Abstract
Protein kinases are key regulators of cell function that constitute one of the largest and most functionally diverse gene families. We developed a novel assay system, based on the bimolecular fluorescence complementation (BiFC) technique in Escherichia coli, for detecting transient interactions such as those between kinases and their substrates. This system detected the interaction between OsMEK1 and its direct target OsMAP1. By contrast, BiFC fluorescence was not observed when OsMAP2 or OsMAP3, which are not substrates of OsMEK1, were used as prey proteins. We also screened for interacting proteins of calcium-dependent protein kinase 8 (OsCPK8), a regulator of plant immune responses, and identified three proteins as interacting molecules of OsCPK8. The interaction between OsCPK8 and two of these proteins (ARF-GEF and peptidyl prolyl isomerase) was confirmed in rice cells by means of BiFC technology. These results indicate that our new assay system has the potential to screen for protein kinase target molecules.
Graphical Abstract
A novel assay system, for detecting specific interactions was developed based on the BiFC technique in Escherichia coli.
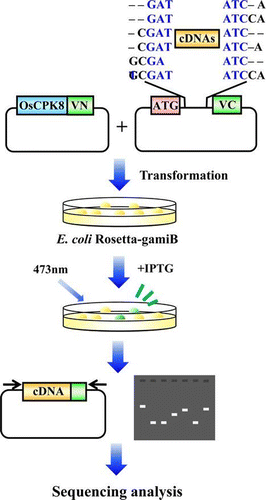
Protein kinases are enzymes that modify substrate proteins by chemical addition of phosphate groups. Plant genomes contain genes for many protein kinases (>1000 in Arabidopsis, >1500 in rice), and 60% of all plant proteins are involved in protein-kinase signaling pathways.Citation1) Kinases comprise a highly conserved family of enzymes that control diverse cellular processes and play key roles in biological systems. Eukaryotic protein kinases are classified into seven major phylogenetic groups.Citation2) The Arabidopsis and rice genomes contain members of six of these groups: AGC, containing protein kinase A (PKA), protein kinase G (PKG), and protein kinase C (PKC); GMGC, containing cyclin-dependent protein kinase (CDK), mitogen-activating protein kinase (MAPK), and glycogen synthase kinase (GSK); CAMK, containing calcium/calmodulin dependent protein kinases; CK1, containing casein kinase 1; TKL, containing tyrosine kinases, mixed-lineage kinases, transforming growth factor-B receptor kinases, and Raf kinases; and STE, containing homologs of yeast STE7, STE11, and STE20 kinases.Citation3)
The activity of a particular protein kinase is usually regulated via phosphorylation by other kinases. Upon phosphorylation by upstream protein kinases, a kinase is activated, transducing its downstream signal.Citation4) Among kinase-cascade pathways in plant cells, the MAPK cascade is one of the major pathways by which extracellular stimuli are transduced into intracellular responses.Citation1,Citation5–7) MAPK and its immediate upstream activators, MAPK kinase (MAPKK) and MAPKK kinase (MAPKKK), constitute the functionally interlinked MAPK cascade. Activated MAPK can phosphorylate a variety of substrates, including transcription factors, other protein kinases, and cytoskeleton-associated proteins.Citation1,8) MAPKs are involved in plant signal transduction in response to pathogens, drought, salinity, cold, wounding, heavy metals, reactive oxygen species, and hormone stimuli.Citation5–7,Citation9–11) A biological stimulus activates a MAPK cascade that consists of specific kinase and substrate molecules. For instance, OsMEK1 interacts with OsMAP1, but not with OsMAP2 or OsMAP3, and the specific interaction between OsMEK1 and OsMAP1, and the phosphorylation of OsMAP1 by OsMEK1 are involved in the signaling pathways that respond to low-temperature stress in rice.Citation12) Moreover, recognition of flagellin by the FLS2 receptor, a leucine-rich repeat (LRR) receptor kinase involved in plant innate immunity, activates the complete plant MAP kinase cascade, consisting of MEKK1, MKK4/MKK5, and MPK3/MPK6, and the WRKY22/WRKY29 transcription factors.Citation13) Although plant kinase cascades play a very important role in plant signaling, the biological functions of most protein kinases and the identities of their target proteins remain unclear.
To study protein kinase functions, strategies based on single-knock out mutations and gene silencing are widely employed but such efforts are often compromised by lethality, in the case of essential genes, or by functional redundancy and cellular homeostasis. Cell-permeable inhibitors that are highly specific for individual protein kinases would overcome the limitations of genetic approaches to enzyme inactivation, and hence such compounds represent invaluable tools for defining plant kinase functions. However, although many small-molecule inhibitors have been developed recently for animal protein kinases, their use in the study of plant kinases has been very limited.
Information on the direct target of individual protein kinases is useful in studying protein kinase functions because interaction between a kinase and its substrate is essential in order for the enzymatic reaction to take place. Many methods have been developed of investigating protein–protein interactions. Most methods that enable direct detection of these interactions, such as co-purification and affinity-precipitation assays, require stable, continuous interaction among protein molecules, but these methods are unsuitable for the identification of the substrates of individual kinases, because the interactions between kinases and their substrates are transient.
A method of analysis of protein–protein interactions has been established based on bimolecular fluorescence complementation (BiFC),Citation14,15) an alternative technique for detecting protein–protein associations in live cells.Citation15,16) In this technique, the proteins of interest are tagged with split N- and C-terminal fragments of a fluorescent protein, such as green fluorescent protein (GFP) and its derivatives. These fragments are non-fluorescent themselves, but the interactions between the tagged proteins bring the N- and C-terminal fragments into close proximity, resulting in reassembly of the fluorescent protein. Thus, reconstituted fluorescence is coupled to the interaction of the two proteins, and can be used to detect this interaction conveniently in living cells.Citation16) Because BiFC complexes are irreversible once formed,Citation15) BiFC fluorescence persists after the dissociation of the two proteins. This suggests that a screening system based on BiFC technology would be very useful in efforts to identify target (substrate) proteins of particular kinase molecules.
Here we describe a new assay method, based on BiFC technology, for the identification of the interacting molecules of plant protein kinases. As proof of principle, we produced evidence that this assay method detected OsMEK1 and its direct target, OsMAP1. In addition, screening using this assay method identified three interacting molecules of calcium-dependent protein kinase 8 (OsCPK8), a member of a kinase family that is ubiquitous in the plant kingdom, but not in animals. Using BiFC technology, we also confirmed interaction between OsCPK8 and two of the identified proteins in rice cells. These results indicate that our new assay system is useful in discriminating between kinase and its interacting protein.
Materials and methods
Plants and bacteria
Suspension cultures of rice cells (line Oc) were grown at 30 °C under light irradiation.Citation17) The cells were diluted in fresh medium every week, and all experiments were performed 4d after transfer. Acidovorax avenae strains N1141 (MAFF 301141) were grown for 1d on Pseudomonas F agar plates (Difco, Franklin Lakes, NJ) at 30 °C.Citation18,19)
Protoplast preparation and gene transformation
Rice protoplasts were isolated from cultured Oc rice cells. After 4d of culture, the cells were harvested, and the protoplasts were isolated as described previously.Citation20) The cultured rice cells were digested in an enzyme solution containing 1.0% (w/v) cellulase Onozuka RS (Yakult, Tokyo), 0.5% (w/v) macerozyme R10 (Yakult), 0.1% (w/v) pectolyase Y23 (Kyowa Chemical Products, Osaka, Japan), 0.6 m mannitol, 5 mm MES-KOH (pH 5.7), 10 mm CaCl2, and 0.1% (w/v) Bovine serum albumin (BSA) at 30 °C for 2–3 h without shaking. Protoplasts were separated from undigested cell clumps by filtration with a 100 μm cell strainer and collected by centrifugation at 100 g for 3 min at room temperature. The protoplasts were gently resuspended and washed twice in KMC solution (117 mm KCl, 82 mm MgCl2, and 85 mm CaCl2). Concentration of the protoplasts was quantified with a hemocytometer and a light microscope. Almost all the protoplasts were observed in their usual healthy and round shape. Before PEG-mediated transformation, the protoplasts were centrifuged and resuspended at 2 × 105 cells/mL in MMg solution (0.3 m mannitol, 15 mm MgCl2, and 4 mm MES-KOH, pH 5.7). The protoplast suspension (0.2 mL, 4 × 104 cells) was mixed with about 20 μL of each vector solution. An equal volume of PEG solution (40% PEG 4000 from Fluka, Buchs, Switzerland, 0.2 m mannitol, and 0.1 m CaCl2) was added and gently mixed. After incubation for 10 min at room temperature, the protoplasts were diluted with 0.8 mL of KMC solution and centrifuged at 100 g for 3 min at room temperature. After removal of the supernatant, the protoplasts were suspended in 0.2 mL KMC solution and incubated for 16 h at 30 °C before assay.
Plasmid construction
In making the Venus fusion protein, OsMEK1 (AF216315), OsMAP1 (AF216314), OsMAP2 (AF216316), OsCPK8 (AK066615), CS1 (Enolase1), CS2 (ARF-GEF), and CS3 (PPIase) were PCR amplified from a rice cDNA library with the specific primer sets. OsMEK1; (5′-CACCATGAGGGGGAAGAAGCCG-3′ and 5′-CTCGGATATATTCATTGGAG-3′), OsMAP1; (5′-CACCATGGACGGGGCGCCGGT-3′ and 5′-GTACCGGATGTTTGGGTTCA-3′), OsMAP2; (5′-CACCATGGCGATGATGGTGAGAC-3′ and 5′-CATATTCACTCCTGCAACAACCTCTGG-3′), OsCPK8; (5′-CACCATGGGATGGGCAACTCGC-3′ and 5′-GCGTGTCGTCACTGATGG-3′), CS1; (5′-CACCATGGCGGGCGACGA-3′ and 5′-GTAGGGCTCCACGGGTGC-3′), CS2; (5′-CACCATGGCTTCCCCGG-3′ and 5′-GGTACACCTCAAGGACCTCCTTA-3′), and CS3; (5′-CACCATGCCGAACCCTCAG-3′ and 5′-GAGCTCACCACAGTCGGC-3′). The resulting PCR product was cloned into pENTR-D-TOPO (Invitrogen, Carlsbad, CA). The resulting plasmids, designated OsMEK1/pENTR-D-TOPO, OsMAP1/pENTR-D-TOPO, OsMAP2/pENTR-D-TOPO, OsCPK8/pENTR-D-TOPO, CS1/pENTR-D-TOPO, CS2/pENTR-D-TOPO, and CS3/pENTR-D-TOPO. The Venus genes were amplified and cloned into pBI221 vector possessing The Gateway cassette (Invitrogen). The resulting vector was designated GW-Venus/pBI221. For the construction of Venus-fused proteins, the OsMEK1/pENTR-D-TOPO, OsMAP1/pENTR-D-TOPO, OsMAP2/pENTR-D-TOPO, OsCPK8/pENTR-D-TOPO, CS1/pENTR-D-TOPO, CS2/pENTR-D-TOPO, and CS3/pENTR-D-TOPO plasmids were converted to a gateway-compatible destination vector (GW-Venus/pBI221) by the Gateway vector conversion system.
For the interaction assay in rice protoplasts, the Vn region (1Met-154Thr) and the Vc region (155Ala-239Lys) were amplified with the following primer pairs: Vn, 5′-ATGGTGAGCAAGGGCGAGGA-3′ and 5′-GGTGATATAGACGTTGTGGC-3′; and Vc, 5′-GCCGACAAGCAGAAGAACGG-3′ and 5′-TTACTTGTACAGCTCGTCCA-3′. They were cloned into the pBI221 vector possessing the Gateway cassette. The resulting vectors were designated GW-Vn/pBI221 and GW-Vc/pBI221, respectively. For the construction of Vn-fused proteins, the OsMEK1/pENTR-D-TOPO, CS1/pENTR-D-TOPO, CS2/pENTR-D-TOPO, and CS3/pENTR-D-TOPO plasmids were converted to a gateway-compatible destination vector (GW-Vn/pBI221) with the Gateway vector conversion system. For the construction of the Vc-fused proteins, the OsMAP1/pENTR-D-TOPO, OsMAP2/pENTR-D-TOPO, and OsCPK8/pENTR-D-TOPO plasmids were converted to a gateway-compatible destination vector (GW-Vc/pBI221).
For expression in Escherichia coli, a trimethoprim resistance gene fragment was PCR-amplified from pBBR1Tp with a specific primer set (5′-TCAACGGGAAACGTCTTGCTCTA-3′ and 5′-TTTCAGAAACAACTCTGGCGCAT-3′). The amplified trimethoprim resistance gene was introduced into the NruI site of pET28b (Merck, Darmstadt, Germany) with an In-Fusion HD Cloning Kit (Clontech, Mountain View, CA). The resulting plasmid was designated pETTP. For the expression of Vn-fused protein, OsMEK1-Vn and Venus were PCR-amplified with each of the specific primer sets, (OsMEK1-Vn, 5′-CATGCCATGGCTATGAGGGGGAAGAAGCCG-3′ and 5′-TAAAGCGGCCGCAAATCAGGTGATATAGACGTT-3′; and Venus, 5′-CCATGGCAATGGTGAGCAAGGGCGAGGA-3′ and 5′-CTCGAGGTACAGCTCGTCCATGCCCA-3′). The amplified fragments were digested with NcoI and NotI and cloned into pETTp. The resulting plasmids were designated pETTp-OsMEK1-Vn and pETTp-Venus.
For the expression of the Vc-fused protein in E. coli, the Vc region DNA was amplified by PCR with a specific primer set (5′-CCATGGCAGCCGACAAGCAGAAGAACGG-3′ and 5′-CTCGAGGTACAGCTCGTCCATGCCCA-3′). Amplified fragments were digested with NcoI and XhoI and cloned into pCDF-Duet (Novagen), and the resulting plasmid was designated NcoI-pCDF-Vc. To change the NcoI site to EcoRV site in NcoI-pCDF-Vc, a KOD-Plus-mutagenesis kit (Toyobo, Osaka, Japan) with specific primers (5′-TGAGCAATAACTAGCATAACCCCTT-3′, and 5′-GTCAGTCAGTCACTTGTACAGCTCGTCCATGCCGAGA-3′) was used. The resulting plasmid was designated pCDF-Vc. OsMAP1, OsMAP2, and OsMAP3 were amplified from the rice cDNA library with specific primer sets. GUS (the β-glucuronidase encoding gene) was also amplified from pBI221 with specific primers. The gene-specific primers were as follows: OsMAP1, 5′-CACCATGGAGGGGGCGCCGGT-3′ and 5′-GTACCGGATGTTTGGGTTCA-3′; OsMAP2, 5′-GATATCATG-GCGATGATGGTGGAC-3′ and 5′-GATATCCATATTCACTCCTGCAACAACAACC-3′; OsMAP3, 5′-GATATCATGGCGATCATGGTGGATC-3′ and 5′-GATATCTCGGGCACTCATTGCTG-3′; and GUS, 5′-TTTAAAATGTTACGCCTGTAGAAACCCC-3′ and 5′-TTTAAATTGTTTGCCTCCCTGCTG-3′. The DNA fragments were inserted into EcoRV site of pCDF-Vc.
To construct a cDNA library, three reading frame vectors, designated, pCDF-T7A-Vc, pCDF-T7B-Vc, and pCDF-T7C-Vc, were prepared from pCDF-Vc with three forward primers (A, 5′-GATATCGCCGACAAGCAGAAGAACGGCATCA-3′; B, 5′-GATATCCAGCCGACAAGCAGAAGAACGGCAT-3′; and C, 5′-GATATCAGCCGACAAGCAGAAGAACGGCATC-3′). The common reverse primer was 5′-CATGGTATATCTCCTTATTAAAGTTAAACA-3′. Moreover, two reading frame vectors were prepared from each of pCDF-T7A-Vc, pCDF-T7B-Vc, and pCDF-T7C-Vc with two reverse primers (1, 5′-GCCATGGTATATCTCCTTATTAAAGTT-3′; and 2, 5′-GCATGGTATATCTCCTTATTAAAGTT-3′). The common forward primer was 5′-GATATCGCCGACAAGCAGAAGAACGGCATCA-3′. The resulting plasmids were designated pCDF-T7A-Vc1, pCDF-T7A-Vc2, pCDF-T7B-Vc1, pCDF-T7B-Vc2, pCDF-T7C-Vc1, and pCDF-T7C-Vc2. The six plasmids were mixed and designated pCDF-Vc-mix.
Construction of the cDNA library
Cultured rice cells were inoculated with A. avenae incompatible N1141 strain (108 cfu mL−1) at 30 °C. Total RNA was isolated from the cells with Isogen (Nippon gene, Tokyo). Poly (A)+ RNA was purified with a Oligotex-dT30 mRNA Purification Kit (Takara Bio, Shiga, Japan). First-strand cDNA was synthesized by the Super Script III First-Strand Synthesis System (Invitrogen) following the manufacturer′s instructions. cDNAs shorter than 400 bp were removed with a Chroma Spin column (Takara). pCDF-Vc-mix (six reading frame vectors) were digested with EcoRV and then dephosphorylated with Alkaline Phosphatase (Takara). Synthesized cDNAs were cloned into the digested pCDF-Vc vector. The resulting plasmids were electrotransformed into NEB 10-beta Electrocompetent E. coli (New England BioLabs, Ipswich, MA).
Screening system for the identification of OsCPK8 interacting protein
E. coli Rosetta-gami B (Merck) was transformed with pETTp-OsCPK8-Vn and pCDF-OscDNAs-Vc, and then cultured at 37 °C on a Magna Nylon Transfer Membrane (GE Osmonics, Minnetonka, MN) set on LB plates containing kanamycin (50 μg/mL) and spectinomycin (50 μg/mL). After 16 h, 0.1 mm IPTG was added to the LB plates and the transformants were cultured at 25 °C for 6 h. BiFC fluorescence was detected by FLA-3000 (Fujifilm, Tokyo) or stereoscopic fluorescence microscope (MZFLIII, Leica, Wetzlar, Germany). cDNA inserts of identified clones were amplified by PCR through 30 cycles with two primers, T7, 5′-TCATTAGGCACCCCAGGCTTTACAC-3′, and Vc, 5′-GTAATACGACTCACTATAGGGC-3′. The amplified products were sequenced with the same sets of primers with a DNA sequencer (ABI Prism 3130 Genetic Analyzer, applied biosystems, Carlsbad, CA).
Immunoblotting analysis with an anti-GFP antibody
Each bacterial strain was resuspended with distilled water at 0.75 of OD610, and then supplemented with the same volume of 2 × SDS-PAGE sample buffer (125 mm Tris-HCl, pH 6.8, 4% SDS, β-mercaptoethanol, 20% glycerol, and 0.01% BPB). After centrifugation, samples were separated by means of a SDS-polyacrylamide gel (12.5%). The separated proteins were transferred electrophoretically to a nitrocellulose membrane (Millipore, Bedford, MA) with a Bio-Rad semidry blotter (Bio-Rad, Hercules, CA). Nonspecific binding was blocked with 3% skimmed milk in TBST buffer (20 mm Tris-HCl, pH 8.0, 150 mm NaCl, 0.05% Tween 20) for 1 h at room temperature. The membrane was incubated with polyclonal anti-GFP antibody (Medical and Biological Laboratories, Nagoya, Japan) diluted with TBST overnight at 4 °C. After washing with TBST, the membrane was incubated with a horseradish peroxidase-conjugated rabbit IgG antibody (Medical and Biological Laboratories) diluted with TBST for 1 h at room temperature. Visualization was done with an enhanced immunochemiluminescence kit (ECL-prime, GE Healthcare, Pittsburgh, PA), and chemiluminescence was detected by LAS-4000 (GE Healthcare). DynaMarker Protein Multicolor III (BioDynamics, Tokyo) was used to calculate the sizes of the proteins.
Quantitative real-time RT-PCR
Total RNA was isolated from cultured rice cells with an RNeasy Plant Mini kit (Qiagen, Hilden, Germany) with DNase digestion following the manufacturer’s instructions. Quantitative real-time RT-PCR was performed on an Opticon2 instrument (Bio-Rad) using a QuantiTect SYBR Green RT-PCR kit (Qiagen) with OsCPK8 gene-specific primers, 5′-CACGCAATAGACTGGACAGAGA-3′ and 5′-ATCGCTGCTGGTAAGCACAA-3′. OsCPK8 mRNA levels were calculated with a calibration curve prepared with standard OsCPK8 genes of known template amounts (1 ng–0.1 pg) and normalized based on reference gene Act-1.
Reporter assay
The OsCPK8 vector was co-transfected with OsWRKY70::LUC (firefly luciferase) and 35S::RLUC (Renilla luciferase) into the rice protoplasts. The protoplasts were incubated for 12–14 h at 30 °C. Protein was then isolated, and LUC activity was recorded by following the Dual-Luciferase Reporter system (Promega, Madison, WI) following the manufacturer’s instructions.
Results
Detection of the interaction between a kinase and its substrate in rice
We chose BiFC as our method of detecting the interaction between a given kinase and its substrate. This technique was initially developed to detect interactions of transcription factors in animal cells,Citation8) but it can effectively detect interactions of specific proteins in plant cells.Citation16,21,22) BiFC is a peptide complementation assay in which non-fluorescent fragments of a fluorescent protein, such as GFP or one of its derivatives, are fused in-frame with test proteins. Interactions between the test proteins bring the GFP fragments into proximity in such a way that they refold and restore fluorescence.Citation8)
OsMEK1 interacts with OsMAP1, but not with OsMAP2 or OsMAP3. We selected one pair of interacting proteins, OsMEK1 and OsMAP1, and one pair of non-interacting proteins, OsMEK1 and OsMAP2, to evaluate our BiFC assay system. As split fluorescent protein for the BiFC assay, we chose Venus protein, a derivative of yellow fluorescent protein (YFP). To determine whether OsMEK1 interacts with specifically OsMAP1 but not OsMAP2, the cDNA encoding OsMEK1 was fused with the N-terminal fragment of Venus (OsMEK1-Vn), and the cDNAs encoding OsMAP1 and OsMAP2 were fused with the C-terminal fragment of Venus (OsMAP1-Vc and OsMAP2-Vc). Both the OsMEK1-Vn and the OsMAPs-Vc gene were under the control of the cauliflower mosaic virus 35S promoter. We introduced each of the vectors alone or in combination (OsMEK1-Vn and either OsMAP1-Vc or OsMAP2-Vc) into rice protoplasts, by the PEG method,Citation20) and observed Venus-derived fluorescence by confocal laser microscopy. When these vectors were co-introduced into rice protoplasts along with a DsRed expression vector (for the identification of transformed cells), no Venus-derived fluorescence was observed in the transformed protoplasts (DsRed-positive cells) (Fig. ). The protoplasts transformed with OsMEK1-Vn and OsMAP1-Vc (DsRed-positive cells) exhibited Venus-derived fluorescence in the cytoplasm, whereas no fluorescence was observed in the protoplasts transformed with OsMEK1-Vn and OsMAP2-Vc (Fig. ). These data suggest that OsMEK1 interacts with OsMAP1 but not OsMAP2 in rice cells. Furthermore, the data clearly indicated that a BiFC system based on Venus protein could detect specific interactions such as those between enzymes and substrates.
Fig. 1. BiFC analysis of interaction between OsMEK1 and OsMAP1 or OsMAP2 in rice protoplasts.
Note: (I) BiFC; (II) DsRed; (III) Merged image of BiFC, DsRed, and bright field. OsMEK1 was fused with the N-terminal fragment of Venus (OsMEK1-Vn). OsMAP1 and OsMAP2 were fused with the C-terminal fragment of Venus (OsMAP1-Vc and OsMAP2-Vc). OsMAP1-Vc, OsMAP2-Vc, and OsMEK1-Vn: each construct was individually co-transformed into rice protoplasts with DsRed expression vector. OsMEK1-Vn/OsMAP1-Vc: OsMEK1-Vn and OsMAP1-Vc were co-transformed into protoplasts with DsRed expression vector. OsMEK1-Vn/OsMAP2-Vc: OsMEK1-Vn and OsMAP2-Vc were co-transformed into protoplasts with DsRed expression vector. The transformed protoplasts were observed 12 h after the introduction of the vectors. Bar, 10 μm.
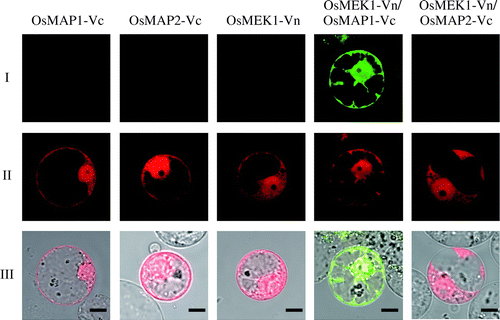
Establishment of a screening system for the detection of kinase-substrate interactions in E. coli
For high-throughput screening of kinase-interacting proteins, a convenient and effective assay system is necessary. Due to easy handling and transformation, E. coli is widely used in experiments in molecular biology, including the production of proteins and the detection of protein–protein interactions. Hence, we attempted to establish a convenient and simple high-throughput screening system for kinase-substrate interactions by the BiFC technique in E. coli.
To establish the BiFC screening system, cDNA encoding OsMEK1 was fused with the N-terminal fragment of Venus and placed downstream of the T7 promoter (pETTp-OsMEK1-Vn). OsMAP1, OsMAP2, OsMAP3, and uidA (which encodes a β-glucuronidase, GUS) were also fused with the N-terminal fragment of Venus and placed under the control of the T7 promoter of pCDF-Duet. These vectors were designated pCDF-OsMAP1-Vc, pCDF-OsMAP2-Vc, pCDF-OsMAP3-Vc, and pCDF-GUS-Vc, respectively (Fig. ).
Fig. 2. Vectors used in the establishment of the screening system utilizing E. coli Rosetta-gami B.
Note: (A) Venus expression vector. pETTp-Venus contains the Venus gene placed between the T7 promoter and the T7 terminator. (B) OsMEK1-Vn expression vector. pETTp-OsMEK1-Vn contains OsMEK1 fused to the N-terminal fragment of the Venus gene (Vn) placed between the T7 promoter and the T7 terminator. (C) OsMAPs-Vc and GUS-Vc expression vectors. pCDF-X-Vc contains OsMAP1, OsMAP2, or OsMAP3 fused to the C-terminal fragment of Venus (Vc) placed between the T7 promoter and the T7 terminator. pCDF-GUS-Vc encodes GUS fused to the C-terminal fragment of Venus (Vc) placed between the T7 promoter and the T7 terminator. TmpR, trimethoprim resistance gene; SmR, streptomycin/spectinomycin resistance gene; GUS, β-glucuronidase.
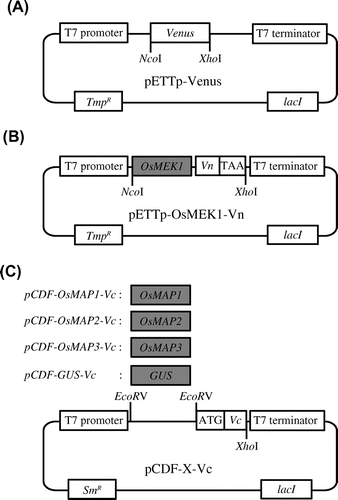
We chose the Rosetta-gami B strain of E. coli for the BiFC screening system because it combines the key features of Origami and Rosetta, enhancing both the expression of eukaryotic proteins and the formation of target-protein disulfide bonds in the bacterial cytoplasm. First, we tested whether to determine Rosetta-gami B cells would produce the mature Venus protein at the original fluorescence wavelength. Rosetta-gami B bacteria expressing Venus genes (pETTp-Venus) cultured on LB plates with IPTG for 6 h became brightly fluorescent at an emission wavelength of 530 nm (Fig. (A)), suggesting that the Venus protein produced by this strain emits at the original fluorescence wavelength. Next, we attempted to detect protein–protein interactions between OsMEK1 and OsMAPs in Rosetta-gami B cells. pETTp-OsMEK1-Vn was co-introduced into this strain along with pCDF-OsMAP1-Vc, pCDF-OsMAP2-Vc, pCDF-OsMAP3-Vc, or pCDF-GUS-Vc, and the transformants were cultured on nylon membranes placed on a LB plate with IPTG. After 6 h, bright fluorescence derived from refolded Venus was observed in the cells co-expressing OsMEK1 and OsMAP1. In contrast, no fluorescence was observed in cells co-expressing OsMEK1 and OsMAP2, OsMAP3, or GUS (Fig. (A)). Immunoblot analysis by anti-GFP antibody revealed that all the proteins encoded by the transformed vectors were expressed at similar levels in the corresponding transformants (Fig. (B)). Together, the data suggest that this E. coli screening system based on the BiFC technique can efficiently detect specific kinase-substrate interactions.
Fig. 3. Detection of specific interaction by BiFC in E. coli strain Rosetta-gami B.
Note: (A) Detection of fluorescence derived from BiFC of a culture plate. The cells contained the following vectors: 1, pETTp-Venus; 2, pETTp-Vn and pCDF-OsMAP1-Vc; 3, pETTp-OsMEK1-Vn and pCDF-OsMAP1-Vc; 4, pETTp-OsMEK1-Vn and pCDF-OsMAP2-Vc; 5, pETTp-OsMEK1-Vn and pCDF-OsMAP3-Vc; and 6, pETTp-OsMEK1-Vn and pCDF-GUS-Vc. Transformed E. coli Rosetta-gami B were cultured on nylon membranes left LB plates for 16 h at 37 °C, and then cultured on LB plates with 12.5 mm IPTG for 6 h at 25 °C. BiFC fluorescence was detected by means of an FLA3000 at an emission wavelength of 530 nm. (B) Immunoblot analysis of accumulated proteins in transformed cells by means of the anti-GFP antibody. The cells contained the following vectors: 1, pETTp-Venus; 2, pETTp-Vn and pCDF-OsMAP1-Vc; 3, pETTp-OsMEK1-Vn and pCDF-OsMAP1-Vc; 4, pETTp-OsMEK1-Vn and pCDF-OsMAP2-Vc; 5, pETTp-OsMEK1-Vn and pCDF-OsMAP3-Vc; and 6, pETTp-OsMEK1-Vn and pCDF-GUS-Vc. The same amount of each of the fractions was separated by SDS-PAGE, and proteins were detected by silver staining (below). Non-specific bands are indicated by asterisks.
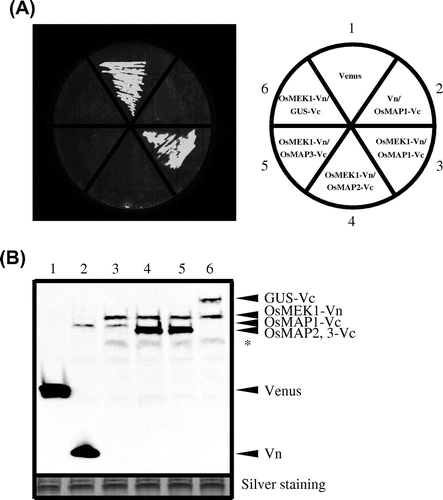
Plant immune induction mediated by calcium-dependent protein kinase 8
Recently, we reported that a rice-avirulent N1141 strain of A. avenae caused several immune responses, including hypersensitive cell death, H2O2 generation, and elevated expression of immunity-related genes, whereas the rice-virulent K1 strain of A. avenae does not induce these immune responses.Citation23–26) To elucidate the molecular mechanism of immune induction in rice, we analyzed gene-expression patterns by means of cDNA microarrays containing 3,353 independent rice cDNA clones. Statistical analysis and quantitative real-time RT-PCR revealed that 136 genes were upregulated during the induction of the immune responses. Analysis of sequence homology revealed that the ubiquitin-conjugating enzyme, the calcium-dependent protein kinase, the MAP kinase, the heat shock protein, the LRR-kinase, the Ca2+-binding protein, EDS1, and senescence-associated proteins were included among the upregulated genes.Citation27)
Calcium-dependent protein kinases (CPKs) play important roles in plant immune induction. They have a characteristic structure comprising an N-terminal variable domain, a serine/threonine kinase domain, a junction containing an auto-inhibitory domain, and a C-terminal calmodulin domain.Citation28) CPK activities are regulated not only by calcium, but also by specific phospholipids and autophosphorylation.Citation29Citation–Citation31) Some CPK genes are ubiquitously expressed, whereas others are regulated during immune responses.Citation30,32) Hence, we attempted to identify the CPK genes, that were induced during immune responses induced by the avirulent N1141 strain of A. avenae. Quantitative real-time RT-PCR analysis revealed that the OsCPK8 transcript was induced 3 h after inoculation with the N1141 strain, and that its expression levels gradually increased up to 6 h after inoculation. In the K1-inoculated cultured rice cells, the OsCPK8 mRNA levels were lower than those in the N1141-inoculated cells (Fig. (A)).
Fig. 4. Immune responses induced by OsCPK8 in rice.
Note: (A) Time-course of OsCPK8 expression in cultured rice cells following inoculation with the avirulent N1141 strain (hollow circle) and with the virulent K1 strain (solid circle) of A. avenae. The amounts of OsCPK8 mRNA were measured by real-time RT-PCR. Each data point represents the average of three independent experiments. Bars indicate standard deviation. (B) Enhancement of OsWRKY expression activity by transient overexpression of OsCPK8. Protoplasts isolated from cultured rice cells were transformed with OsWRKY70:: Luc, RLuc, and either empty vector or OsCPK8/pHAC17. OsWRKY promoter activities are represented by the ratio between firefly luciferase and Renilla luciferase activities, normalized against the value obtained for mock treatment. Asterisks indicate a significant increase (t-test; p < 0.05).
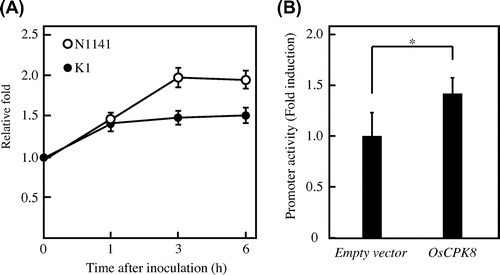
Next, we attempted to determine whether an accumulation of OsCPK8 mRNA is involved in the induction of the plant immune response, by a previously developed transient expression assay system based on immune-responsive rice gene OsWRKY70.Citation20) The OsCPK8 expression vector was co-transformed into rice protoplasts along with an OsWRKY70 promoter-driven luciferase reporter, and the luciferase activities in the transformed rice protoplasts were measured by the reporter assay. The luciferase activity in the rice protoplasts transformed with OsCPK8 expression vector and the OsWRKY70 promoter-driven luciferase vector was significantly stronger than that in the rice protoplasts transformed with the OsWRKY70 reporter vector and an empty-vector control (Fig. (B)). These results indicate that the accumulation of OsCPK8 causes an immune response in rice.
Screening of OsCPK8 interacting proteins by the BiFC assay system in E. coli
To validate the BiFC screening system in E. coli Rosetta-gami B, we used it to identify OsCPK8-interacting proteins. To this end, first we constructed a cDNA library for BiFC screening. Total RNA was isolated from cultured rice cells infected with the avirulent N1141 strain of A. avenae. After purification of poly (A) RNA, reverse transcription into cDNA was performed with random primers, and cDNAs shorter than 500 bp were removed. A rice cDNA expression library was constructed by ligation of the purified cDNAs into pCDF-Vc-mix vectors with six reading frames (pCDF-OscDNAs-Vc) (Fig. (A)). To construct the bait vector, cDNA encoding OsCPK8 was fused with the N-terminal fragment of Venus and placed downstream of the T7 promoter of pETTp-28b (pETTp-OsCPK8-Vn) (Fig. (B)).
Fig. 5. BiFC screening for the detection of OsCPK8-interacting protein.
Note: (A) Vectors used in the rice cDNA-Vc library. pCDF-Vc-mix vectors contain six reading frames, and cDNAs were inserted into the EcoRV site. (B) OsCPK8-Vn expression vector. pETTp-OsCPK8-Vn contains OsCPK8 fused to the N-terminal fragment of Venus (Vn) placed between the T7 promoter and the T7 terminator. TmpR, trimethoprim resistance gene; SmR, streptomycin/spectinomycin resistance gene. C, Retest of specific interaction between OsCPK8 and CS1, CS2, and CS3. pETTp-OsCPK8-Vn was co-introduced into E. coli Rosetta-gami B along with pCDF-CS1-Vc, pCDF-CS2-Vc, pCDF-CS3-Vc, or pCDF-Vc, and the transformants were streaked on nylon membranes placed on LB plates with IPTG. After 6 h, bright fluorescence, derived from BiFC, was analyzed by illumination under a stereo fluorescence microscope.
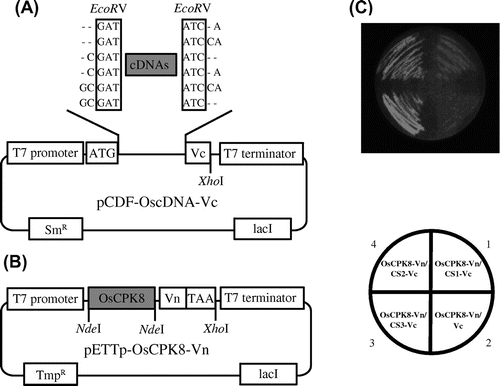
Rosetta-gami B E. coli were co-transformed with pETTp-OsCPK8-Vn and the cDNA expression library in pCDF-OscDNAs-Vc, and then cultured at 37 °C on nylon membranes placed on LB plates without IPTG. Transformant colonies formed and the nylon membrane were removed. IPTG was added to the plate and the nylon membrane was returned to the plate and heated to 25 °C. After 6 h of cultivation, approximately 80,000 colonies were analyzed by illumination under a stereo fluorescence microscope. Since 34 colonies were isolated, colony PCR was carried out for the fluorescence colonies to confirm the insertion of the cDNA. Among the analyzed colonies, the 20 colonies possessed in-frame protein-encoding genes. Three abundant genes from 20 colonies were designated, CS1, CS2, and CS3.
DNA sequencing analysis revealed that CS1, CS2, and CS3 were 413, 336, and 1,017 bp in length, respectively. A homology search of these genes against the GenBank/EMBL database was done by the FASTA program. It revealed that CS1, CS2, and CS3 encode enolase 1 (AK099292), a guanine-nucleotide exchange factor for ADP-ribosylation factor GTPase (ARF-GEF) (NM00156080.1), and peptidyl prolyl isomerase (AK103172.1), respectively. To confirm interaction between OsCPK8 and CS1, CS2, and CS3, pETTp-OsCPK8-Vn was co-introduced into E. coli Rosetta-gami B along with pCDF-CS1-Vc, pCDF-CS2-Vc, pCDF-CS3-Vc, or pCDF-Vc, and the transformants were streaked on nylon membranes placed on a LB plate with IPTG. After 6 h, bright fluorescence derived from refolded Venus was observed in the cells co-expressing OsCPK8 and CS1, CS2, and CS3 (Fig. (C)).
Detection of BiFC signals by OsCPK8 and the identified proteins
To characterize the specific interaction between OsCPK8 and the identified proteins (CS1, CS2, and CS3) in rice cells, first we were analyzed the localization of each protein. Full-length cDNAs encoding OsCPK8, CS1, CS2, and CS3 were fused with Venus and expressed under the control of the cauliflower mosaic virus 35S promoter. These plasmids were introduced by the PEG method into protoplasts derived from cultured rice cells, and transient expression was observed by confocal laser fluorescence microscopy. When the OsCPK8-Venus construct was observed into the rice protoplasts, green fluorescence was seen on the plasma membrane (Fig. (A)). In contrast, green fluorescence in the nucleus was observed in the rice protoplasts transfected with CS1-Venus (Fig. (A)). When CS2-Venus and CS3-Venus were introduced into rice protoplasts, fluorescence derived from Venus was observed in both the cytoplasm and the nucleus.
Fig. 6. BiFC analysis of the interaction between OsCPK8 and CS1, CS2, or CS3 in rice protoplasts.
Note: (A) Localization of the identified proteins in rice protoplasts. Venus-fused identified proteins (CS1-Venus, CS2-Venus, and CS3-Venus) were expressed in rice protoplasts. Upper panels are Venus images, and lower panels are merges of Venus and bright-field images. (B) Interaction between OsCPK8 and the identified proteins detected by BiFC in rice protoplasts. Proteins fused to the N-terminal fragment of Venus (Vn) (CS1-Vn, CS2-Vn, and CS3-Vn) and the C-terminal fragment of Venus (Vc) (OsCPK8-Vc) were co-expressed in rice protoplasts. Reconstructed Venus fluorescence and bright field were obtained by fluorescence confocal microscopy. Bar, 10 μm. BF, bright field.
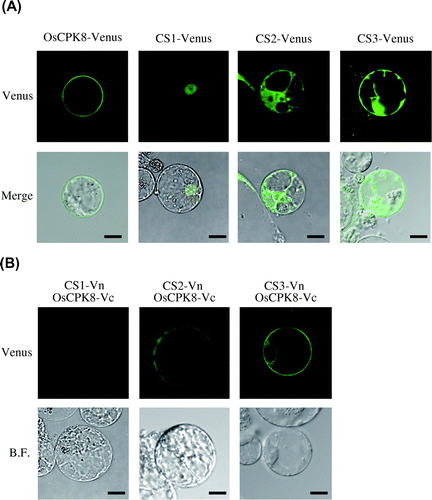
Next, we investigated whether OsCPK8 would interact with the three identified proteins in rice cells. cDNA encoding OsCPK8 was fused with the C-terminal fragment of Venus (OsCPK8-Vc), and the full-length cDNAs of CS1, CS2, and CS3 were fused with the N-terminal fragment of Venus (CS1-Vn, CS2-Vn, and CS3-Vn). All of the fused genes were placed under the control of the cauliflower mosaic virus 35S promoter. When OsCPK8-Vc was co-introduced with CS2-Vn or CS3-Vn, BiFC signals were observed on the plasma membrane. In contrast, in the protoplasts co-transformed with OsCPK8-Vc and CS1-Vn, no fluorescence signal was detected (Fig. (B)). These results indicate that OsCPK8 specifically interacts with CS2 and CS3, but not with CS1, on the plasma membrane of rice cells.
Discussion
Here, we describe a novel screening system in E. coli, based on the BiFC technique, for detecting specific interactions. Using this system, we identified proteins that interact with OsCPK8. Several high-throughput detection systems for protein–protein interactions have been developed, of which the most widely used is yeast two-hybrid screening. In that method, the GAL4 transcription factor is split into two separate fragments, a binding domain (BD) and an activating domain (AD). The BD fragment is fused with a bait protein, and the AD fragment is fused with a prey protein. If the bait interacts with the prey, then AD and BD are juxtaposed, bringing AD in proximity to the transcription start site and activating transcription of the reporter gene. Hence, successful detection of protein–protein interactions in the two-hybrid system requires continuous interaction between the bait and prey proteins in the yeast nucleus. In contrast, our novel screening system, which uses BiFC in E. coli, is based on protein fragment complementation. The proteins of interest are tagged with split N- and C-terminal fragments of a fluorescent protein such as YFP or Venus. Although these split fragments are themselves non-fluorescent, interaction between the tagged proteins brings the fragments together and promotes their refolding into a β-barrel structure. The formation of the fluorescent protein β-barrel allows a slower oxidation reaction to generate the internal chromophore, resulting in simple single-wavelength fluorescence derived from the complemented fluorescence protein as an indicator of protein–protein interaction. The first fragment-refolding step commits a particular interaction to form a BiFC complex, and this process is fast (a half-time of 60 s in vitro). In addition, BiFC complexes also appear irreversible once formed. Hence, a detection system based on the BiFC technique can detect transient interactions between a kinase and its substrate.Citation14)
When the yeast-two hybrid system is used to detect protein interactions, a difficult problem occurs as to auto-activation of reporter genes by the bait protein. If the bait protein is a transcriptional activation factor, often a reporter gene is activated only in the bait protein. In contrast, our screening system based on BiFC functions effectively even if the bate protein is a transcription activation factor. Hence, our system based on the BiFC technique should function even when the bait protein shows auto-activation.
In E. coli and rice protoplasts, the BiFC assay with OsCPK8 as bait revealed that OsCPK8 interacts specifically with a guanine-nucleotide exchange factor for ADP-ribosylation factor GTPase (ARF-GEF) and peptidyl prolyl isomerase. ARF-GEFs regulate membrane trafficking and the actin cytoskeleton at the plasma membrane in all eukaryotic kingdoms, and facilitate the exchange of bound GDP for GTP by ARF GTPase. ARF-GEFs are currently classified into eight subfamilies of eukaryotes, but only the ancestral large Golgi-specific Brefeldin A resistance factor (GBF) type is found in plants.Citation33,34) The large ARF-GTP exchange factor (ARF-GEF) GNOM is vital to the accumulation of callose and mediates the transport required for plant innate immunity.Citation35) The gnom mutant exhibits a delay in papilla formation caused by penetration of powdery mildew fungi, and this delay is abolished upon functional complementation of the mutant, suggesting that ARF-GEF is involved in plant disease resistance. In addition, the data also indicate the possibility that OsCPK8 regulates the function of ARF-GEF via phosphorylation resulting from the specific interaction.
Guanine nucleotide exchange factors also regulate OsRac1-mediated plant immune responses.Citation36) Rice SWAP70A and SWAP70B, homologs of human GEFs, interact with rice Rho GTPase OsRac1, an important signaling factor in immune responses. Transient expression of OsSWAP70A in plant cells enhances the OsRac1-mediated production of reactive oxygen species. Reductions in OsSWAP70A and OsSWAP70B mRNA levels by RNA interference result in the suppression of chitin elicitor-induced defense gene expression and ROS production. Thus, it is likely that ARF-GEF directly regulates the induction of immune responses in plants. Although there is as yet no evidence that ARF-GEF is phosphorylated, several immune responses of rice mediated by ARF-GEF might be controlled by phosphorylation resulting from direct interaction with OsCPK8. Therefore, in order to elucidate the mechanism of OsCPK8-mediated immune induction, it is important to detect direct phosphorylation of ARF-GEF by OsCPK8.
Another candidate substrate of OsCPK8 is peptidyl prolyl isomerase (PPIase). Our sequence analysis revealed that the identified PPIase is a member of FK506-binding proteins (FKBP). These proteins are receptors for immunosuppressant drugs as well as prolyl isomerases. FKBPs were initially characterized as target molecules of FK506 and rapamycin, soil-bacterium macrolides that are used as immunosuppressant drugs.Citation37) The FKBPs joined the unrelated cyclosporin-binding cyclophilin (CYP) proteins in the class of immunosuppressant receptors known as immunophilins. In animal cells, the FK506–FKBP and cyclosporin–CYP complexes interact with calcium-dependent phosphatase, interrupting the phosphorylation signal pathway required for expression of the genes involved in the immune response, FKBP-bound rapamycin interacts with a kinase to cause a cell-cycle arrest that is also immunosuppressive.Citation38) Another characteristic of FKBPs is peptidyl-prolyl isomerase activity, which catalyzes the rotation of the peptide bond immediately preceding a proline residue.
FKBPs form large protein families in eukaryotes, including higher plants, with 23 family members in Arabidopsis thaliana and 29 family members in rice.Citation37,38) Previous functional and structural investigations have implicated plant FKBPs in diverse cellular processes, including development, stress responses, transcription regulation, and chloroplast function.Citation39) Furthermore, a cytosolic FKBP in Arabidopsis functions as a co-chaperone of HSP90 and interacts with freshly synthesized pre-proteins.Citation39) In addition, we have reported that overexpression of OsNAC4, which encodes a plant-specific transcription factor, activates the hypersensitive response (HR), which involves cell death accompanied by a loss of plasma membrane integrity, nuclear DNA fragmentation, and characteristic morphological changes. A microarray analysis with OsNAC4-knockdown lines indicated that OsHSP90 expression levels differed between the OsNAC4-knockdown and control lines during HR cell death. Furthermore, transient expression of OsHSP90 clearly induced HR cell death, which was accompanied by a loss of plasma membrane integrity.Citation40) These data suggest the attractive possibility that plant immune responses are caused by a complex of OsHSP90 and PPIase, and that the formation of this complex is regulated by the phosphorylation of PPIase mediated by OsCPK8. This is consistent with the findings, described above, that the phosphorylation signal is interrupted by a complex of FKBP and the calcium-dependent phosphatase.
Although our novel screening system in E. coli, based on the BiFC technique, detected direct kinase-substrate interactions such as OsMAP1 and OsMEK1, it is not clear whether interaction between OsCPK8 and CS2 or CS3 derives from kinase-substrate interaction. To clarify this, it is important to detect direct phosphorylation of CS2 and CS3 by OsCPK8, if it occurs.
Acknowledgments
We are grateful to Ms. Hiromi Morii for excellent technical support. This work was supported in part by a Grant-in-Aid for Scientific Research (B) (25292067) from the Ministry of Education, Culture, Sports, Science, and Technology of Japan, and by the Program for the Promotion of Basic and Applied Research for Innovation in Bio-Oriented Industry.
References
- Nakagami H, Pitzschke A, Hirt H. Trends Plant Sci. 2005;10:339–346.10.1016/j.tplants.2005.05.009
- Manning G, Whyte DB, Martinez R, Hunter T, Sudarsanam S. Science. 2002;298:1912–1934.10.1126/science.1075762
- Dardick C, Chen J, Richter T, Ouyang S, Ronald P. Plant Physiol. 2007;143:579–586.
- Pitzschke A, Schikora A, Hirt H. Curr. Opin. Plant Biol. 2009;12:421–426.10.1016/j.pbi.2009.06.008
- Tena G, Asai T, Chiu WL, Sheen J. Curr. Opin. Plant Biol. 2001;4:392–400.10.1016/S1369-5266(00)00191-6
- Zhang S, Klessig DF. Trends Plant Sci. 2001;6:520–527.10.1016/S1360-1385(01)02103-3
- Jonak C, Okrész L, Bögre L, Hirt H. Curr. Opin. Plant Biol. 2002;5:415–424.10.1016/S1369-5266(02)00285-6
- Pitzschke A, Hirt H. Plant Physiol. 2006;141:351–356.10.1104/pp.106.079160
- Mittler R. Trends Plant Sci. 2002;7:405–410.10.1016/S1360-1385(02)02312-9
- Mittler R, Vanderauwera S, Gollery M, Van Breusegem F. Trends Plant Sci. 2004;9:490–498.10.1016/j.tplants.2004.08.009
- Nakagami H, Soukupova H, Schikora A, Zarsky V, Hirt H. J. Biol. Chem. 2006;281:38697–38704.10.1074/jbc.M605293200
- Wen JQ, Oono K, Imai R. Plant Physiol. 2002;129:1880–1891.10.1104/pp.006072
- Asai T, Tena G, Plotnikova J, Willmann MR, Chiu WL, Gomez-Gomez L, Boller T, Ausubel FM, Sheen J. Nature. 2002;415:977–983.10.1038/415977a
- Hu CD, Chinenov Y, Kerppola TK. Mol. Cell. 2002;9:789–798.10.1016/S1097-2765(02)00496-3
- Kerppola TK. Methods Cell Biol. 2008;85:431–470.10.1016/S0091-679X(08)85019-4
- Ohad N, Shichrur K, Yalovsky S. Plant Physiol. 2007;145:1090–1099.10.1104/pp.107.107284
- Baba A, Hasezawa S, Syono K. Plant Cell Physiol. 1986;27:463–472.
- Kadota I, Ohuchi A, Nishiyama K. Ann. Phytopathol. Soc. Jpn. 1991;57:268–273.10.3186/jjphytopath.57.268
- Che FS, Nakajima Y, Tanaka N, Iwano M, Yoshida T, Takayama S, Kadota I, Isogai A. J. Biol. Chem. 2000;275:32347–32356.10.1074/jbc.M004796200
- Takai R, Kaneda T, Isogai A, Takayama S, Che FS. Biosci. Biotechnol. Biochem. 2007;71:590–593.10.1271/bbb.60526
- Weinthal D, Tzfira T. Trends Plant Sci. 2009;14:59–63.10.1016/j.tplants.2008.11.002
- Lee LY, Wu FH, Hsu CT, Shen SC, Yeh HY, Liao DC, Fang MJ, Liu NT, Yen YC, Dokládal L, Sykorová E, Gelvin SB, Lin CS. Plant Cell. 2012;24:1746–1759.10.1105/tpc.112.097998
- Che FS, Iwano M, Tanaka N, Takayama S, Minami E, Shibuya N, Kadota I, Isogai A. Plant Cell Physiol. 1999;40:1036–1045.10.1093/oxfordjournals.pcp.a029485
- Tanaka N, Nakajima Y, Kaneda T, Takayama S, Che FS, Isogai A. Plant Biotechnol. 2001;18:295–299.10.5511/plantbiotechnology.18.295
- Iwano M, Che FS, Goto K, Tanaka N, Takayama S, Isogai A. Mol. Plant Pathol. 2002;3:1–8.10.1046/j.1464-6722.2001.00087.x
- Tanaka N, Che FS, Watanabe N, Fujiwara S, Takayama S, Isogai A. Mol. Plant Microbe Interact. 2003;16:422–428.10.1094/MPMI.2003.16.5.422
- Fujiwara S, Tanaka N, Kaneda T, Takayama S, Isogai A, Che FS. Mol. Plant Microbe Interact. 2004;17:986–998.10.1094/MPMI.2004.17.9.986
- Cheng SH, Willmann MR, Chen HC, Sheen J. Plant Physiol. 2002;129:469–485.10.1104/pp.005645
- Harper JF, Binder BM, Sussman MR. Biochemistry. 1993;32:3282–3290.10.1021/bi00064a010
- Romeis T, Piedras P, Jones JD. Plant Cell. 2000;12:803–816.
- Hegeman AD, Rodriguez M, Han BW, Uno Y, Phillips GN Jr., Hrabak EM, Cushman JC, Harper JF, Harmon AC, Sussman MR. Proteomics. 2006;6:3649–3664.10.1002/(ISSN)1615-9861
- Romeis T, Ludwig AA, Martin R, Jones JD. EMBO J. 2001;20:5556–5567.10.1093/emboj/20.20.5556
- Jackson CL, Casanova JE. Trends Cell Biol. 2000;10:60–67.10.1016/S0962-8924(99)01699-2
- Cox R, Mason-Gamer RJ, Jackson CL, Segev N. Mol. Biol. Cell. 2004;15:1487–1505.10.1091/mbc.E03-06-0443
- Nielsen ME, Feechan A, Böhlenius H, Ueda T, Thordal-Christensen H. Proc. Nat. Acad. Sci. USA. 2012;109:11443–11448.10.1073/pnas.1117596109
- Yamaguchi K, Imai K, Akamatsu A, Mihashi M, Hayashi N, Shimamoto K, Kawasaki T. Plant J. 2012;70:389–397.10.1111/tpj.2012.70.issue-3
- He Z, Li L, Luan S. Plant Physiol. 2004;134:1248–1267.10.1104/pp.103.031005
- Romano P, Gray J, Horton P, Luan S. New Phytol. 2005;166:753–769.10.1111/j.1469-8137.2005.01373.x
- Fellerer C, Schweiger R, Schöngruber K, Soll J, Schwenkert S. Mol. Plant. 2011;4:1133–1145.10.1093/mp/ssr037
- Kaneda T, Taga Y, Takai R, Iwano M, Matsui H, Takayama S, Isogai A, Che FS. EMBO J. 2009;28:926–936.10.1038/emboj.2009.39