Abstract
The Loess Plateau of China suffers serious soil erosion primarily resulting from irrational land uses. Soil water plays a critical role influencing vegetation-restoration processes, but varies with temporal and spatial characteristics, of concern to many researchers. However, few studies consider the influence of deep soil water and varied weather patterns in a semi-arid region. Four vegetation types chosen for this study include artificial caragana shrubland, artificial alfalfa grassland, naturally restored vegetation land (Artemisia capillaries, Agropyron chistatum, Heteropapus altaicus Novop, Stipa bungeana, Stipa breviflora griseb and Lespedeza davurica), and bare land. Soil-water content at the top 400 cm depth was monitored continuously from 2004 to 2007 using a neutron probe. Water and wind soil erosion were investigated gravimetrically. Results showed that soil water varied greatly during May through October with different land-use types and annual precipitations. Soil water reached a lower level in both artificial vegetation lands in dry years but recharged with great variation in normal years. Soil water in two other lands tended to have similar changes with less variation as compared with the artificial vegetation lands. Soil water was intensively consumed with depth and continuously decreased in the deeper layers under the two artificial vegetations. The consumption in the artificial caragana shrubland was more intensive, while that in the artificial alfalfa grassland took place at greater depths. The naturally restored vegetation land had less soil-water consumption (close to the level in the bare land) as compared with the artificial vegetation lands. Soil-water content and its variability were increased along the slope with less variation over time. In general, both artificial vegetations effectively reduced the runoff and water-wind soil erosion in the third year after planting, while the naturally restored vegetation consistently showed weak conservation effects in the initial stage.
Introduction
The Loess Plateau of China is widely known for its severe soil-erosion problem, especially in the water-wind erosion crisscross region, due to alternative water erosion in rainy seasons and wind erosion in dry seasons. One of the most important reasons is the unreasonable land use (Fu & Gulinck, Citation1994). The land use, to a large extent driven by human activities, is the most active factor that influences soil water and is closely related to food production, human lives, and ecological environments, determines soil-water level and temporal-spatial variation by affecting runoff, infiltration, water redistribution, and evapotranspiration (Fu et al., 2000, 2003; Qiu et al., Citation2001; Wahl et al., Citation2003; Zhang & Schilling, Citation2006; Cubera & Moreno, Citation2007). It also controls soil-erosion intensity, frequency through intercepting rainfall, and improves soil physical and chemical properties (Bochet et al., Citation1998, Citation2006; Boix-Fayos et al., Citation1998; Wei et al., Citation2007).
Under extremely dry conditions, soil water becomes the primary limiting factor influencing ecological environments for plant species and vegetation-restoration processes in both arid and semi-arid regions (Nash et al., Citation1991; Hu & Shao, Citation2002). Soil water helps one understand soil-water dynamics, hydrological models, and linkages among regional hydrology, ecology, and physiography (Grayson et al., Citation1992; Petrone et al., Citation2004). Soil water is influenced by many factors, such as soil properties (Reynolds, Citation1970), mean initial soil-water content (Bell et al., Citation1980), slope position, relative elevation (Crave & Gascuel-odux, Citation1997), aspects (Western et al., Citation1999), topography (Burt & Butcher, Citation1985), and vegetation (Fu et al., Citation2000; Wang et al., Citation2003a). To some extent, soil water is primarily affected by precipitation in a semi-arid region with the exception of the land-use types. The uneven distribution of inter-annual and -seasonal precipitation greatly impacts soil-water level, variation, and interaction depth, causing soil-water content to exhibit tremendous temporal and spatial variability in semi-arid regions (Grayson et al., Citation1997; Famiglietti et al., Citation1998; Gómez-Plaza et al., Citation2000). The intensity of temporal and spatial variability of soil water on the surface controls matter interactions and energy fluxes, and this has been studied for many years (Schume et al., Citation2003; Hupet & Vanclooster, Citation2005; Ran et al., Citation2005; Lin et al., Citation2006; Brocca et al., Citation2007). However, various researches were conducted merely to measure soil water within upper layers for a few days to a few months (Martínez-Fernández & Ceballos, Citation2003, Citation2005; Thierdefelder et al., Citation2003; Grant et al., Citation2004; Petrone et al., Citation2004; Pachepsky et al., Citation2005; Bosch et al., Citation2006; Cosh et al., Citation2006; Starks et al., Citation2006). In other words, these studies emphasized the effects of shallow soil layers, short durations, or existing status rather than entire variations of the process. Cosh et al. attempted to extend their study period and investigated overall changes of soil water in Chickasha, Oklahoma, but their results were beyond their expectations because of an unusually dry summer, which yields a short-term experimental period. They concluded that a longer period was needed to avoid influences of seasons and weather patterns (Cosh et al., Citation2004, 2006). On the other hand, Western and Grayson found that soil water further affected hydrological processes on surface soil as well as in the subsoil (Western & Grayson, Citation1998).
On the Loess Plateau, the greater thickness of the loess and the lower water table make precipitation the sole source of soil water. Hence, pedogenic reservoirs become a crucial source of soil water in the region and play an important role in regionalized ecological environment (Zhu, Citation2000, Citation2006). Meanwhile, various investigations verified that soil water in the deeper layers also significantly influenced the semi-arid areas of the Loess Plateau (Wang et al., Citation2003b; Li et al., Citation2007, Citation2008). Therefore, soil water in the deeper layers should be considered as well as in the shallow layers.
In this study, four plots were established to represent the different land-use types: artificial caragana (Caragana korshinskii) shrubland, artificial alfalfa (Medicago sativa) grassland, naturally restored vegetation land, and bare land. Those plots of 61 m in length and 5 m in width originated from a unique abandoned farmland and were constructed to continuously measure soil water at a depth of 0–400 cm during a four-year period. Precipitation levels and water-wind erosion of the soil were also investigated during the experimental period. The aims of this study were: 1) to investigate temporal and spatial variability of soil water under four types of land use; 2) to evaluate effects of soil and water conservation with different land-use types.
Materials and methods
Study area and experimental units
The study was conducted in Liudaogou catchment (38°46′–38°51′N, 110°21′–110°23′E, 1081–1274 m elevation), Shenmu County, on the Northern Loess Plateau of China. The catchment was located in the transitional region from the Loess hills to the Maowusu desert. This crisscross region on the Loess Plateau is a water-wind erosion hotspot. The region has a semi-arid continental climate with average annual temperature of 8.4 °C and total annual precipitation level of 437.4 mm averaged across 1957–1989. The annual precipitation level varies greatly among seasons and accounts for 70% of the rainfall during June through September. The original vegetation was destroyed by human activities and replaced mainly by drought shrub-clustered grass and artificial plants in the past 20–30 years.
The basic experimental unit is the plot, constructed in July through October, 2003. The experiment was laid on uniform cultivation farmland that had been abandoned for 2 years before this study started. Each plot was 61 m long and 5 m wide, with a slope gradient of 13–14° in the northwest direction. The protective belt totalling 1 m in width was established between neighboring plots. Runoffs in the plots were collected by the runoff pools at the lower end of the slope. Soil in the plots is Aeolian loess (45.4–50.9% of sand, 30.1–44.5% of silt, 11.2–14.3% of clay, according to the USDA soil classification system), highly erodible with erosion modulus of 15 000 t·km−2·a−1 (Tang et al., Citation1993). Soil bulk density is about 1.35–1.42 g·cm−3. Soil fertility is very low, with 4.36 g·kg−1 of organic matter content, 0.38 g·kg−1of total nitrogen, 0.53 g·kg−1of total phosphorus, and 6.40 g·kg−1 of available potassium.
Experimental designs
Four different land-use types were selected: artificial caragana shrubland (T1), artificial alfalfa grassland (T2), naturally restored vegetation land (T3), and bare land (T4) (), with detailed information as follows: 1) T1: 1-year caragana seedlings were sown in holes at a density space of 70 cm × 70 cm, then left alone to grow naturally; 2) T2: alfalfa seeds were planted in furrows at a density of 50 cm per row, then left alone to grow naturally, except the above-ground parts were cut in the beginning of July and October every year; 3) T3: recovered naturally without any human intervention after the construction. The dominating populations were Artemisia capillaries, Agropyron chistatum, Heteropapus altaicus (willd.) Novop in the first two years of restoration, and then gradually replaced by Stipa bungeana, Stipa breviflora griseb, and Lespedeza davurica; 4) T4: plowed once in May of 2004, without human intervention but regular weeding thereafter.
Figure 1. Locations of the field study and experimental layout of the plots (T1-artificial caragana shrubland, T2-artificial alfalfa grassland, T3-natural restoration land, T4-bare land).
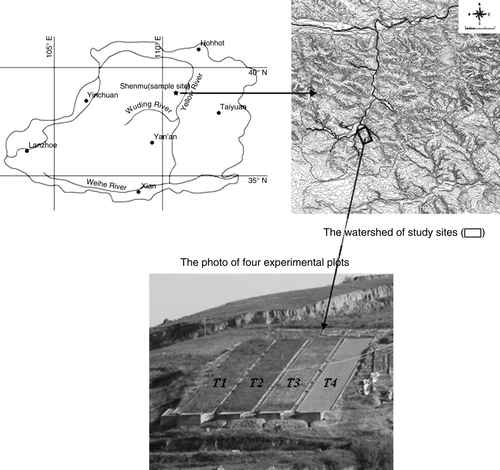
Soil water at 0–400 cm layers of soil profile was monitored by neutron tubes. In each plot, 11 neutron tubes were installed with the equal interval of 5 m along the center line at top-bottom aspect. Precipitation was recorded by an auto data-logged rain gauge in the nearby meteorology station. Rain levels were also measured manually during the rainy seasons by an additional rain gauge to calibrate the auto hyetometer in a rainstorm. Runoffs were gravimetrically measured after rainfall each time. All of the studies began from July of 2004 until the end of 2007. Wind erosion were investigated from December 1, 2006 through May 20, 2007 by sand collectors and gravimetrically measured for size distribution. All collectors were placed on the top of the windward slope with 20cm2 collection area at three different heights: 10–30 cm, 40–60 cm, and 70–90 cm.
One-way ANOVA was performed to test the influence of land-use types on soil-water content with the significance level set at 0.05. Coefficient of variation (CV%) were used to evaluate the magnitude of soil-water variability. Heterogeneity was considered weak if CV% ≤ 10%, moderate if 10% < CV% ≤ 100% and strong if CV% >100%. All the analyses were conducted with the SPSS 13.0 software, Ver. 13.0 software for Windows (SPSS Inc., Chicago, Illinois, USA).
Results and discussion
Precipitation characteristics
The lack of surface-water resources, thickness of the loess, and low groundwater tables all contribute to the fact that precipitation is the sole source of soil water in the study area. Precipitation varied inter-annually and -seasonally. The total annual precipitation levels of 410.1, 279.8, 343.4, and 428.4 mm in 2004 through 2007 accounted for 94, 64, 79, and 98%, respectively, of that for an average year (). Hence, both the years 2004 and 2007 were characterized as average while the years 2005 and 2006 were characterized as drought (The State Bureau of Technical Supervision, Citation2006). Variability of seasonal precipitation was low during the previous November and the next March, whereas it was high during July and August. The other months were moderate. This was in accord with the climatic characteristics of the semi-arid region.
Table I. Monthly precipitation distribution and averaged annual precipitation (mm) during 2004–2007.
Temporal variation of water storage in four types of land use
shows the temporal variation of soil-water storage within the 400 cm depth in four types of land use during 2004–2007, as well as daily precipitation and standard deviation of soil water over time. The inter-annual and -seasonal variations of soil-water storage are noteworthy. Soil-water storage in the four types of land use was relatively stable from the previous November to the next April. Similar trends were observed for the four types of land use. This could be attributed to the scanty precipitation and weak evapotranspiration during the periods (Famiglietti et al., Citation1998).
Figure 2. Temporal variations of soil-water storage within 400 cm depth in four types of land use with daily precipitation (mm). Also shown are related standard-deviation values.
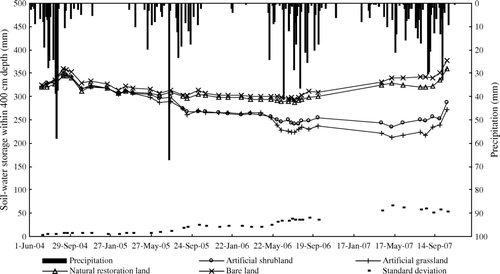
Variations of water storage between May and October were more obvious in each year due to different precipitation and vegetation types. In the drought years of 2005 and 2006, water storage in T3 and T4 plots was basically kept in balance under drought. However, water levels in T1 and T2 plots were significantly decreased during drought periods until sufficient water was supplied to meet a higher need for excessive water consumption by the artificial vegetations. Hence, the variation of water storage among the four types of land use was increased during the drought period. In the normal years of 2004 and 2007, the water storage was recharged in four types of land use due to frequent and strong rainfall events. Variation among the land-use types tended to decrease following a sufficient water supply (2007) after a long-term drought in 2005 and 2006. The reduced runoff and increased infiltration attributed to vegetation covers seemed to be responsible for the deduction of water-storage variation (Qiu et al., Citation2001). The sufficient rainfall in 2007 might have eliminated the differences in evapotranspiration or soil properties among the land-use types.
Further analyses of soil-water storage during the four years (2004–2007) indicated that the highest water storage almost always occurred around October after a rainy season (). This occurrence could be attributed to the sufficient water supply during this period. In contrast, the lowest water storage always occurred around June before a rainy season. This could be attributed to less rainfall and higher evapotranspiration during this period. The result was in accord with those previously reported by other researchers (Fu et al., Citation2003; Kimura et al., Citation2006; Chen et al., Citation2007). In our study, soil-water storage within the 400 cm depth in T1 and T2 plots was about 40–50 mm less (consumed) by the end of the study (October 20, 2007), as compared with the initial phase of this study (July 8, 2004). However, in T3 and T4 plots, soil-water storage was recharged about 40–50 mm though the precipitation conditions were the same.
The net budgets of soil-water storage during the four years of study were calculated for different types of land use, in terms of the water balance sheet (). Variation of soil-water storage in each year tended to be linearly related to the annual infiltration. The relationships can be described by the equation shown in , where a and b are two parameters guiding rainfall infiltration conversion rate and water-consumption rate, respectively. As observed from different plots in this field experiment, both a and b showed an order of T2 > T1 > T3 > T4. Lands with artificial vegetation showed higher water consumption over the no-rainfall period and higher water conversion during the rainfall events. About 316.5 mm of net infiltration could keep soil water in a good balance for the bare land, and about 345.9, 355.7, and 321.5 mm for the artificial caragana shrubland, artificial alfalfa grassland, and naturally restored vegetation land, respectively. These values can be roughly used to estimate the budget of soil-water storage with different rainfall patterns or land-use types in the study region.
Table II. Relationships between variation of soil-water storage and infiltration capacity.
Multiple comparisons indicated that there were significant differences in soil-water storage for T1, T2 plots verses T3 and T4 plots after drought periods in October 2005 (). The soil-water storage in T1 and T2 plots was less than in the T3 and T4 plots. The two artificial vegetations tended to have higher water consumption than did the naturally restored vegetation under drought conditions. Differences between T1 and T2 plots became apparent in October 2006, with soil-water storage in the order of T2 < T1 < T3 < T4. Water storage in T3 plot was always close to that in T4 plot. This might be due to weak water consumption by the naturally restored vegetation in the initial restoration stage. The results further indicated that soil-water storage was greatly influenced by the artificial vegetation, especially at the later growing stages.
Table III. Soil-water storage and standard deviation within the 400-cm depth in four land-use types.
The inter-annual soil-water storage within the 400 cm depth of the four types of land use was assessed with coefficients of variation shown in . Heterogeneities for both the artificial vegetations in 2005 were considered to be moderate due to moderate drought, whereas they were considered lower in other years. The heterogeneities for T3 and T4 plots were not so obvious during the study period. The magnitude of variation in T1 and T2 plots was always greater than in T3 and T4 plots, except in the initial year of planting. However, the magnitude of soil-water storage differences under four types of land use tended to inversely relate to the drought. As the annual precipitation decreased, coefficients of variation in soil-water storage were increased in T1 and T2 plots, and decreased in T3 and T4 plots. The discrepancy seems reasonable, when one considers the fact that the little precipitation and continuous evaporation caused the top layer of soil to be extremely dry, which could lead to weak evaporation thereafter, whereas, in the deeper layers of soil, water was consumed continuously through root uptake. The amount of water consumed by the roots was much more than the reduction of surface evaporation by canopy. As compared with the bare land, artificial caragana and artificial alfalfa indicated more extensive water consumption, while the naturally restored vegetation tended to have relatively weak consumption for soil water.
Profile variation of soil water in four types of land use
shows five periods selected to represent the annual soil-water variation in the soil profile. The trend for the four types of land use were similar (in terms of distribution feature) at the first stages, but gradually showed differences at the later stages. Soil water within the upper shallow layers in the profile showed a low-high-low fluctuation in T1, T2, and T3 plots covered with vegetation, and continuously increased in T4 plot without any plant. This might be due to an interaction of precipitation and evapotranspiration. The deepest depth of soil water increasing could be considered as the maximum rainfall infiltration depth. The maximum rainfall infiltration depths under four types of land use differed as follows: 80 cm in the T1 and T2 plots, 60 cm in the T3 plot, and 100 cm in the T4 plot. Two factors could be considered to be involved in the process. First, different vegetation types would themselves result in differences of infiltration. Second, in the shallow layer of dry soil with vegetations, more water could be stored, which could cause larger differences of soil-water redistribution within the upper layers.
Figure 4. Profile distributions of soil-water content in four types of land use. Standard-deviation values are indicated for the 42 sampling times.
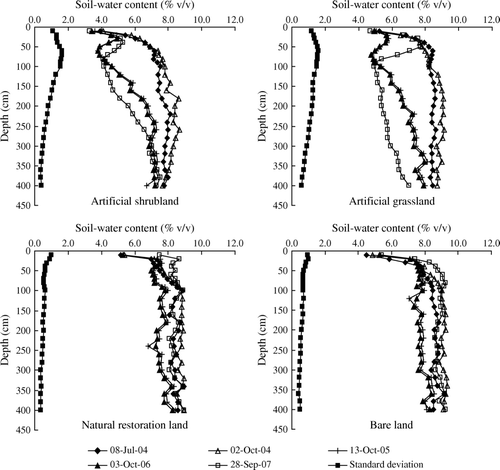
Under the maximum rainfall infiltration depth, soil water in the profile showed two different patterns: a stabilized pattern in T3 and T4 plots and an increasing pattern in T1 and T2 plots. Soil water in the profile was generally stable with the changes of annual precipitation during the experimental periods in T3 and T4 plots. Soil water in the profile of T3 and T4 plots changed similarly, within a relatively small range of decreases under drought through the entire profile in dry years and recoverable with sufficient precipitation supply in normal years. Soil water in the profile of T1 and T2 plots was constant prior to the spring of 2005, but decreased thereafter to a depth below 80 cm. These results could be attributed to different roots distribution in the profile that caused differences in water uptake by roots and changes of soil physical properties such as bulk density and porosity with depth (Zhu, Citation1993).
Standard deviation of soil water measured with samples taken 42 times from the profile is presented in . Soil water in the upper layers of soil profile was highly variable, primarily due to precipitation infiltration, soil-surface evaporation, and water redistribution as reported earlier (Barling et al., Citation1994). Owing to extensive surface evaporation, soil water in T3 and T4 plots fluctuated greatly in the top 10 cm. In T1 and T2 plots, the greatest variation of soil water occurred at the 60-cm depth due to root uptake. In profile, soil water in the naturally restored vegetation land and the bare land tended to have a mild and similar change. The naturally restored vegetation consistently had weak water consumption in the deeper layers during the early stages of restoration, mainly due to the distribution of relatively shallow roots of the annual pioneer species. However, water-consumption patterns seemed quite strong but different between the two types of artificial vegetation. The artificial caragana showed greater water-consumption intensity in relatively shallow layers while the artificial alfalfa exhibited a relatively weak intensity of water consumption in deeper layers. For example, soil-water content in the artificial caragana land reached 4% at the 80 cm depth and was less than in the artificial alfalfa land in 2006, but in the artificial caragana land the impact depth was much shallower. As observed in our study, soil water of both artificial vegetation lands was consumed in the entire soil profile in dry years, whereas it was recharged in the shallow layers. Under the maximum rainfall-infiltration depth, soil water was continuously decreased and exhibited desiccation unless under a plentiful water supply. Therefore, both artificial vegetations were expected to grow with lower density.
Spatial variation of soil-water in four types of land use
Distributions of mean soil-water content along the slope are shown in . Soil water fluctuated along the slope and showed a high-low-high-low pattern. There seemed to be an increase of soil water along the slope between 15 m and 50 m from the top and a decrease in the top 15 m and bottom 10 m of the slope for all types of land use. This result was partially consistent with the previous findings that soil water increased from top to foot along a slope, due to precipitation redistribution (Famiglietti et al., Citation1998; Qiu et al., Citation2001). The differences were probably due to the marginal effect between plots where the upper edge of the plot was beneficial to reduce runoff. The soil profile at the bottom of the slope was exposed by runoff pools, which caused extra water consumption by profile evaporation and lateral movement. Therefore, the influence of marginal effects should be taken into account. Otherwise, it might introduce errors if small-scale plots were used. As shown in , soil water tended to show similar changes along the slope under all types of land use over time. Soil-water distribution was relatively stable along the slope.
shows similar values for the mean soil-water content that were observed at different slope positions, with the trend of upper < middle < lower under the four types of land use. However, soil water varied greatly by the types of land use. Plots T1 and T2 showed moderate variation in soil water, while plots T3 and T4 showed weak variation in soil water. Coefficients of soil-water variation under all types of land use increased along the slope and had similar trends as that of mean soil-water content (upper < middle < lower). The difference could possibly be related to the differences in soil-water infiltration, redistribution, and plant-growing status ().
Table IV. Soil-water content and annual crop yield at different slope positions in 2006.
Effects of soil and water conservation in four types of land use
The study region suffers from severe water erosion in humid rainy seasons and from wind erosion in dry seasons. Runoff and soil erosion during the experimental period are shown in and , respectively. The relative amounts of runoff generated were about 7 in 2004, 1 in 2005, 4 in 2006, and 2 in 2007. The runoff events principally resulted from overland flow where the rainfall intensity exceeded the soil-infiltration rate, which indicated that the runoff would not occur while the infiltration threshold is not exceeded by vegetation cover in a rain event (Fu et al., Citation2003). The runoff coefficients under the four types of land use were similar in the initial planting year but different in the succeeding years. Two artificial vegetations could be beneficial in reducing runoff after the planting year. In addition, the runoff coefficients followed the order T1 < T2 < T3 < T4 in the later years. also indicates that runoff increased as precipitation amounts increased under similar precipitation intensity (see the runoffs on July 13, 28, and August 2 of 2004); runoff increased as precipitation intensity increased under similar precipitation amounts (see the runoffs on July 26, 28, and August 11 of 2004); runoff decreased with vegetation growth under similar precipitation amount and intensity (see the runoffs on July 13 of 2004, August 23 of 2005, and July 24 of 2007); runoff decreased with lower initial surface soil-water content under similar rainfall characteristics (see the runoff on August 21 of 2004, and June 23 of 2006). Totalled 201.23 mm and 12.10 mm of rainfall (), respectively, fell within 30 days before the runoff events, caused different initial water conditions in surface soil. The lower initial water conditions implied a higher potential for infiltration. Generally, runoff was comprehensively influenced by precipitation amount, precipitation intensity, surface cover, and initial surface soil-water content.
Table V. Runoffs in four land-use types during the experimental period.
The soil-water erosion was closely related to runoff events as shown by comparison of and . shows the water erosion during the experimental periods. The soil erosion in T1, T3, and T4 plots reached a strong modulus (5000–8000 t·km−2·a−1) and that for T2 plot reached an extremely strong modulus (8000–15,000 t·km−2·a−1) in 2004. The erosion modules in T1 and T2 plots were higher than in T3 and T4 plots in the initial year of planting. There are two possible explanations. First, the differences in erosion resistance among various vegetation lands were not obviously in their initial phase. Second, previous planting treatments by plowing in artificial vegetation lands may contribute to the increase of soil erosion. In our studies, hole-plowing was better than furrow-plowing, due to the fewer disturbances created. However, soil erosion reduced the efficiency of artificial vegetations after their maturity. For example, little soil was eroded in 2006 in T1 and T2 plots, but soil erosion reached 9942.9 t·km−2·a−1 (extremely strong modulus, 8000–15,000 t·km−2·a−1) in T3 plot and 17348.6 t·km−2·a−1 (severe level modulus, >15,000 t·km−2·a−1) in T4 plot. Both artificial vegetation lands showed better soil-conservation effect than did the naturally restored vegetation land. Furthermore, we found that most of the soil erosion was caused by one or two heavy rainfalls, with the rainfall intensity as reported by many authors (Fraser et al., Citation1999; Abu-Hammad et al., Citation2006).
Table VI. Water erosion in four land-use types during the experimental period.
The loose soil surface in the study area is easily eroded by wind as the dry season arrives, especially in the spring after thawing, because soil is mostly bare elsewhere, with higher-velocity winds and drier soil surfaces (Buschiazzo et al., Citation2007). Monthly statistical values of wind from October 2006 to June 2007 are shown in , with the maximum daily average wind speed represented during the spring of 2007 and the prevailing wind direction towards the southeast. shows the wind erosion from October 2006 to June 2007. Total collected sand indicates that land with vegetation more effectively reduced the wind erosion as compared with bare land. The wind erosion in T1, T2, and T3 plots roughly accounted for 1, 10, and 50% of the total wind erosion in T4 plot. Artificial caragana shrubs showed best wind-erosion resistance due to their relatively luxuriant and stronger branches. Artificial alfalfa grass showed relatively weak wind-erosion resistance because the cutting on October 5, 2006 reduced above-ground biomass. The naturally restored vegetation mainly dominated by annuals in the first few years showed the weakest wind-erosion resistance due to poor above-ground cover. The vegetation cover could reduce soil wind erosion by decreasing wind speed and reducing sediment in the air. Results in also shows that the sediments of Aeolian transport decreased exponentially with height without vegetation cover in T4 plot. The vegetation cover increased the thickness of maximum sediment located by the barrier effects of the canopy. The thickness mentioned above in four types of land use followed the order T1 > T2 > T3 > T4 due to the height difference of the canopy.
Table VII. The monthly statistical values of wind from October 2006 to June 2007.
Table VIII. Wind erosion in four land-use types during the experimental period*.
Generally speaking, rainfall rarely occurs from November to March on the Losses Plateau, and most precipitation levels are high from April to September, but vary in an inter-annual cycle. The water showed weak variation in all lands in either an average year or a drought year during the dry periods. Variation among four types of land use was kept roughly stable during the periods. However, soil water changed intensively during the rainy periods with various types of land use. The variation of soil water was higher in both artificial vegetation lands than in two others. The values of net infiltration to keep soil water balance obtained in this study region were 345.9, 355.7, 321.5, and 316.5 mm, respectively, which could keep soil water balance in T1 to T4 plots in the study region. Artificial caragana and artificial alfalfa showed intense consumption for soil water, while naturally restored vegetation consumed less. In soil profile, soil water was mainly affected by rainfall and evapotranspiration in the shallow layers. In deeper layers, however, soil water was principally influenced by water uptake by the roots. Artificial caragana and artificial alfalfa showed intense consumption for soil water, while naturally restored vegetation consumed less. In soil profile, soil water was mainly affected by rainfall and evapotranspiration in shallow layers. In deeper layers, however, soil water was primarily influenced by root uptake. Artificial caragana showed greater water consumption in shallower layers, while artificial alfalfa exhibited weaker consumption at deeper depths. The naturally restored vegetation always showed weak water consumption in deeper layers during the early stage of restoration. Under the maximum rainfallinfiltration depth, soil-water content continuously decreased due to the stronger water consumption by artificial vegetation, and soil became dry unless plenty of water was supplied. Soil-water content and its variability were increased along the slope and its variation was stable over time. The marginal effect obviously influenced soil water in the top and bottom sections of the experimental plots. Hence the location effect should be taken into account for small size plots.
Vegetation cover could reduce runoff and erosion efficiently. As compared with T4 plot, artificial caragana showed the best erosion resistance due to its relatively luxuriant and stronger branches, artificial alfalfa showed great resistance to water erosion in the rainy season due to its high coverage, but the cutting weakened its wind-erosion resistance to a certain extent. The naturally restored vegetation always showed weak soil- and water-conservation effects in the initial stages due to less above-ground biomass.
The results from this study suggest that the vegetation distribution along the slope in the restoration process should alternate by artificial and naturally restored vegetation with the intercropping pattern to retain soil water, as well as to strengthen soil-erosion resistance. The naturally restored vegetation was primarily suitable on the upper slope to maintain soil water, and artificial grass (alfalfa) or artificial shrub (caragana) should be planted at the lower slope to reduce runoff and soil erosion.
Acknowledgements
The study was financially supported by the Innovation Team Program of Chinese Academy of Sciences, the program for Innovative Research Team in University (No. IRT0749) and National Science and Technology Supporting Project (2007BAD381303).
References
- Abu-Hammad , A.H. , Børresen , T. and Haugen , L.E. 2006 . Effects of rain characteristics and terracing on runoff and erosion under the Mediterranean . Soil and Tillage Research , 87 : 39 – 47 .
- Barling , R.D. , Moore , I.D. and Grayson , R.B. 1994 . A quasi-dynamic wetness index for characterizing the spatial distribution of zones of surface saturation and soil water content . Water Resource Research , 30 : 1029 – 1044 .
- Bell , K.R. , Blanchard , B.J. , Schmugge , T.J. and Witczak , M.W. 1980 . Analysis of surface moisture variation within large field sites . Water Resources Research , 16 : 796 – 810 .
- Bochet , E. , Rubio , J.L. and Poesen , J. 1998 . Relative efficiency of three representative matorral species in reducing water erosion at the microscale in a semi-arid climate (Valencia, Spain) . Geomorphology , 23 : 139 – 150 .
- Bochet , E. , Poesen , J. and Rubio , J.L. 2006 . Runoff and soil loss under individual plants of a semi-arid Mediterranean shrubland: influence of plant morphology and rainfall intensity . Earth Surface Processes and Landforms , 31 : 536 – 549 .
- Boix-Fayos , C. , Calvo-Cases , A. , Imeson , A.C. , Soriano-Soto , M.D. and Tiemessen , I.R. 1998 . Spatial and short-term temporal variations in runoff, soil aggregation and other soil properties along a Mediterranean climatological gradient . Catena , 33 : 123 – 138 .
- Bosch , D.D. , Lakshmi , V. , Jackson , T.J. , Choi , M. and Jacobs , J.M. 2006 . Large scale measurements of soil moisture for validation of remotely sensed data: Georgia soil moisture experiment of 2003 . Journal of Hydrology , 323 : 120 – 137 .
- Brocca , L. , Morbidelli , R. , Melone , F. and Moramarco , T. 2007 . Soil moisture spatial variability in experimental areas of central Italy . Journal of Hydrology , 333 : 356 – 373 .
- Burt , T.P. and Butcher , D.P. 1985 . Topographic controls of soil moisture distribution . Journal of Soil Science , 36 : 469 – 486 .
- Buschiazzo , D.E. , Zobeck , T.M. and Abascal , S.A. 2007 . Wind erosion quantity and quality of an Entic Haplustoll of the semi-arid pampas of Argentina . Journal of Arid Environments , 69 : 29 – 39 .
- Chen , L.D. , Huang , Z.L. , Gong , J. , Fu , B.J. and Huang , Y.L. 2007 . The effect of land cover/vegetation on soil water dynamic in the hilly area of the Loess Plateau, China . Catena , 70 : 200 – 208 .
- Cosh , M.H. , Jackson , T.J. , Bindlish , R. and Prueger , J.H. 2004 . Watershed scale temporal and spatial stability of soil moisture and its role in validating satellite estimates . Remote Sensing of Environment , 92 : 427 – 435 .
- Cosh , M.H. , Jackson , T.J. , Starks , P. and Heathman , G. 2006 . Temporal stability of surface soil moisture in the Little Washita River watershed and its applications in satellite soil moisture product validation . Journal of Hydrology , 323 : 168 – 177 .
- Crave , A. and Gascuel-odux , C. 1997 . The influence of topography on the time and space distribution of soil surface water content . Hydrological Processes , 11 : 203 – 210 .
- Cubera , E. and Moreno , G. 2007 . Effect of land-use on soil water dynamic in dehesas of Central-Western Spain . Catena , 71 : 298 – 308 .
- Famiglietti , J.S. , Rudnicki , J.W. and Rodell , M. 1998 . Variability in surface moisture content along a hillslope transect: Rattlesnake Hill, Texas . Journal of Hydrology , 210 : 259 – 281 .
- Fraser , A.I. , Harrod , T.R. and Haygarth , P.M. 1999 . The effect of rainfall intensity on soil erosion and particulate phosphorus transfer from arable soils . Water Science and Technology , 39 : 41 – 45 .
- Fu , B.J. and Gulinck , H. 1994 . Land evaluation in area of severe erosion: the Loess Plateau of China . Land Degradation and Rehabilitation , 5 : 33 – 40 .
- Fu , B.J. , Chen , L.D. , Ma , K.M. , Zhou , H.F. and Wang , J. 2000 . The relationships between land use and soil conditions in the hilly area of the Loess Plateau in northern Shannxi, China . Catena , 39 : 69 – 78 .
- Fu , B.J. , Wang , J. , Chen , L.D. and Qiu , Y. 2003 . The effects of land use on soil moisture variation in the Danangou catchment of the Loess Plateau, China . Catena , 54 : 197 – 213 .
- Gómez-Plaza , A. , Alvarez-Rogel , J. , Albaladejo , J. and Castillo , V.M. 2000 . Spatial patterns and temporal stability of soil moisture across a range of scales in a semi-arid environment . Hydrological Processes , 14 : 1261 – 1277 .
- Grant , L. , Seyfried , M. and McNamara , J. 2004 . Spatial variation and temporal stability of soil water in a snow-dominated, mountain catchment . Hydrological Processes , 18 : 3493 – 3511 .
- Grayson , R.B. , Moore , I.D. and McMahon , T.A. 1992 . Physically based hydrologic modeling 1. A terrain-based model for investigative purposes . Water Resource Research , 28 : 2639 – 2658 .
- Grayson , R.B. , Western , A.W. , Chiew , F.H.S. and Blöschl , G. 1997 . Preferred states in spatial soil moisture patterns: local and nonlocal controls . Water Resource Research , 33 : 2897 – 2908 .
- Hu , L.J. , & Shao , M.A. 2002 . Review on water eco-environment in vegetation restoration in the Loess Plateau . Chinese Journal of Applied Ecology , 13 , 1045 1048 (in Chinese) .
- Hupet , F. and Vanclooster , M. 2005 . Micro-variability of hydrological processes at the maize row scale: implications for soil water content measurements and evapotranspiration estimates . Journal of Hydrology , 303 : 247 – 270 .
- Kimura , R. , Fan , J. , Zhang , X.C. , Takayama , N. , Kamichika , M. and Matsuoka , N. 2006 . Evapotranspiration over the Grassland field in the liudaogou basin of the Loess Plateau, China . Acta Oecologica , 29 : 45 – 53 .
- Li , J. , Chen , B. , Li , X.F. , Shao , M.A. , & Cheng , J.M. 2007 . Water potential productivity and soil desiccation effect of alfalfa grasslands in different type arid areas of Loess Plateau: A simulation study . Chinese Journal of Applied Ecology , 18 , 2418 2425 (in Chinese) .
- Li , J. , Chen , B. , Li , X.F. , Zhao , Y.J. , Ci-Ren , Y.J. , Jiang , B. , & Hu , W. 2008 . Soil desiccating effects of forestlands, grasslands and croplands in zones different in rainfall pattern on the Loess Plateau . Acta Pedologica Sinica , 45 , 40 49 (in Chinese) .
- Lin , H.S. , Kogelmann , W. , Walker , C. and Bruns , M.A. 2006 . Soil moisture patterns in a forested catchment: a hydropedological perspective . Geoderma , 131 : 345 – 368 .
- Martínez-Fernández , J. and Ceballos , A. 2003 . Temporal stability of soil moisture in a large-field experiment in Spain . Soil Science Society of America Journal , 67 : 1647 – 1656 .
- Martínez-Fernández , J. and Ceballos , A. 2005 . Mean soil moisture estimation using temporal stability analysis . Journal of Hydrology , 312 : 28 – 38 .
- Nash , M.S. , Wierenga , P.J. and Gutjahr , A. 1991 . Time series analysis of soil moisture and rainfall along a line transect in arid rangeland . Soil Science , 152 : 189 – 198 .
- Pachepsky , Y. , Guber , A. and Jacques , D. 2005 . Temporal persistence in vertical distributions of soil moisture contents . Soil Science Society of America Journal , 69 : 347 – 352 .
- Petrone , R.M. , Price , J.S. , Carey , S.K. and Waddington , J.M. 2004 . Statistical characterization of the spatial variability of soil moisture in a cutover peatland . Hydrological Processes , 18 : 41 – 52 .
- Qiu , Y. , Fu , B.J. , Wang , J. and Chen , L.D. 2001 . Soil moisture variation in relation to topography and land use in a hillslope catchment of the Loess Plateau, China . Journal of Hydrology , 240 : 243 – 263 .
- Ran , Q. , Zhang , Z.X. , Zhang , G.P. , & Zhou , Q.B. 2005 . DEM correction using TVDI to evaluate soil moisture status in China . Science of Soil and Water Conservation , 3 , 32 36 (in Chinese) .
- Reynolds , S.G. 1970 . The gravimetric method of soil moisture determination. I: A study of equipment, and methodological problems . Journal of Hydrology , 11 : 258 – 273 .
- Schume , H. , Jost , G. and Katzensteiner , K. 2003 . Spatio-temporal analysis of the soil water content in a mixed Norway spruce (Picea abies L. Karst.)-European beech (Fagus sylvatica L.) stand . Geoderma , 112 : 273 – 287 .
- Starks , P.J. , Heathman , G.C. , Jackson , T.J. and Cosh , M.H. 2006 . Temporal stability of soil moisture profile . Journal of Hydrology , 324 : 400 – 411 .
- Tang , K.L. , Hou , Q.C. , Wang , B.K. , & Zhang , P.C. 1993 . The environment background and administration way of wind-water erosion crisscross region and Shenmu experimental area on the Loess Plateau . Research of Soil and Water Conservation , 18 , 2 15 (in Chinese) .
- The State Bureau of Technical Supervision 2006 . Meteorological drought indices, GB/T 20481-2006 , Standards Press of China (in Chinese) .
- Thierdefelder , T.K. , Grayson , R.B. , van Rosen , D. and Western , A.W. 2003 . Inferring the location of catchment characteristics soil moisture monitoring sites, Covariance structure in the temporal domain . Journal of Hydrology , 280 : 13 – 32 .
- Wahl , N.A. , Bens , O. , Schäfer , B. and Hüttl , R.F. 2003 . Impact of changes in land-use management on soil hydraulic properties: hydraulic conductivity, water repellency and water retention . Physics and Chemistry of the Earth , 28 : 1377 – 1387 .
- Wang , P.X. , Wan , Z.M. , Gong , J.Y. , Li , X.W. , & Wang , J.D. 2003a . Advances in drought monitoring by using remotely sensed normalized difference vegetation index and land surface temperature products . Advance in Earth Sciences , 18 , 527 533 (in Chinese) .
- Wang , Z.Q. , Liu , B.Y. , & Lu , B.H. 2003b . A study on water restoration of dry soil layers in the semi-arid area of Loess Plateau . Acta Ecologica Sinica , 23 , 1944 1950 (in Chinese) .
- Wei , L.H. , Zhang , B. and Wang , M.Z. 2007 . Effects of antecedent soil moisture on runoff and soil erosion in alley cropping systems . Agricultural Water Management , 94 : 54 – 62 .
- Western , A.W. and Grayson , R.B. 1998 . The tarrawarra data set: Soil moisture patterns, soil characteristics, and hydrological flux measurements . Water Resources Research , 34 : 2765 – 2768 .
- Western , A.W. , Grayson , R.B. , Blöschl , G. , Willgoose , G.R. and McMahon , T.A. 1999 . Observed spatial organization of soil moisture and its relation to terrain indices . Water Resources Research , 35 : 797 – 810 .
- Zhang , Y.K. and Schilling , K.E. 2006 . Effects of land cover on water table, soil moisture, evapotranspiration, and groundwater recharge: A Field observation and analysis . Journal of Hydrology , 319 : 328 – 338 .
- Zhu , X.M. 2000 . Environment in Loess Plateau and “Pedogenic Reservoir” . Quaternary Sciences , 20 : 514 – 520 .
- Zhu , X.M. 2006 . Rebuild soil reservoir is a rational approach for soil and water conservation on the Loess Plateau . China Academic Journal , 21 : 320 – 324 .
- Zhu , Z.C. 1993 . The main characteristics of the vegetation and its impact on the soil essence in the Loess Plateau of northern Shaanxi Province . Acta Phytoecologica et Geobotanica Sinica , 17 , 280 286 (in Chinese) .