Abstract
Subretinal fibrosis has been recognized as a feature of an advanced stage of exudative age-related macular degeneration (AMD) that leads to irreversible loss of vision. This study was aimed at elucidating roles of interlukin-6 (IL-6) in the development of subretinal fibrosis. Immunohistochemistry (IHC) was performed with anti-human IL-6 antibody in surgically excised choroidal neovascular tissues from patients with exudative AMD. The area of subretinal fibrosis was measured in a mouse subretinal fibrosis model with injection of control small interfering RNA(siRNA) or IL-6 siRNA, or isotype control antibody or anti-IL-6 receptor antibody after peritoneal exudative cells (PECs) injection into the vitreous cavity. PECs derived from IL-6+/+ or IL-6−∕− mice were placed into the subretinal space of IL-6+/+ mice. IL-6 was expressed in the stroma and retinal pigment epithelial (RPE) layer in the choroidal neovascular tissues. IL-6 knockdown or blocking of the IL-6 receptor suppressed the formation of subretinal fibroblastic scars. The area of subretinal fibrosis induced by PECs derived from IL-6−∕− mice was less than that induced by PECs from IL-6+/+ mice. The results suggested that IL-6, expressed by activated macrophages, is a crucial mediator that promotes subretinal fibrosis. Targeting IL-6 and the corresponding signaling pathway would be an attractive therapeutic approach not only in choroidal neovascularization, but also in subretinal fibrosis.
1. Introduction
Age-related macular degeneration (AMD) is the most common cause of severe vision loss in developed countries [Citation1]. Most blindness in AMD results from choroidal neovascularization (CNV), which is the result of aberrant new vessels growing from the pre-existing choroidal vasculature, breaking through the Bruch’s membrane and extending into the subretinal pigment epithelial space and/or subretinal space [Citation2]. The mechanisms of CNV are still poorly understood in most conditions; however, the extent to which a wound healing response contributes the initiation of a CNV response is completely unclear [Citation3,Citation4].
Tissue fibrosis is a common pathophysiological response to chronic injury and can be considered as a wound repair of damaged organs, mostly associated with a robust inflammatory response [Citation5]. CNV causes hemorrhage and extravasation of plasma proteins, including those of the extracellular matrix, such as fibrinogen that clots to form a fibrin gel. Infiltration of inflammatory cells into the fibrin gel, the transdifferentiation of retinal pigment epithelial (RPE) cells into fibroblast-like cells via epithelial-mesenchymal transition (EMT) and an excessive and poorly ordered extracellular matrix deposition often result in the formation of subretinal fibrovascular tissue, which replaces the normal retinal structure including photoreceptors, within and/or around the macula area. Furthermore, subretinal fibrosis develops in approximately 50% of all treated eyes within two years post continuous anti-vascular endothelial growth factor (VEGF) treatment, which is the first choice for treatment in patients with wet AMD, which results in visual impairment [Citation6,Citation7]. Because patients with this condition show severe permanent visual loss, both the elucidation of the molecular mechanisms underlying tissue scar formation in CNV and the development of therapeutic strategies for tissue fibrosis are desired.
Several studies have reported that activated macrophages play a pivotal role in the development of tissue fibrosis in other organs such as the liver and kidney [Citation8,Citation9]. In the eye, macrophage infiltration is also thought to be an important pathological process in subretinal proliferation in retinal diseases, because macrophages can produce fibrogenic, proliferation and angiogenic factors [Citation10]. Recently, we showed that injection of isolated peritoneal exudates cells (PECs) into the subretinal space can promote EMT in RPE cells and subsequently result in subretinal scar formation [Citation11], which indicated that macrophages may trigger subretinal fibrosis.
Interleukin (IL)-6 is a pleiotrophic cytokine that plays a role in biological processes such as immune response, inflammation, wound healing response and angiogenesis [Citation12]. IL-6 binds to the IL-6–binding chain receptor (IL-6 R) and the complex of IL-6 and IL-6 R associates with signal-transducing chain gp130 to result in homodimerization of gp130 to trigger the IL-6 signaling pathway [Citation13]. Then, the dimerization of gp130 leads to activation of the Janus kinase (JAK)-signal transducer and activator of transcription 3 (STAT3) and the JAK-extracellular signal-regulated kinase (ERK) pathway. Persistent aberrant IL-6 production has been implicated in the development of various autoimmune and chronic inflammatory diseases as well as in cancers [Citation14]. IL-6 has also been found to be associated with many fibrotic diseases including idiopathic lung fibrosis, hepatocellular fibrosis, renal fibrosis, graft-versus-host disease (GVHD), liver fibrosis, keloids and systemic sclerosis [Citation15]. In a previous study, analyses of mice deficient in IL-6 revealed an impaired wound healing response in several organs, including the liver and the skin [Citation16,Citation17]. However, this impaired cutaneous wound healing response in the IL-6 deficient mice was recovered upon IL-6 administration, indicating that IL-6 might be essential for wound healing [Citation17]. In human AMD, high levels of IL-6 in the blood samples of patients with exudative AMD have been correlated with disease progression [Citation18]. Moreover, in a murine laser-induced CNV model, IL-6 has been reported to induce VEGF and ccl2 expression in vascular endothelial cells, leading to the development of CNV [Citation19]. Furthermore, transforming growth factor β2 (TGFβ2), which promotes the EMT of RPE cells [Citation20], was found to induce subretinal fibrosis as well as IL-6 production in RPE cells [Citation21]. Based on these findings, we predicted that the analysis of the relationship between subretinal fibrosis and IL-6 using our recently developed animal model could provide new insights into the mechanisms of subretinal fibrosis [Citation11]. Therefore, in this study, we investigated the role of IL-6 in mice by genetic ablation and its potential as a therapeutic target using short-interfering RNA (siRNA) against IL-6 and neutralizing anti-IL-6 receptor antibody in a murine subretinal fibrosis model.
2. Materials and methods
2.1. Human tissue
This study was conducted in accordance with the guidelines of the Declaration of Helsinki and approved by the Kyushu University Institutional Review Board for Clinical Research. We obtained written informed consent from all the participants before any study procedures or examinations were performed. CNV tissue was excised from patients with AMD who had not received previous AMD treatment at the Department of Ophthalmology, Nihon University Hospital. The diagnoses were confirmed by dilated ophthalmic examination prior to acquisition of the tissues or eyes. The study follows the guidelines of the Declaration of the Helsinki.
2.2. Mice
Female C57BL/6 J (IL-6+/+) mice and IL-6−∕− mice with a C57BL/6 J background between 7 and 10 weeks of age were purchased from Japan SLC (Shizuoka, Japan) or Jackson Laboratories (Bar Harbor, ME, USA). All the animal experiments were approved by the committee on the Ethics of Animal Experiments, Kyushu University, Graduate School of Medical Sciences. All the animals were maintained in specific pathogen-free conditions at Kyushu University and treated humanely according to the Association for Research in Vision and Ophthalmology (ARVO) Statement for the Use of Animals in Ophthalmic and Vision Research.
2.3. Immunohistochemistry (IHC)
Paraffin-embedded sections of human eyes or surgically excised CNV tissues were deparaffinized and rehydrated with a graded alcohol series. Immunohistochemical staining with the primary antibodies specific for human IL-6 (Santa Cruz Biotechnology, Santa Cruz, CA, USA) was performed using horseradish peroxidase. 3,3'-Diaminobenzidine (DAB) was used as a chromogen with the Vectastain ABC kit (Vector Laboratories, Burlingame, CA, USA). Hematoxylin (Dako, Glostrup, Denmark) was used for counterstaining.
2.4. Preparation of PECs
Macrophage-rich peritoneal exudative cells (PECs) were obtained from mice that received 2.5 mL of 3% aged thioglycolate solution (Difco, Detroit, MI, USA) three days before killing. The PECs were placed in 10 cm dishes, from which the nonadherent cells were washed out using phosphate buffered saline (PBS). Next, the remaining adherent cells were incubated with 0.02% ethylenediaminetetraacetic acid (EDTA) and were collected by vigorous pipetting. The cells were then counted and resuspended in PBS.
2.5. Mouse model of subretinal fibrosis
Mouse models of subretinal fibrosis were established as described previously [Citation11]. Briefly, laser photocoagulation (wavelength 630 nm, 0.1 s, spot size 75 μ, and power 200 mW) was performed in the posterior pole of retina around the disc to create a subretinal bubble and rupture Bruch’s membrane. PECs (4 × 107) collected as described above without any stimulation were then inoculated into the subretinal space. Neutralizing anti-mouse IL-6 receptor antibody (MR16-1) which was kindly provided by Chugai Pharmaceutical Co. (Tokyo, Japan) or an isotype-matched control rat IgG was injected into the vitreous cavity two hours after PEC-inoculation. On day 7 after PEC-inoculation, the mice were killed and their eyes were enucleated; the eyes were then fixed in 4% paraformaldehyde. Next, choroid flatmount were prepared and stained with anti-glial fibriary acidic protein (GFAP) antibody conjugated with fluorescein isothiocyanate (FITC) (Dako). GFAP was used as a marker of subretinal fibrosis in this model, because the area that glia cells accumulated in coincided with the subretinal fibrosis area stained with collagen type I, a direct marker of fibrosis. However, direct collagen type I staining could not constantly detect the fibrotic area in this model [Citation11]. Images were captured using a fluorescence microscope (DMIRE2; Leica). GFAP-positive area was measured using Image J software (NIH, Bethesda, MD, USA).
2.6. Intravitreal injection of siRNA
IL-6-targeting siRNA and control siRNA were purchased from Thermo Fisher Scientific (Waltham, MA, USA). The siRNAs were resolved in siRNA suspension buffer and were injected into the vitreous cavity just after PEC inoculation in the subretinal fibrosis model.
2.7. Quantitative real-time polymerase chain reaction (RT-PCR)
Total RNA was extracted using Trizol reagent (Invitrogen, Carlsbad, CA, USA) from the eyes of wild type (WT) mice treated with either IL-6-targeting siRNA or control siRNA at day 14 after immunization. Aliquots containing 1 μg of total RNA were then reverse transcribed using a RT-PCR kit (First-Strand complementary DNA (cDNA) Synthesis Kit; Roche Diagnostics GmbH, Mannheim, Germany), according to the manufacturer’s instructions. Next, the cDNA samples were subjected to 40 cycles of amplification with predesigned TaqMan Gene Expression Assays (Applied Biosytems, Foster City, CA, USA) for each target (IL-6: Mm00446190_m1) in a LightCycler Instrument (Roche Diagnostics) and was quantified by the comparative cycling threshold method. Estimated mRNA values were then normalized to glyceraldehyde 3-phosphate dehydrogenase (GAPDH) messenger RNA (mRNA) levels.
2.8. Statistical analysis
Data were analyzed using analysis of variance (ANOVA) and Scheffes test. p < .05 was considered statistically significant. Each histogram represents the mean ± standard deviation (SD) unless otherwise noted.
3. Results
3.1. IL-6 expression was detected in the human fibrotic tissue obtained from AMD patients
IL-6 levels in the aqueous humor in patients with exudative AMD are significantly increased compared to those in the controls [Citation22]. To assess the expression of IL-6 histologically, we performed IHC for negative control or anti-IL-6 antibody on surgically excised choroidal fibrovascular membranes from a patient with exudative AMD who had not received previous AMD treatment. In IHC with the negative control, a partially intact monolayer of RPE cells was observed in the patient (). IL-6 was expressed in the stroma cells and an intact monolayer of RPE cells in the examined specimen ().
Figure 1. Expression of IL-6 in the stroma cells and a partially intact monolayer of RPE cells in human choroidal fibrovascular tissues from a patient with exudative AMD. Immunohistochemistry shows expression of IL-6 (B) in surgically excised AMD choroidal neovascular tissues, in the stroma (red arrowheads) and a partially intact monolayer of RPE cells (yellow arrows). The specificity of staining is confirmed by the absence of staining with isotype control IgG (A). Scale bars, 50 μm.
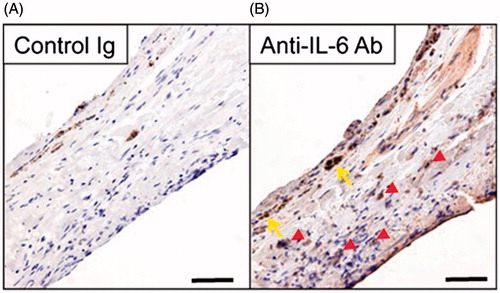
3.2. Neutralization of the IL-6 signaling pathway reduced subretinal fibrosis
Initiation of fibrosis is induced by activated fibroblasts that produce excess collagen and extracellular matrix [Citation23]. The collagen synthesis is induced by the stimulation of IL-6 in hepatic inflammation [Citation24]. IL-6 also enhances the production of tissue inhibitor of metalloproteinases (TIMP), which inhibit enzymatic activity to digest the extracellular matrix [Citation25]. To assess whether the IL-6 signaling pathway involving the IL-6 receptor promoted subretinal fibrosis, we treated the eyes with the anti-IL-6 R antibody (MR16-1) in order to inhibit IL-6 signaling in the eyes of mice with subretinal fibrosis. The area of subretinal fibrosis in anti-IL-6 R antibody-treated mice was significantly lower than that in the control antibody-treated mice ().
Figure 2. Neutralization of IL-6 R suppressed subretinal fibrosis. The area of subretinal fibrosis was reduced upon the intravitreal injection of neutralizing anti-IL-6 R antibody (MR16-1) compared to isotype control IgG (n = 3). The data are shown as means ± SD. The experiment was repeated three times with similar results. *p < .05.
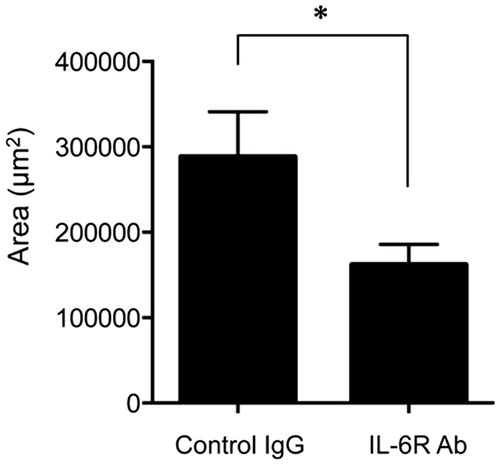
3.3. IL-6 knockdown reduced subretinal fibrosis
Next, to assess the effect of endogenous IL-6 on subretinal fibrosis, we suppressed IL-6 synthesis by using IL-6 siRNA. First, we defined the effective concentration of IL-6 siRNA required to suppress the expression of the IL-6 gene in the eyes of mouse models of subretinal fibrosis. Compared with control siRNA, 49 and 73 ng of IL-6 siRNA significantly reduced the expression of the IL-6 gene in the eyes (). We then performed treatment with 73 ng of IL-6 siRNA or control siRNA in the subretinal fibrosis model and found that the area of subretinal fibrosis in mice injected with IL-6 siRNA was significantly reduced when compared with the mice injected with control siRNA ().
Figure 3. Effects of knockdown of the IL-6 gene on subretinal fibrosis. (A) Expression of the IL-6 gene was assessed in the eyes of WT mice injected with IL-6 siRNA in a dose-dependent manner and were compared with the results for the scramble siRNA-injected eyes in the subretinal fibrosis model (n = 3). (B) The area of subretinal fibrosis was decreased upon intravitreal injection of IL-6-siRNA compared with that observed upon injection of control-siRNA (n = 3). The data are shown as means ± SD. The experiment was repeated three times with similar results. *p < .05.
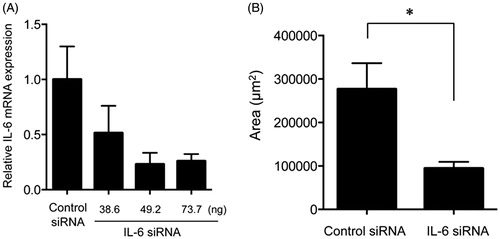
3.4. Subretinal fibrosis was suppressed upon administration of IL-6−∕− PECs
To investigate whether IL-6 derived from macrophages promoted subretinal fibrosis, we injected IL-6+/+ or IL-6−∕− PECs into the subretinal space of IL-6+/+ mice. The area of subretinal fibrosis induced upon injection of IL-6−∕− PECs was significantly decreased when compared with that induced upon injection with of IL-6+/+ PECs (). These results suggested that IL-6 derived from macrophages played a pivotal role in subretinal fibrosis.
Figure 4. Subretinal fibrosis upon administration of IL-6−∕− PECs. The area of subretinal fibrosis in the eyes of the WT mice was reduced upon the subretinal injection of IL-6−∕− PECs when compared with that observed upon injection of IL-6+/+ PECs (n = 3). The data are shown as means ± SD. The experiment was repeated three times with similar results. *p < .05.
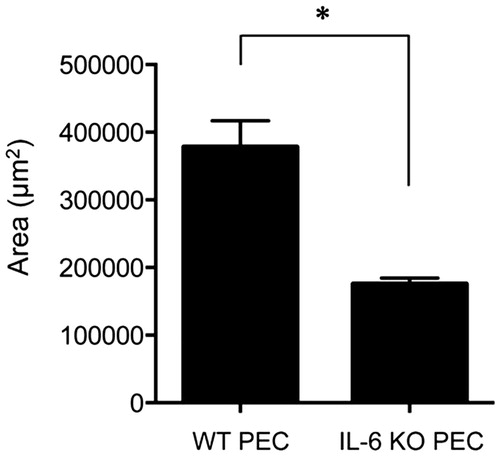
4. Discussion
In this study, we found the IL-6 expression in the stroma and monolayer of RPE cells in patients with exudative AMD. Most of the stromal cells were immunoreactive for both alpha-SMA and cytokeratin, as determined using IHC [Citation26]. In addition, because of a gradient change of RPE cells from cytokeratin-positive, mildly alpha-SMA-positive cells adjacent to the normal RPE layer to non-pigmented cytokeratin-negative, alpha-SMA -positive stroma cells and to cytokeratin-negative, alpha-SMA -positive cells in the stroma, we hypothesized that myofibroblasts in human choroidal fibrovascular tissues originate from RPE cells [Citation26]. Cui et al. [Citation27] reported that IL-6 is increased in the RPE-choroid lesion and that inhibition of IL-6 signaling can suppress subretinal fibrosis in the murine subretinal fibrosis model. These results suggest that IL-6 derived from RPE cells is essential for the development of human choroidal fibrovascular tissues.
In addition, infiltration of CD68-positive macrophages is reported to be abundant in the stroma as well as in RPE cells [Citation28]. Our previous study as well as other previous reports have shown that ocular-infiltrating macrophages play a pivotal role in the development of CNV via CCR2, the receptor of ccl2, in a laser-induced CNV model [Citation29,Citation30]. In the laser-induced CNV model, IL-6 was reported to be able to recruit macrophages into laser-burned sites through the induction of ccl2 [Citation19]. In an analysis of ccl2−∕− mice in our subretinal fibrosis model, we revealed that ccl2 is essential to promote the accumulation of macrophages in the laser-burned area, leading to the development of subsequent subretinal fibrosis [Citation11]. Therefore, lack of IL-6 from injected macrophages reduced the subretinal scar formation in vivo, which suggested that IL-6 produced by infiltrating macrophages play a primary role in ccl2 induction in subretinal fibrosis as well as in CNV.
EMT, which is characterized by the loss of cell-cell junctions and cell polarity, is one of the pivotal process during the development of subretinal scar formation [Citation6]. We previously showed that transforming growth factor-beta 2 (TGF-β2) not only promotes the EMT through the upregulation of alpha-SMA, vimentin and fibronectin, but also promotes IL-6 production in RPE cells [Citation21]. It has also been reported that IL-6 synergistically acts with TGF-β2 to promote EMT through the JAK2/STAT3 pathway and subsequent activation of mothers against decapentaplegic homolog 3 (Smad3) in lung cancer cells [Citation31]. IL-6 has also been found to directly induce EMT through the JAK/STAT3/Snail pathway in head and neck tumor cells [Citation32]. Although the expression of IL-6 R is limited in specific cell lineages, RPE cells express the IL-6 R gene [Citation33], which suggests that IL-6 mediates the EMT in RPE cells, not only in an autocrine loop, but also in a paracrine loop with TGF-β2.
Several researchers also have reported that activation of STAT3 is associated with renal fibrosis and lung fibrosis [Citation34,Citation35]. However, during a wound healing response in the skin, IL-6 induces alpha-SMA expression via the activation of JAK1 and ERK, but not STAT3 phosphorylation, in dermal fibroblasts [Citation36]. Recently, it has been reported that IL-6 R−∕− mice do not show a delay in wound healing response in the skin with the activation of the ERK pathway compared to IL-6−∕− mice [Citation37]. In contrast, blocking of ERK activation using the MEK inhibitor, U0126, in IL-6 R−∕− mice has been found to result in a delay in wound healing response in the skin, which indicated that the ERK pathway also might control a wound healing response in the skin. Consistent with these reports, we found that the decrease in subretinal fibrosis observed upon blockade of IL-6 R using MR16-1 was somewhat lower than that observed in mice deficient in IL-6 and those subjected to gene silencing using IL-6 siRNA in our subretinal fibrosis model. These results indicated that the JAK1-ERK pathway could be related to the regulation of subretinal scar formation. However, further studies are required to clarify which IL-6-dependent signaling pathway is dominant in subretinal fibrosis.
In summary, our results from the present study indicate that IL-6 plays a pivotal role in the development of subretinal fibrosis. Numerous studies on the mechanisms of CNV have been performed and the accumulated knowledge has led to the development of therapies for exudative AMD, such as anti-VEGF therapy, verteporfin photodynamic therapy and combined therapy [Citation38]. Recently, therapeutic strategies for the prevention of subretinal fibrosis in patients with exudative AMD has also been initiated synergistically with anti-CNV therapy by targeting platelet-derived growth factor BB [Citation6]. Therefore, we propose that anti-IL-6 therapy could be effective for both CNV and subretinal fibrosis and that it will help efficiently achieve better visual prognosis in patients with exudative AMD, although it is necessary to confirm its long-term safety and impaired wound healing response in the retina. The potential of therapeutic targeting against subretinal fibrosis also warrants further research in this area.
Acknowledgements
We would like to thank Ms. Michiyo Takahara and Ms. Yukari Mizuno for their excellent technical support for all the experiments.
Disclosure statement
No potential conflict of interest was reported by the authors.
Additional information
Funding
References
- Ambati J, Ambati BK, Yoo SH, et al. Age-related macular degeneration: etiology, pathogenesis, and therapeutic strategies. Surv Ophthalmol. 2003;48:257–293.
- Witmer AN, Vrensen GF, Van Noorden CJ, et al. Vascular endothelial growth factors and angiogenesis in eye disease. Prog Retin Eye Res. 2003;22:1–29
- Friedlander M. Fibrosis and diseases of the eye. J Clin Invest. 2007;117:576–586.
- Kent D, Sheridan C. Choroidal neovascularization: a wound healing perspective. Mol Vis. 2003;9:747–755.
- Stramer BM, Mori R, Martin P. The inflammation-fibrosis link? A Jekyll and Hyde role for blood cells during wound repair. J Invest Dermatol. 2007;127:1009–1017.
- Ishikawa K, Kannan R, Hinton DR. Molecular mechanisms of subretinal fibrosis in age-related macular degeneration. Exp Eye Res. 2016;142:19–25.
- Daniel E, Toth CA, Grunwald JE, et al. Risk of scar in the comparison of age-related macular degeneration treatments trials. Ophthalmology. 2014;121:656–666.
- Diamond JR. Macrophages and progressive renal disease in experimental hydronephrosis. Am J Kidney Dis. 1995;26:133–140.
- Fallowfield JA, Mizuno M, Kendall TJ, et al. Scar-associated macrophages are a major source of hepatic matrix metalloproteinase-13 and facilitate the resolution of murine hepatic fibrosis. J Immunol. 2007;178:5288–5295.
- Lim WK, Chee SP, Sng I, et al. Immunopathology of progressive subretinal fibrosis: a variant of sympathetic ophthalmia. Am J Ophthalmol. 2004;138:475–477.
- Jo YJ, Sonoda KH, Oshima Y, et al. Establishment of a new animal model of focal subretinal fibrosis that resembles disciform lesion in advanced age-related macular degeneration. Invest Ophthalmol Vis Sci. 2011;52:6089–6095.
- Kishimoto T. IL-6: from its discovery to clinical applications. Int Immunol. 2010;22:347–352.
- Murakami M, Hibi M, Nakagawa N, et al. IL-6-induced homodimerization of gp130 and associated activation of a tyrosine kinase. Science. 1993;260:1808–1810.
- Tanaka T, Narazaki M, Kishimoto T. Therapeutic targeting of the interleukin-6 receptor. Annu Rev Pharmacol Toxicol. 2012;52:199–219.
- O'Reilly S, Ciechomska M, Cant R, et al. Interleukin-6, its role in fibrosing conditions. Cytokine Growth Factor Rev. 2012;23:99–107.
- Cressman DE, Greenbaum LE, DeAngelis RA, et al. Liver failure and defective hepatocyte regeneration in interleukin-6-deficient mice. Science. 1996;274:1379–1383.
- Gallucci RM, Simeonova PP, Matheson JM, et al. Impaired cutaneous wound healing in interleukin-6-deficient and immunosuppressed mice. FASEB J. 2000;14:2525–2531.
- Seddon JM, George S, Rosner B, et al. Progression of age-related macular degeneration: prospective assessment of C-reactive protein, interleukin 6, and other cardiovascular biomarkers. Arch Ophthalmol. 2005;123:774–782.
- Izumi-Nagai K, Nagai N, Ozawa Y, et al. Interleukin-6 receptor-mediated activation of signal transducer and activator of transcription-3 (STAT3) promotes choroidal neovascularization. Am J Pathol. 2007;170:2149–2158.
- Saika S, Kono-Saika S, Tanaka T, et al. Smad3 is required for dedifferentiation of retinal pigment epithelium following retinal detachment in mice. Lab Invest. 2004;84:1245–1258.
- Kimura K, Orita T, Liu Y, et al. Attenuation of EMT in RPE cells and subretinal fibrosis by an RAR-gamma agonist. J Mol Med (Berl). 2015;93:749–758.
- Chalam KV, Grover S, Sambhav K, et al. Aqueous interleukin-6 levels are superior to vascular endothelial growth factor in predicting therapeutic response to bevacizumab in age-related macular degeneration. J Ophthalmol. 2014;2014:502174.
- van der Veer WM, Bloemen MC, Ulrich MM, et al. Potential cellular and molecular causes of hypertrophic scar formation. Burns. 2009;35:15–29.
- Choi I, Kang HS, Yang Y, et al. IL-6 induces hepatic inflammation and collagen synthesis in vivo. Clin Exp Immunol. 1994;95:530–535.
- Sato T, Ito A, Mori Y. Interleukin 6 enhances the production of tissue inhibitor of metalloproteinases (TIMP) but not that of matrix metalloproteinases by human fibroblasts. Biochem Biophys Res Commun. 1990;170:824–829.
- Lopez PF, Sippy BD, Lambert HM, et al. Transdifferentiated retinal pigment epithelial cells are immunoreactive for vascular endothelial growth factor in surgically excised age-related macular degeneration-related choroidal neovascular membranes. Invest Ophthalmol Vis Sci. 1996;37:855–868.
- Cui W, Zhang H, Liu ZL. Interleukin-6 receptor blockade suppresses subretinal fibrosis in a mouse model. Int J Ophthalmol. 2014;7:194–197.
- Oh H, Takagi H, Takagi C, et al. The potential angiogenic role of macrophages in the formation of choroidal neovascular membranes. Invest Ophthalmol Vis Sci. 1999;40:1891–1898.
- Sakurai E, Anand A, Ambati BK, et al. Macrophage depletion inhibits experimental choroidal neovascularization. Invest Ophthalmol Vis Sci. 2003;44:3578–3585.
- Tsutsumi C, Sonoda KH, Egashira K, et al. The critical role of ocular-infiltrating macrophages in the development of choroidal neovascularization. J Leukoc Biol. 2003;74:25–32.
- Liu RY, Zeng Y, Lei Z, et al. JAK/STAT3 signaling is required for TGF-β-induced epithelial-mesenchymal transition in lung cancer cells. Int J Oncol. 2014;44:1643–1651.
- Yadav A, Kumar B, Datta J, et al. IL-6 promotes head and neck tumor metastasis by inducing epithelial-mesenchymal transition via the JAK-STAT3-SNAIL signaling pathway. Mol Cancer Res. 2011;9:1658–1667.
- Holtkamp GM, Van Rossem M, de Vos AF, et al. Polarized secretion of IL-6 and IL-8 by human retinal pigment epithelial cells. Clin Exp Immunol. 1998;112:34–43.
- Pang M, Ma L, Gong R, et al. A novel STAT3 inhibitor, S3I-201, attenuates renal interstitial fibroblast activation and interstitial fibrosis in obstructive nephropathy. Kidney Int. 2010;78:257–268.
- Pedroza M, Schneider DJ, Karmouty-Quintana H, et al. Interleukin-6 contributes to inflammation and remodeling in a model of adenosine mediated lung injury. PLoS One. 2011;6:e22667.
- Gallucci RM, Lee EG, Tomasek JJ. IL-6 modulates alpha-smooth muscle actin expression in dermal fibroblasts from IL-6-deficient mice. J Invest Dermatol. 2006;126:561–568.
- Miki K, Miki A, Matsuoka M, et al. Effects of intraocular ranibizumab and bevacizumab in transgenic mice expressing human vascular endothelial growth factor. Ophthalmology. 2009;116:1748–1754.
- Miller JW. Treatment of age-related macular degeneration: beyond VEGF. Jpn J Ophthalmol. 2010;54:523–528.