33]. The apoptosis of alveolar epithelial cells is a pivotal factor in the pathological process of ALI [Citation34]. CAL treatment relieved lung damage and decreased cell apoptosis and pulmonary edema in LPS-treated mice. Moreover, the levels of TNF-α and IL-1β were decreased and levels of IL-4 and IL-10 were augmented in BALF of LPS-treated mice after CAL treatment. Neutrophils, macrophages, and lymphocytes dominate the inflammatory response in ALI [Citation35]. CAL reduced the number of macrophages, neutrophils, and lymphocytes in BALF of LPS-treated mice. A dose-dependent pattern was observed in mice treated with CAL at low and medium dose levels. In addition, the identical tendency in cell apoptosis and levels of inflammatory cytokines was observed in the in vitro assay of LPS-induced MLE-12 cells. Plus, CAL treatment greatly enhanced the viability of LPS-treated MLE-12 cells. These results coincided with the finding of Yu et al. [Citation12] that CAL extenuates LPS-induced ALI.
miR-375-3p presents a decreased expression level in hypoxia-induced pulmonary microvascular endothelial cells [Citation18]. CAL can elevate miR-375 expression in cerebral ischemia/reperfusion injury [Citation36]. Our results showed a similar downward pattern in miR-375-3p expression in ALI mice and an upward trend after CAL treatment. Moreover, the inhibition of miR-375-3p reduced MLE-12 cell viability and levels of anti-inflammatory factors and augmented cell apoptosis and levels of pro-inflammatory factors. miR-375-3p can relieve myocardial injury in septic mice and participate in the development of lung disease [Citation17, Citation18]. Thus, it can be deduced that CAL upregulates miR-375-3p and the inhibition of miR-375-3p partially annuls CAL-mediated inhibitory functions on MLE-12 cell injury.
In terms of functional mechanisms, the miR-424/ROCK2 axis is implicated in ALI [Citation37]. ROCK2 also acts as a target gene of miR-330-5p in LPS-induced injury to MLE-12 cells [Citation20]. Our results disclosed that ROCK2 was highly expressed in LPS-induced MLE-12 cells and downregulated after miR-375-3p overexpression, and dual-luciferase assay further confirmed the target relationship between miR-375-3p and ROCK2. The knockdown of ROCK2 in LPS-treated RLE-6TN cells facilitates cell proliferation, hinders cell apoptosis, and inhibits inflammation [Citation38]. Likewise, our results elicited that overexpression of ROCK2 in LPS-treated MLE-12 cells decreased cell viability, increased apoptosis, raised the levels of TNF-α and IL-1β, and diminished the levels of IL-4 and IL-10. Silencing of ROCK2 alleviates lung injury induced by a ventilator in mice through the inhibition of NLRP3 signaling in alveolar macrophages [Citation39]. ROCK2 knockdown in LPS-induced HK-2 cells reversed cell injury and inhibited the levels of pro-inflammatory cytokines and apoptosis in acute kidney injury [Citation21]. Similarly, our results demonstrated that miR-375-3p ameliorates LPS-evoked MLE-12 cell injury by inhibiting ROCK2 expression.
In conclusion, our study highlighted that CAL can upregulate miR-375-3p to suppress ROCK2 expression, thus protecting against LPS-induced ALI in mice. However, there are some limitations. First, LPS was used to establish both the in vivo mouse models and in vitro cell models of ALI and further verification in cecal ligation and puncture (CLP)-established models is lacking. Second, this study lacks the investigation of potential regulatory pathways other than the miR-375-3p/ROCK2 axis involved in the mechanism of CAL in LPS-triggered ALI mice. Third, by using male C57BL/6N mice only to establish the LPS-induced ALI models, this study didn’t probe into the effect of gender on the mechanism that CAL extenuates LPS-induced ALI in mice through the miR-375-3p/ROCK2 axis. The establishment of CLP mouse models is necessary for future studies to further validate the protective effect of CAL on ALI mice through miR-375-3p. Furthermore, whether gender affects the functional mechanism that CAL extenuates LPS-induced ALI in mice through the miR-375-3p/ROCK2 axis shall be investigated in depth.
Authors’ contributions
JY is the guarantor of integrity of the entire study and contributed to the study design, study concepts, experimental studies, manuscript review; MYC contributed to the literature research, data acquisition, manuscript editing; FY contributed to the definition of intellectual content, data analysis, statistical analysis; All authors read and approved the final manuscript.
Ethical statement
Animal-associated experimental procedures were approved by the Animal Ethics Committee of People’s Hospital Affiliated with the Fujian University of Chinese Medicine in compliance with the ARRIVE guidelines and relevant regulations and guidelines. All animals were given humane care.
Supplemental Material
Download TIFF Image (228.6 KB)Data availability statement
The data that support the findings of this study are available from the corresponding author upon reasonable request.
Disclosure statement
The authors declared no potential conflicts of interest with respect to the research, authorship, and publication of this article.
Additional information
Funding
References
- Liu X, Gao C, Wang Y, Niu L, Jiang S, Pan S. BMSC-derived exosomes ameliorate LPS-induced acute lung injury by miR-384-5p-controlled alveolar macrophage autophagy. Oxid Med Cell Longev. 2021;2021:1. doi:10.1155/2021/9973457.
- Kumar V. Pulmonary innate immune response determines the outcome of inflammation during pneumonia and sepsis-associated acute lung injury. Front Immunol. 2020;11:1722. doi:10.3389/fimmu.2020.01722.
- Butt Y, Kurdowska A, Allen TC. Acute lung injury: a clinical and molecular review. Arch Pathol Lab Med. 2016;140(4):345–9. doi:10.5858/arpa.2015-0519-RA.
- Mowery NT, Terzian WTH, Nelson AC. Acute lung injury. Curr Probl Surg. 2020;57(5):100777. doi:10.1016/j.cpsurg.2020.100777.
- Mokra D. Acute lung injury – from pathophysiology to treatment. Physiol Res. 2021;69:S353–S366. doi:10.33549/physiolres.934602.
- da Silva Sergio LP, Mencalha AL, de Souza da Fonseca A, de Paoli F. DNA repair and genomic stability in lungs affected by acute injury. Biomed Pharmacother. 2019;119:109412. doi:10.1016/j.biopha.2019.109412.
- He YQ, Zhou CC, Yu LY, et al. Natural product derived phytochemicals in managing acute lung injury by multiple mechanisms. Pharmacol Res. 2021;163:105224. doi:10.1016/j.phrs.2020.105224.
- Ding Z, Zhong R, Xia T, et al. Advances in research into the mechanisms of Chinese Materia Medica against acute lung injury. Biomed Pharmacother. 2020;122:109706. doi:10.1016/j.biopha.2019.109706.
- Deng M, Chen H, Long J, Song J, Xie L, Li X. Calycosin: a review of its pharmacological effects and application prospects. Expert Rev anti Infect Ther. 2021;19(7):911–925. doi:10.1080/14787210.2021.1863145.
- Wang C, Luo J, Bai X, et al. Calycosin alleviates injury in airway epithelial cells caused by PM 2.5 exposure via activation of AMPK signalling. Evid Based Complement Alternat Med. 2021;2021:8885716. doi:10.1155/2021/8885716.
- Chen G, Hou Y, Li X, Pan R, Zhao D. Sepsis-induced acute lung injury in young rats is relieved by calycosin through inactivating the HMGB1/MyD88/NF-kappaB pathway and NLRP3 inflammasome. Int Immunopharmacol. 2021;96:107623. doi:10.1016/j.intimp.2021.107623.
- Xia Y, Cao Y, Sun Y, et al. Calycosin alleviates Sepsis-induced acute lung injury via the inhibition of mitochondrial ROS-mediated inflammasome activation. Front Pharmacol. 2021;12:690549. doi:10.3389/fphar.2021.690549.
- Lu TX, Rothenberg ME. MicroRNA. J Allergy Clin Immunol. 2018;141(4):1202–1207. doi:10.1016/j.jaci.2017.08.034.
- Jiang ZF, Zhang L, Shen J. MicroRNA: potential biomarker and target of therapy in acute lung injury. Hum Exp Toxicol. 2020;39(11):1429–1442. doi:10.1177/0960327120926254.
- Ju M, Liu B, He H, et al. MicroRNA-27a alleviates LPS-induced acute lung injury in mice via inhibiting in fl ammation and apoptosis through modulating TLR4/MyD88/NF-kappaB pathway. Cell Cycle. 2018;17(16):2001–2018. doi:10.1080/15384101.2018.1509635.
- Xie W, Lu Q, Wang K, et al. miR-34b-5p inhibition attenuates lung inflammation and apoptosis in an LPS-induced acute lung injury mouse model by targeting progranulin. J Cell Physiol. 2018;233(9):6615–6631. doi:10.1002/jcp.26274.
- Hong X, Wang J, Li S, Zhao Z, Feng Z. MicroRNA-375-3p in endothelial progenitor cells-derived extracellular vesicles relieves myocardial injury in septic rats via BRD4-mediated PI3K/AKT signaling pathway. Int Immunopharmacol. 2021;96:107740. doi:10.1016/j.intimp.2021.107740.
- An Y, Liu Z, Ding H, et al. MiR-375-3p regulates rat pulmonary microvascular endothelial cell activity by targeting Notch1 during hypoxia. J Int Med Res. 2020;48(7):300060520926851. doi:10.1177/0300060520926851.
- Wang Y, Wang X, Liu W, Zhang L. Role of the Rho/ROCK signaling pathway in the protective effects of fasudil against acute lung injury in septic rats. Mol Med Rep. 2018;18(5):4486–4498. doi:10.3892/mmr.2018.9446.
- Ren Y, Li L, Wang M, et al. Knockdown of circRNA paralemmin 2 ameliorates lipopolysaccharide-induced murine lung epithelial cell injury by sponging miR-330-5p to reduce ROCK2 expression. Immunol Invest. 2022;51(6):1707–1724. doi:10.1080/08820139.2022.2027961.
- Qian X, Yang L. ROCK2 knockdown alleviates LPS-induced inflammatory injury and apoptosis of renal tubular epithelial cells via the NF-kappaB/NLRP3 signaling pathway. Exp Ther Med. 2022;24(3):603. doi:10.3892/etm.2022.11540.
- Vettorazzi S, Bode C, Dejager L, et al. Glucocorticoids limit acute lung inflammation in concert with inflammatory stimuli by induction of SphK1. Nat Commun. 2015;6:7796. doi:10.1038/ncomms8796.
- Sweeney KA, Dao DN, Goldberg MF, et al. A recombinant Mycobacterium smegmatis induces potent bactericidal immunity against Mycobacterium tuberculosis. Nat Med. 2011;17(10):1261–1268. doi:10.1038/nm.2420.
- Chen J, Yang X, Zhang W, et al. Therapeutic effects of resveratrol in a mouse model of LPS and cigarette smoke-induced COPD. Inflammation. 2016;39(6):1949–1959. doi:10.1007/s10753-016-0430-3.
- Yang P, Xiong W, Chen X, Liu J, Ye Z. Overexpression of miR-129-5p mitigates sepsis-induced acute lung injury by targeting high mobility group Box 1. J Surg Res. 2020;256:23–30. doi:10.1016/j.jss.2020.05.101.
- Sheng B, Zhao L, Zang X, Zhen J, Chen W. miR-375 ameliorates sepsis by downregulating miR-21 level via inhibiting JAK2-STAT3 signaling. Biomed Pharmacother. 2017;86:254–261. doi:10.1016/j.biopha.2016.11.147.
- Wu Q, Zhao Y, Sun Y, Yan X, Wang P. miR-375 inhibits IFN-gamma-induced programmed death 1 ligand 1 surface expression in head and neck squamous cell carcinoma cells by blocking JAK2/STAT1 signaling. Oncol Rep. 2018;39(3):1461–1468. doi:10.3892/or.2018.6177.
- Rubenfeld GD, Caldwell E, Peabody E, et al. Incidence and outcomes of acute lung injury. N Engl J Med. 2005;353(16):1685–1693. doi:10.1056/NEJMoa050333.
- Lu ZB, Ou JY, Cao HH, Liu JS, Yu LZ. Heat-clearing Chinese medicines in lipopolysaccharide-induced inflammation. Chin J Integr Med. 2020;26(7):552–559. doi:10.1007/s11655-020-3256-7.
- Zhang YY, Tan RZ, Zhang XQ, Yu Y, Yu C. Calycosin ameliorates diabetes-induced renal inflammation via the NF-kappaB pathway in vitro and in vivo. Med Sci Monit. 2019;25:1671–1678. doi:10.12659/MSM.915242.
- Cheng Y, Zhao J, Tse HF, Le XC, Rong J. Plant natural products calycosin and gallic acid synergistically attenuate neutrophil infiltration and subsequent injury in isoproterenol-induced myocardial infarction: a possible role for leukotriene B4 12-hydroxydehydrogenase? Oxid Med Cell Longev. 2015;2015:434052. doi:10.1155/2015/434052.
- Zhou L, Chen W, Yang H, Liu J, Meng H. Calycosin inhibits the malignant behaviors of lung adenocarcinoma cells by regulating the circ_0001946/miR-21/GPD1L/HIF-1alpha signaling axis. Dis Markers. 2022;2022:3969389. doi:10.1155/2022/3969389.
- Knudsen L, Ochs M. The micromechanics of lung alveoli: structure and function of surfactant and tissue components. Histochem Cell Biol. 2018;150(6):661–676. doi:10.1007/s00418-018-1747-9.
- Liu Y, Xiang D, Zhang H, Yao H, Wang Y. Hypoxia-inducible factor-1: a potential target to treat acute lung injury. Oxid Med Cell Longev. 2020;2020:8871476. doi:10.1155/2020/8871476.
- Wang L, Wang X, Tong L, et al. Recovery from acute lung injury can be regulated via modulation of regulatory T cells and Th17 cells. Scand J Immunol. 2018;88(5):e12715. doi:10.1111/sji.12715.
- Wang Y, Dong X, Li Z, Wang W, Tian J, Chen J. Downregulated RASD1 and upregulated miR-375 are involved in protective effects of calycosin on cerebral ischemia/reperfusion rats. J Neurol Sci. 2014;339(1–2):144–148. doi:10.1016/j.jns.2014.02.002.
- Chen H, Hu X, Li R, et al. LncRNA THRIL aggravates sepsis-induced acute lung injury by regulating miR-424/ROCK2 axis. Mol Immunol. 2020;126:111–119. doi:10.1016/j.molimm.2020.07.021.
- Liu J, Wu YH, Zhang ZL, Li P. Tanshinone IIA improves sepsis-induced acute lung injury through the ROCK2/NF-kappaB axis. Toxicol Appl Pharmacol. 2022;446:116021. doi:10.1016/j.taap.2022.116021.
- Zhang S, Zhu L, Dai H, Pan L. Silencing ROCK1 ameliorates ventilator-induced lung injury in mice by inhibiting macrophages’ NLRP3 signaling. Int Immunopharmacol. 2021;101(Pt A):108208. doi:10.1016/j.intimp.2021.108208.