Abstract
Brassinosteroids are plant steroid hormones that regulate plant organs and chloroplast development. The detailed molecular mechanism for plant development by BR signaling is yet to be revealed, and many points regarding the relationship between BR signaling and chloroplast development remain unknown. We identify here the dominant mutant Brz-insensitive-pale green3-1D (bpg3-1D) from the Arabidopsis FOX lines that show reduced sensitivity to the chlorophyll accumulation promoted by the BR biosynthesis inhibitor, Brassinazole (Brz), in the light. BPG3 encodes a novel chloroplast protein that is evolutionally conserved in bacteria, algae, and higher plants. The expression of BPG3 was induced by light and Brz. The inhibition of electron transport in photosystem II of the chloroplasts was detected in bpg3-1D. These results suggest that BPG3 played an important role in regulating photosynthesis in the chloroplast under BR signaling.
Graphical Abstract
BR-inhibitor Brz activated chloroplast functions. bpg3 (Brz-insensitive-pale green3) mutant is disrupted in the signaling from BR-deficient condition to chloroplast activation.
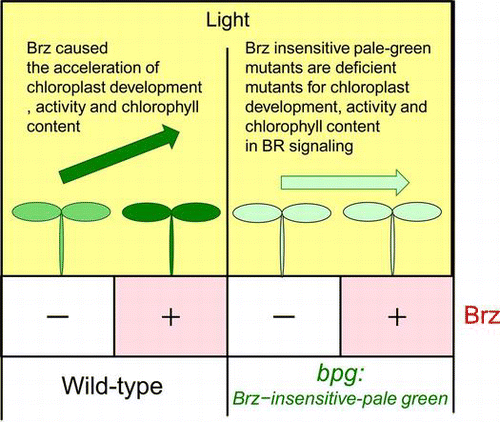
Brassinosteroids (BRs) are plant steroid hormones that regulate various processes of plant development, including photomorphogenesis, leaf expansion, stem elongation, leaf elongation, and xylem differentiation. In the past two decades, genetic studies have revealed the phenotypic characterization of mutants that affect BR synthesis and signaling.
deetiolated2 (det2) was first isolated as a mutant with a photomorphogenesis phenotype in the darkCitation1) and was later identified as the first mutant to be deficient in the BR biosynthesis enzymes of Arabidopsis thaliana (hereafter Arabidopsis). DET2 encodes a steroid-5α-reductase involved in BR biosynthesis which has the ability to catalyze human steroid-5α-reduction.Citation2–4) det2 was observed to have a short hypocotyl and open cotyledons in the dark and to have dark-green and round leaves in the light. In dark-grown det2 mutants, the increased expression of light-induced photosynthetic genes and translation of their produced proteins were generally observed. The photosynthetic genes highly expressed in det2 were rbcS, the small subunit of ribulose-1,5-bisphosphate carboxylase oxygenase (Rubisco SSU), and psbA, the D1 protein of photosystem II, that were major proteins of photosynthesis in chloroplasts. These results suggested that the BR deficiency in det2 activated the transcription of photosynthesis genes in chloroplasts. Several BR-deficient mutants have subsequently been isolated based on the det2 phenotype. Some were BR biosynthesis-deficient mutants in which the addition of BR resolved the dwarf phenotype: dwarf4 (dwf4)Citation5,6)and constitutive photomorphogenesis and dwarfism (cpd)Citation7). Some were BR signaling-deficient mutants that were insensitive to BR and in which the dwarf phenotype could not be rescued by BR: bri1Citation4,8) and bin2.Citation9,10) The regulatory mechanism for plant development in these mutants has been well analyzed, and the results suggested the effect of a BR deficiency on plant growth. These mutants also showed the dark green leaf phenotype in the light and the de-etiolation phenotype in the dark. These results suggested that a BR deficiency regulated not only plant development but also the promotion of chloroplast differentiation. Nevertheless, more detailed analyses of the correlation between chloroplast differentiation and BR have not been performed. Studies to clarify the molecular mechanism for BR regulation of chloroplast differentiation are required.
Brassinazole (Brz) is the specific inhibitor of BR biosynthesis at the step of P450 steroid C-22 hydroxylase, encoded by DWF4/CYP90B1. Brz causes the de-etiolation phenotype in the dark and dwarf phenotype in the light in Arabidopsis, similar to the phenotypes of BR-deficient mutants. These Brz phenotypes have been rescued by treating with BR.Citation10–12) Biochemical analyses have recently revealed the molecular mechanism for BR signaling in plant development that was not clarified by analyses of BR-deficient mutants using Brz screening. Brz-insensitive-long hypocotyl 1-1D (bil1-1D) has been isolated from EMS-mutation linesCitation12) and it showed longer hypocotyls than the wild-type with Brz in the dark. bil1-1D has the same mutation as brassinazole-resistant 1-1D (bzr1-1D), which was independently screened by Brz, and BZR1 encodes a bHLH-type transcription factor with dual functions involving BR biosynthesis genes and growth regulatory genes.Citation13,14)
The BR deficiency caused by Brz activated the photosynthesis-related gene expression and protein translation. In the light-grown wild-type, the level of the chloroplast-encoded large subunit of the ribulose-1,5-bisphosphate carboxylase oxygenase (Rubisco LSU) protein from Brz-treated seedlings was increased approximately 1.4-fold and that of the photosystem II D1 protein’s increased approximately 1.3-fold when compared to that of seedlings that had not been treated with Brz.Citation15) In the light-grown wild-type, the levels of the nuclear-encoded light-harvesting chlorophyll a/b binding protein (CAB/LHCP) were increased approximately 1.3-fold, and the Rubisco SSU protein was increased approximately 1.6-fold by Brz treatment.Citation15) In the same light-grown condition, the chlorophyll content was increased by Brz treatment.Citation15) These four proteins and chlorophyll were core factors for plant photosynthesis in chloroplasts. These results suggest that Brz generally up-regulated the activity of chloroplast as a photosynthesis organelle.
To analyze the molecular mechanism for BR signaling that regulates chloroplast functions, we screened Brz-insensitive bpg (Brz-insensitive-pale green) mutants that showed reduced sensitivity to chlorophyll accumulation by Brz in the light. We have previously reported Brz-insensitive-pale green 2 (bpg2) as an Arabidopsis recessive mutant.Citation15) The cotyledons of bpg2 were insensitive to Brz, which induced the promotion of greening in the cotyledon. BPG2 encodes a chloroplast-localized protein with four GTP-binding domains, and its deficiency caused the abnormal accumulation of the precursors of 16S and 23S rRNA transcribed from the chloroplast genome. The chloroplast protein accumulation induced by Brz was suppressed by the bpg2 mutation. These results suggest that BPG2 plays important roles in post-transcription and translation in the chloroplast with BR and Brz.
We isolated here and characterized the novel mutant, Brz-insensitive-pale green 3-1D (bpg3-1D), from full-length cDNA overexpressor (FOX) linesCitation16) that showed pale-green cotyledons and were insensitive to the Brz-induced promotion of greening in the light. Although bpg2 is a recessive mutant, bpg3-1D is the first known dominant mutant of this type. BPG3 encodes a novel chloroplast protein that has been evolutionally conserved in bacteria, algae, and higher plants. The possible function of BPG3 in the photosynthesis in chloroplasts is discussed.
Materials and methods
Plant material and growth conditions
A. thaliana Columbia-0 (Col-0) was used as the wild-type. The plants were germinated and grown on a 1/2 Murashige and Skoog (MS) medium (Duchefa, http://www.duchefa.com) containing 1.5% sucrose and 0.9% phyto-agar (Duchefa), with or without Brz. The germinated plants were transferred to soil, and grown under 16-h light (100 μE m−2 s−1 of white light)/8-h dark cycle at 22.
Insert identification
DNA was extracted from the selected FOX line by using a Nucleon extraction kit (GE healthcare, http://www.gehealthcare.com/) and then amplified by PCR, using the following primers: FOX-forward, 5′-GGAAGTTCATTTATTCGGAGAG-3′; FOX-reverse, 5′-GGCAACAGGATTCAATCTTAAG-3′.
Measurement of chlorophylls a and b
Chlorophyll was extracted from 14-d-old seedlings grown in the light (100 μE m−2 s−1) or in the weak light (20 μE m−2 s−1) under long days (16-h light/8-h dark). The bpg3-1D analysis (Fig. ) was performed under the weak light condition, and the BPG3-OX analysis (Fig. ) was performed under the light condition. The plants were homogenized in 80% v/v acetone. The chlorophyll content of the centrifuged supernatants was determined by the absorbance at 645 and 663 nm. The chlorophyll a and b contents were determined as previously described by Arnon (1949):
Chlorophyll a (μg/mg fresh weight) =
Chlorophyll b (μg/mg fresh weight) =
Generating the transgenic plants
In the recapitulation of the bpg3-1D phenotype, BPG3 cDNA was amplified from Arabidopsis wild-type Col-0 cDNA with the following primers: BPG3-forward, 5′-CACCATGAAGCCCACGACCAATGG-3′; and BPG3-reverse 5′-TCATTGATTCTTAAGATCATCC-3′. The PCR product was cloned into the pENTR/D-TOPO vector (Invitrogen, http://www.invitrogen.com/). The resulting pENTR-BPG3 vector was further cloned into binary vector pGWB2Citation17) (http://shimane-u.org/nakagawa/gbv.htm), which contained a CaMV 35S promoter. The BPG3-GFP construct was generated for subcellular localization. The open reading frame (ORF) of BPG3 was amplified from Arabidopsis Col-0 cDNA with the following primers: BPG3-forward, 5′-CACCATGAAGCCCACGACCAATGG-3′; and BPG3-GFP-reverse, 5′-TTGATTCTTAAGATCATCCATAC-3′. The PCR product was cloned into the pENTR/D-TOPO vector and subsequently cloned into binary vector pGWB5.Citation17) The generated constructs were transformed into Col-0 by the Agrobacterium-mediated floral dip method. The transgenic plants were screened on 1/2 MS agar plates containing 25 μg mL−1 of kanamycin.
Quantitative real-time PCR
Total RNA was extracted by an RNeasy Plant Mini Kit (Qiagen, http://www.qiagen.com/) from light-grown, 7-d-old wild-type, and bpg3-1D seedlings. The first-strand cDNA was synthesized by using PrimeScript (Takara, http://www.takara-bio.co.jp/) and was used in quantitative real-time PCR (Takara, Thermal Cycler Dice TP 800) with the SYBR Premix ExTaq system (Takara). The sequences of the gene-specific primers were as follows: BPG3, BPG3-RT-forward, 5′-TCCAAATCCAAACGTCGTCA-3′; and BPG3-RT-reverse 5′-CGGTGGTGCGACGAGAAT-3′; constitutively expressed control gene ACT2 (At3g18780), ACT2-RT-forward 5′-CGCCATCCAAGCTGTTCTC-3′; and ACT2-RT-reverse 5′-TCACGTCCAGCAACGTCAAG-3′.
Chlorophyll fluorescence measurements
The chlorophyll fluorescence was measured from light-grown, 10-d-old wild-type, and bpg3-1D seedlings with the FluorCam chlorophyll fluorescence monitoring system (Photon Systems Instruments, http://www.psi.cz/). The plants were adapted to the dark for 30 min before measurement. The chlorophyll fluorescence parameters were determined as described by Schreiber et al. (1988):
The level of minimum fluorescence (Fo) was recorded by exposure to non-actinic measuring flashes. The level of maximum fluorescence (Fm) was obtained by applying a 0.8-s saturating light pulse (600 μE) from a light source. The level of maximum fluorescence during illumination () was obtained with a saturating pulse during actinic illumination. The level of minimum fluorescence during illumination (
) was obtained after turning off the actinic light. Fs is the level of fluorescence yield during illumination.
Results
Isolation of the bpg3-1D mutant
Brz directly binds and inhibits cytochrome P450 steroid C-22 hydroxylase encoded by the DWF4 gene. The Brz treatment resulted in the dwarf phenotype in the light, and the de-etiolation phenotype, which included the induction of chloroplast gene expression, in the dark. In the light, the Brz treatment increased the protein levels of nuclear-encoded LHCP and Rubisco SSU, and chloroplast-encoded D1 protein and Rubisco LSU.Citation15) The Brz treatment in the light also increased greening of the cotyledons in wild-type Arabidopsis. These four photosynthesis proteins and chlorophyll suggested by the green color of the leaves are considered to have been related to the photosynthesis activity in chloroplasts. If a pale-green mutant was not related to BR deficiency by Brz, the pale-green leaves could recover the green color. If a pale-green mutant in which the color is not recovered by Brz could be isolated, the phenotype was considered to have been caused by a decrease in or the disruption of BR signaling for chloroplast regulation. We then considered that the method could isolate chloroplast-regulating mutants that were related to BR signaling (Fig. ). Based on this hypothesis, we isolated the gene-disrupted mutant, Brz-insensitive-pale green2 (bpg2). BPG2 encodes a chloroplast-localized protein and plays important roles in the post-transcription and translation of chloroplasts with Brz. Citation15)
Fig. 1. Screening Strategy for the bpg (Brz-insensitive-pale green) mutants.
Notes: BR inhibitor Brz activated the chloroplast functions. Possible bpg mutants were disrupted in signaling from the BR-deficient condition to chloroplast activation. The BPG genes resulting from the mutants played important roles in chloroplast regulation for BR signaling.
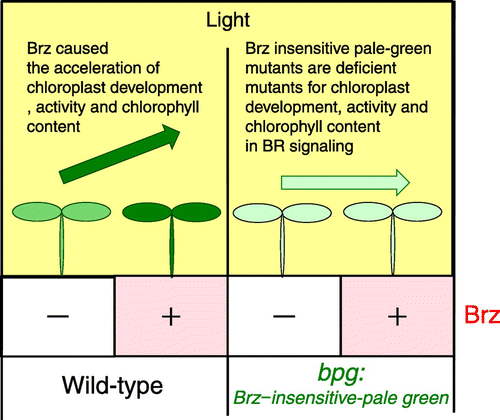
We initially screened approximately 10,000 Arabidopsis FOX linesCitation16) and isolated approximately 20 lines that showed the pale-green phenotype. We then isolated Brz-insensitive-pale green3-1D (bpg3-1D) from these 20 lines (Fig. (C) and (D)). The dominant phenotype of bpg3-1D in the T2 generation suggested that the mutation could be based on the FOX system. The cotyledons of bpg3-1D at the germination stage showed a paler green color than wild-type Arabidopsis on the medium containing 1 μM Brz (Fig. (A)–(D)). To provide a detailed analysis of greening in the cotyledons, the endogenous levels of chlorophyll a and b in wild-type and bpg3-1D seedlings germinated for 14 d were measured with or without Brz in the light (Fig. (E) and (F)). bpg3-1D with Brz accumulated lower amounts of chlorophylls a (Fig. (E)) and b (Fig. (F)) than the wild-type seedlings with Brz. The wild-type seedlings showed an increase in the endogenous chlorophyll a and b levels by the Brz treatment, but the bpg3-1D seedlings did not.
Fig. 2. Identification of the bpg3-1D mutant as a pale-green phenotype by the Brz treatment.
Notes: (A)–(D), Cotyledons of the wild-type ((A) and (B)) and bpg3-1D ((C) and (D)) grown on medium for 4 d without Brz ((A) and (C)) or with 1 µM Brz ((B) and (D)). Scale bars, 1 mm. (E)–(F), Endogenous contents of chlorophyll a (E) and chlorophyll b (F) in wild-type (WT) and bpg3-1D plants grown for 14 days without Brz or with 0.3 and 1 µM Brz under long days (16-h light/8-h dark). Error bars indicate SE (n = 3). (G)–(H), Wild-type (G), and bpg3-1D (H) plants grown on soil for 2 weeks. Scale bars, 10 mm. (I) Wild-type (WT), and bpg3-1D plants grown under long days (16-h light/8-h dark) on soil for 3 weeks. Scale bar, 5 cm.
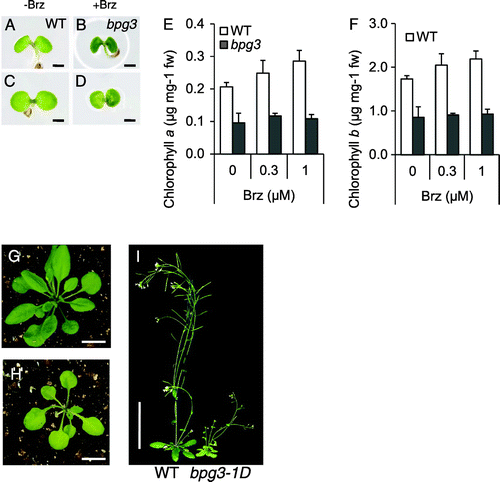
When grown on soil, bpg3-1D developed semi-dwarf and pale-green rosette leaves (Fig. (G) and (H)) and shortened inflorescences (Fig. (I)) when compared with the wild-type. Although dwarf and dark green phenotypes were found in BR-deficient mutant det2 and BR-insensitive mutant bri1, the bpg3-1D mutant showed a dwarf and pale-green phenotype. These phenotypes of bpg3-1D might have been caused by growth inhibition related to the low chlorophyll accumulation.
BPG3 is a novel protein that has been evolutionally conserved from higher plants to photosynthetic bacteria
FOX lines are designed to exhibit phenotypes by the overexpression of full-length cDNAs under the control of the strong CaMV 35S promoter and nopaline synthetase (NOS) terminator. To isolate the cause of the bpg3-1D mutant, inserted cDNA was amplified by the polymerase chain reaction (PCR), using specific primers for the 35S promoter and NOS terminator. The resulting nucleotide sequence showed possible cDNA encoded by At2g40400, which was a novel gene. In the bpg3-1D mutant, the expression of At2g40400 was approximately 12-fold higher than in the wild-type (Fig. (B)).
Fig. 3. Structure of the BPG3 gene.
Notes: (A) Phylogenic relationship among BPG3 and BPG3 homologous proteins. (B) Quantitative real-time PCR analysis of BPG3 (At2g40400) mRNA expression in the hypocotyls of wild-type (WT) and bpg3-1D plants. The BPG3 gene was overexpressed in the bpg3-1D mutant. The value was normalized against the expression of the ACT2 gene. Error bars indicate SD (n = 3). (C) Structure of BPG3. BPG3 has two DUF domains and two transmembrane regions. (D) Model to illustrate BPG3 evolution. In bacteria, the ancestor-type BPG3 protein contained only the DUF 399 domain. In algae, chloroplasts after symbiosis of ancestral cyanobacteria, two short proteins, had either the DUF 399 or DUF 3411 domain existing in parallel. In the chloroplasts of higher plants after their evolution from ancestral algae, these two short proteins were combined in tandem, and the BPG3 protein family emerged. Red box, DUF 399; yellow box, DUF 3411; black box, possible transit peptide. (E) Sequence alignment of BPG3 and BPG3 homologs from higher plants. Red box, DUF399 domain; yellow box, DUF3411 domain; green box, transmembrane domain. Accession numbers: BPG3, NP_850329; A. lyrata, XP_002879867; At3G56160, NP_191173; M. trunacatula, XP_003601002; G. Max, XP_003552200; S. lycopersium, XP_00424089; C. sativus, XP_004138409; F. vesca, XP_004308966; V. vinifera, XP_002278231; P. trichocarpa, XP_002311251; R. communis, XP_002520805; Z. mays, NP_001130529; S. bicolor, XP_002458700; O. sativa, NP_001044677; B. distachyon, XP_003564514; P. patens, XP_001758981; S. moellendorffi, XP_002979031; C. reinhardtii, XP_001703430; V. carteri_3411_type, XP_003080339; O. lucimarinus; XP_001718701; O. tauri, XP_003080339; C. merolae_3411_type, kegg_T00175; A. marina, YP_00151818190; T. elomgatus, NP_682054; Cyanothece_sp., YP_002483463; C. epipsammum, YP_007142727; Microcoleus_sp., YP_007120179; O. acuminata, YP_007085703; C. thermalis, YP_007092813; G. sulfurreducens_PCA, NP_954180; G. sulfurreducens_KN400, YP_005834996; V. carteri_399_type, XP_002954960; C. merolae_399_type, GenBank_BAM79152. Amino acid sequence alignments were generated by ClustalX software. The phylogenic tree was constructed by the UPGMA method from the amino acid sequences.
The product of At2g40400 has been evolutionally conserved in many types of higher plants, green algae, red algae, photosynthetic bacteria, and bacteria. A detailed analysis by BLAST searches for the amino acid sequence of the At2g40400 product identified similar genes in Arabidopsis (AGI encodes At3g56140, an unknown protein), rice (Oryza sativa), Medicago truncatula, grape (Vitis vinifera), soybean (Glycine max), moss Physcomitrella patens, green algae Ostreococcus lucimarinus, red algae C. merolae, cyanobacterium Oscillatoria acuminata, and bacterium Geobacter sulfurreducens (Fig. (A)).
An alignment analysis of the At2g40400 protein with the homologous proteins revealed the novel protein family to contain two conserved domains, a domain of unknown function (DUF) 399 (http://pfam.sanger.ac.uk/family/DUF399) and/or a DUF 3411 (http://pfam.sanger.ac.uk/family/DUF3411) (Fig. (C) and (E)). The evolutionary conservation hypothesis of the two domains is considered in the discussion section.
The bpg3-1D phenotype was recaptured by the overexpression of At2g40400 cDNA
To confirm that the overexpression of At2g40400 was responsible for the bpg3-1D phenotype, the At2g40400 coding region was immediately placed downstream of the CaMV 35S promoter and transformed into wild-type Arabidopsis. The obtained transformants, BPG3-OX1 and BPG3-OX2, showed a higher expression of At2g40400 mRNA than the wild-type by real-time PCR (RT-PCR); (Fig. (A)). At the germination stage, the bpg3-1D mutant and the BPG3-OX1 and BPG3-OX2 transformants showed a pale-green phenotype with Brz in the light (Fig. (D)–(I)) when compared with the wild-type (Fig. (B) and (C)). The endogenous levels of chlorophylls a and b were increased by Brz in the wild-type, but BPG3-OX1 and BPG3-OX2 accumulated lower amounts of chlorophylls a and b than the wild-type Arabidopsis (Fig. (J) and (K)). At the mature growth stage, BPG3-OX1 and BPG3-OX2 showed a pale-green and semi-dwarf phenotype (Fig. (M)–(O)) when compared with wild-type Arabidopsis (Fig. (L)). The BPG3-deficient mutant, BPG3-KO (SALK_116717), did not show a significant phenotype (Fig. (R) and (S)) when compared with the wild-type (Fig. (P) and (Q)). This phenotype might have been the result of redundancy of the BPG3 gene. These results suggest that the bpg3-1D phenotype was caused by the overexpression of At2g40400 cDNA.
Fig. 4. Overexpression of BPG3 recaptured the pale-green phenotype of bpg3-1D.
Notes: (A) Quantitative real-time PCR analysis of BPG3 (At2g40400) mRNA expression in hypocotyls of the wild-type (WT) and BPG3-OX transformants. The BPG3 gene was overexpressed in the BPG3-OX mutant. The value was normalized against the expression of the ACT2 gene. Error bars indicate SD (n = 3). B-I, Cotyledons of wild-type ((B) and (C)), bpg3-1D ((D) and (E)), BPG3-OX1 ((F) and (G)) and BPG3-OX2 (H and I) plants grown on 1/2 MS medium for 5 d without Brz ((B), (D), (F), and (H)) or with 1 µM Brz ((C), (E), (G), and (I)) under long days (16-h light/8-h dark). Scale bars, 1 mm. (J)–(K), endogenous contents of chlorophyll a (J) and chlorophyll b (K) in wild-type (WT), bpg3-1D, BPG3-OX1 and BPG3-OX2 plants grown on medium for 14 d without Brz or with 0.3 and 1 µM Brz under long days (16-h light/8-h dark). The error bars indicate SE (n = 3). (L)–(O), Rosette leaf morphology of wild-type (L), bpg3-1D (M), BPG3-OX-1 (N), and BPG3-OX-2 (O) plants grown on soil for 3 weeks under long days (16-h light/8-h dark). Scale bars = 10 mm. (P)–(S), Cotyledons of wild-type ((P) and (Q)) and BPG3-KO ((R) and (S)) plants grown on 1/2 MS medium for 5 d without Brz ((P) and (R)) or with 1 µM Brz ((Q) and (S)) under long days (16-h light/8-h dark). Scale bars, 1 mm.
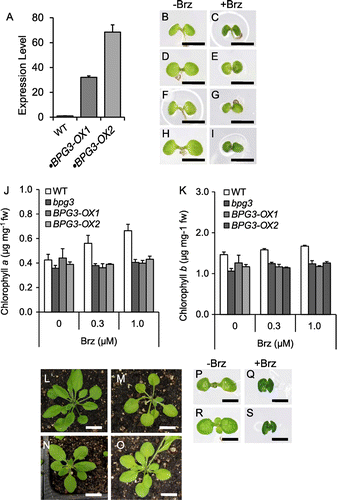
BPG3 was localized in the chloroplast
To determine the subcellular localization of the BPG3 protein, the CaMV 35S promoter and BPG3-GFP fusion construct was transformed into wild-type Arabidopsis. GFP fluorescence was detected in the chloroplasts of guard cells of the 35S::BPG3-GFP plants (Fig. (A)), and the fluorescence signal (Fig. (B)) was co-localized with chlorophyll autofluorescence (Fig. (C) and (D)). These results suggest that the BPG3 protein was localized in the chloroplasts.
Fig. 5. BPG3 protein localized in the chloroplasts.
Notes: Confocal laser scanning microscopy of guard cells in 35S::BPG3-GFP transformants ((A)–(D)). The plants were grown for 14 d in the light on medium containing kanamycin. (A) Green fluorescence indicating BPG3-GFP localization in the guard cells. (B) Red autofluorescence of chlorophyll. (C) Bright-field images. (D) Merged image of (A)–(C). Scale bar, 10 µm.
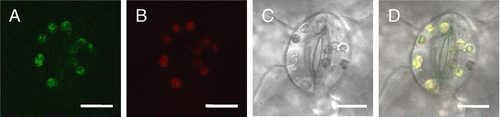
BPG3 mRNA was induced by Brz
In the BR biosynthesis enzyme-disrupted mutant det2, the expressions of photosynthesis genes, rbcS and psbA were up-regulated.Citation1) In the wild-type Arabidopsis treated by BR-biosynthesis inhibitor Brz, the expression and translation of photosynthesis genes rbcS, rbcL, CAB, and psbA were up-regulated.Citation15) These results suggested that the BR biosynthesis-deficient condition induced chloroplast gene expression in both the nuclear and chloroplast genomes. To investigate the relationship between Brz and BPG3 gene expression, the expression levels of the BPG3 gene were analyzed by RT-PCR. In the long-term treatment with Brz of wild-type Arabidopsis germination for seven days, the BPG3 expression in Brz-treated plants was induced 4-fold higher in the dark and 2-fold higher in the light when compared with plants without the Brz treatment (Fig. (A)).
Fig. 6. Expression of BPG3 mRNA is induced by Brz.
Notes: Quantitative real-time PCR analysis of BPG3 mRNA expression in the hypocotyls of wild-type plants treated with or without Brz grown under long days (16-h light/8-h dark) or in the dark. Error bars indicate SD (N = 3). (A) Wild-type plants treated with continuous stimulation for 7 d using 3 µM Brz. (B) Wild-type plants treated with transient stimulation for 3 h using 0, 0.3, 1.0, or 3.0 µM Brz after being light-grown for 7 d.
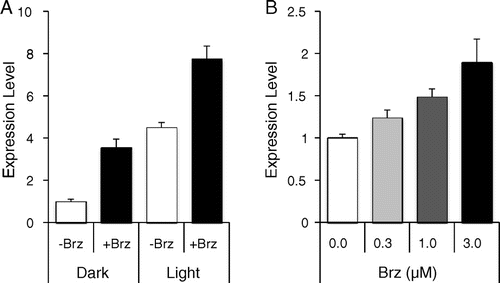
Similar to the plants continuously treated with Brz, transient stimulation with Brz for 3 h in the light also induced BPG3 expression (Fig. (B)). This induction of BPG3 expression was in proportion to the Brz concentration. The BL treatment could not affect BPG3 expression under these conditions (Table ). The functional relationship between BPG3 and BR signaling is considered in the discussion section.
Table 1. Expression of the chlorophyll biosynthesis genes and BPG3 in Col-0 and det2.
Table 2. Photosynthetic characteristics of bpg3-1D plants.
BPG3 did not affect gene expression in the chloroplasts but did affect photosynthetic activity
Given that BPG3 was localized in the chloroplasts, one possible role of BPG3 could be in regulating the important parts of the chloroplast. To investigate the molecular functions of BPG3 responsible for the pale-green phenotype, the expression of photosynthesis genes encoded by the chloroplast genome in bpg3-1D was first analyzed by RT-PCR. However, the expression of rbcL, the large subunit of Rubisco, and psbA, a D1 protein of photosystem II, in bpg3-1D was similar to that of the wild-type (data not shown).
We then attempted to measure the kinetics of chlorophyll fluorescence in bpg3-1D and the wild-type plants by the FluorCam, chlorophyll fluorescence monitoring system (Table ). The electron transport conditions in photosystem II (PSII) on the chloroplast thylakoid membrane can be principally predicted by this system. In the bpg3-1D mutant, the maximum quantum yield of PSII (Fv/Fm), effective quantum yield of PSII (Fv′/Fm′), quantity of effective photosynthetic electron transport (ϕII), and non-photochemical quenching (NPQ) were calculated as being lower than in wild-type Arabidopsis. The parameter for photochemical quenching (qP) in bpg3-1D was the same as that in the wild-type. The possible function of BPG3 in PSII suggested by these results is presented in the discussion section.
Discussion
BRs and their biosynthesis inhibitor, Brz, can regulate not only plant development but also chloroplast development. The BR-deficient mutant and Brz-treated wild-type germinated in the dark have shown photomorphogenesis and increased photosynthetic gene expression, despite the darkness.Citation11) In the light-grown wild-type Arabidopsis, the expression and translation of the photosynthesis genes, rbcS, rbcL, CAB, and psbA, have also been activated.Citation15) We also found that the chlorophyll contents in the light-grown cotyledon were increased under the BR-deficient conditions with Brz (Fig. ). Based on the microarray method, we analyzed the expression levels of the chlorophyll biosynthesis genes under the BR-deficient and BR-treated conditions (Table ) (http://www.arabidopsis.org/portals/expression/microarray/ATGenExpress.jsp)Citation18). Although the difference of chlorophyll biosynthesis gene expressions between wild-type and BR biosynthesis-deficient mutant det2 was not substantial, the upstream genes of the chlorophyll biosynthesis pathway were up-regulated in det2 compared to wild-type. Conversely, the expressions of the chlorophyll biosynthesis genes were mostly down-regulated in BR-treated det2 when compared to the wild-type. We also observed that the accumulation of a crystalized structure of protochlorophyllide under the Brz-treatment conditions was higher than the non-treated conditions.Citation11) Although only the gene expression level could not suggest the final contents of chlorophyll, these characteristics in change in gene expression might suggest the relationship between BR and chlorophyll biosynthesis.
We also clarified that light-harvesting chlorophyll a/b binding protein (CAB/LHCP) was increased approximately 1.3-fold by the Brz-treatment.Citation15) An earlier study suggested that the synthesized chlorophylls were stabilized by LHCP. Stabilization of LHCP by chlorophyll b has also been generally observed in plants. This reciprocal stabilization between LHCP and chlorophyll is considered to be a very important molecular mechanism to regulate the chlorophyll cycle.Citation19) The up-regulated accumulation of LHCP by BR deficiency with Brz could actually support the stabilization, and the accumulation of chlorophyll is up-regulated by BR deficiency with Brz.
The identification of a regulatory gene in the BR-signaling pathway involved in chloroplast development is considered to be required, based on the physiological knowledge. We had previously identified and reported the first Brz-insensitive-pale green mutant, bpg2, as a knockout line by the insertion of a tagging vector. BPG2 encodes a chloroplast GTP-binding protein that regulates the processing of 16S and 23S rRNA from the chloroplast genome and translation in the chloroplast.Citation15) We screened in this present study, a novel bpg mutant and identified the dominant mutant, bpg3-1D. Brz increased the endogenous chlorophyll levels in wild-type Arabidopsis, but chlorophyll synthesis was not increased in the bpg3-1D mutant, in which BPG3 mRNA expression was activated (Fig. ). These results suggest that BPG3 was involved in greening of the chloroplast in BR signaling. BPG3 encoded a novel chloroplast protein containing two transmembrane regions and two domains of unknown function, DUF399 and DUF3411.
A homology search suggested that BPG3 was evolutionally conserved in bacteria, cyanobacteria, rhodophyta, chlorophyta, and embryophyta (Fig. ). In higher plants, BPG3 and its homolog in M. truncatula have been predicted to be localized in the thylakoid.Citation20) Chloroplast localization of the BPG3-GFP protein was apparent (Fig. ). In the higher plants of embryophyta, both of the domains of unknown function, DUF399 and DUF3411, are highly conserved in a long protein with 700 amino acids (Fig. ). In algae, chlorophyta and rhodophyta, a short protein of approximately 500 amino acids containing only the DUF 3411 domain is highly homologous (approximately 40% identity) to the BPG3-C terminal sequence. Additionally, chlorophyta V. carteri and rhodophyta C. meloae also have a short protein of approximately 500 amino acids with only the DUF399 domain that is homologous to the BPG3-N terminal sequence (Fig. ). In bacteria and cyanobacteria, a short protein of approximately 500 amino acids with only the DUF399 domain that is highly homologous (approximately 28% identity) to the BPG3-N terminal sequence is present (Fig. ). Based on these results, we propose the hypothesis for BPG3 protein evolution (Fig. ) that the ancestor of the BPG3 protein might have contained only the DUF399 domain in ancestral cyanobacteria. After the symbiosis of ancestral cyanobacteria and ancestral algae, the DUF3411-type protein emerged in addition to the DUF399-type protein and functioned in parallel in the chloroplasts. During the evolution from algae to higher plants, these two proteins were connected in tandem, and the evolutionally advanced BPG3 protein family was established. Although the protein highly homologous to the cyanobacteria DUF399-type protein could not be identified in the current genome of algae, proteins similar to the cyanobacteria DUF399-type protein might be found by future research in algae genomes.
The Psort prediction (http://wolfpsort.org/) suggested that BPG3 might be localized in the thylakoid membrane in chloroplasts. To reveal the function of BPG3 in the thylakoid membrane, the condition of PSII electron transport in bpg3-1D was analyzed by monitoring the chlorophyll fluorescence (Table ). The bpg3-1D plants showed lower Fv/Fm, Fv′/Fm′, and ϕII values than the wild-type. These parameters have suggested the condition of electron transport in total PSII.Citation21) The qP parameter was the same in bpg3-1D and wild-type plants. The qP parameter has suggested the condition of a redox state of the primary quinone receptor of PSII (QA).Citation21) These results suggest that BPG3 played an important role in electron transport in PSII. The parameter of NPQ was also lower in bpg3-1D. NPQ is known to dissipate excess light energy as heat and be triggered by acidification of the thylakoid lumen induced by electron transport.Citation21) Low NPQ of bpg3-1D might have been due to impaired electron transport in PSII. The pale-green phenotype in bpg3-1D might have been caused by the photoinhibition that is frequently due to the inhibition of PSII.
The expression analysis revealed that BPG3 mRNA was increased by the Brz treatment, but was not affected by BR treatment. Additional BR treatment caused weak changing of the phenotype for the plant, but loss of BR signaling in the BR receptor BRI1-deficient mutant has shown a significant dwarf phenotype.Citation4) Many studies have shown that BRI1 was clearly related with BR signaling and that downstream events were related with BR.Citation22) Our research suggested that the overexpression of BPG3 physiologically affected chloroplasts in the BR-deficient condition. If a pale-green mutant is not related to BR deficiency by Brz, the pale-green leaves could recover the green color by Brz. As the pale-green color of bpg3-1D could not be recovered to green by Brz, the phenotype was caused by a decrease in or disruption of BR signaling for chloroplast regulation. Furthermore, bzs1-1D (bzr1-1D suppressor1-Dominant) has been isolated from activation-tagging mutants, and the bzs1-D mutation caused by the overexpression of B-box zinc finger protein BZS1.Citation23) bzs1-1D showed normal hypocotyl elongation without Brz, but shorter hypocotyls in the presence of Brz than the wild-type. As bzs-1D and the mutant of BR master transcription factor, bzr1-1D (Brz-resistant1) double mutant showed shorter hypocotyls and smaller leaves than the bzr1-1D single mutant, bzs-1D is considered to be a BR-signaling mutant. Studies on the mutant phenotype and/or gene expressions that only appear under the BR-deficient condition might be advanced by future research.
Our results suggested BPG3 to be involved in the chloroplast regulation related with BR. Although the relationship between BPG3- and BR-related chloroplast regulation is reliable, the functional step of BPG3 in BR signaling is not clear. One possibility is that BPG3 is an intermediate player in the upstream step of chlorophyll for BR signaling, and the other possibility is that both BPG3 and chlorophyll are the most downstream players of BR signaling. Decreasing the chlorophyll contents in the BPG3-overexpressed mutant and transformants suggests that BPG3 and chlorophyll might be related to each other and play parallel roles in the downstream region of BR signaling. BPG3 might affect chlorophyll stability that is similar to the relationship between LHCP and chlorophyll.
The BR biosynthesis inhibitor, Brz, has induced the expression of photosynthetic gene psbA encoding the D1 protein in PSII, the rbcL gene encoding the RubisCo large subunit protein and other related genes.Citation15) The BPG3 mRNA was also induced by Brz. These results suggest BPG3 to have a positive function in photosynthesis, although the overexpression of BPG3 caused the pale-green phenotype and inhibition of the electron transport of PSII. The moderate and non-excessive expression of BPG3 might be required for healthy chloroplast conditions. Future analyses will reveal the detailed molecular mechanism for chloroplast regulation by BPG3 via BR signaling.
Supplemental material
The supplemental material for this paper is available at http://dx.doi.org/10.1080/09168451.2014.885831.
Supplementary Fig. 1 caption
Download MS Word (19 KB)Supplementary Fig. 1
Download MS Power Point (110.5 KB)Acknowledgments
We thank Dr. Tsuyoshi Nakagawa (Shimane University) for presenting the gateway vector, pGWBs. This work was supported in part by the Program for Promotion of Basic Research Activities for Innovation Bioscience (PROBRAIN) to T. N. and T. A. and by the Core Research for Evolutional Science and Technology (CREST) Program to T. N. and T. A.
Notes
Abbreviations: BPG3, Brz-insensitive-pale green 3; BPG3-OX, BPG3 overexpressor; BL, brassinolide; BR, brassinosteroid; BRI1, brassinosteroid-insensitive 1; BZR1, brassinazole-resistant 1; CaMV, cauliflower mosaic virus; DET2, de-etiolated 2; DWF4, dwarf 4; MS, Murashige and Skoog; Brz, Brz220; FOX, full-length cDNA overexpressor.
References
- Chory J, Nagpal P, Peto CA. Plant Cell. 1991;3:445–459.
- Li J, Nagpal P, Vitart V, McMorris TC, Chory J. Science. 1996;272:398–401.10.1126/science.272.5260.398
- Fujioka S, Li J, Choi YH, Seto H, Takatsuto S, Noguchi T, Watanabe T, Kuriyama H, Yokota T, Chory J, Sakurai A. Plant Cell. 1997;9:1951–1962.
- Li J, Chory J. Cell. 1997;90:929–938.10.1016/S0092-8674(00)80357-8
- Choe S, Dilkes BP, Fujioka S, Takatsuto S, Sakurai A, Feldmann KA. Plant Cell. 1998;10:231–243.
- Azpiroz R, Wu Y, LoCascio JC, Feldmann KA. Plant Cell. 1998;10:219–230.
- Szekeres M, Németh K, Koncz-Kálmán Z, Mathur J, Kauschmann A, Altmann T, Rédei GP, Nagy F, Schell J, Koncz C. Cell. 1996;85:171–182.10.1016/S0092-8674(00)81094-6
- Clouse SD, Langford M, McMorris TC. Plant Physiol. 1996;111:671–678.10.1104/pp.111.3.671
- Li J, Nam KH, Vafeados D, Chory J. Plant Physiol. 2001;127:14–22.10.1104/pp.127.1.14
- Asami T, Min YK, Nagata N, Yamagishi K, Takatsuto S, Fujioka S, Murofushi N, Yamaguchi I, Yoshida S. Plant Physiol. 2000;123:93–100.10.1104/pp.123.1.93
- Nagata N, Min YK, Nakano T, Asami T, Yoshida S. Planta. 2000;211:781–790.10.1007/s004250000351
- Asami T, Nakano T, Nakashita H, Sekimata K, Shimada Y, Yoshida S. J. Plant Growth Regul. 2003;22:336–349.
- Wang ZY, Nakano T, Gendron J, He J, Chen M, Vafeados D, Yang Y, Fujioka S, Yoshida S, Asami T, Chory J. Dev. Cell. 2002;2:505–513.
- He JX, Gendron JM, Sun Y, Gampala SS, Gendron N, Sun CQ, Wang ZY. Science. 2005;307:1634–1638.10.1126/science.1107580
- Komatsu T, Kawaide H, Saito C, Yamagami A, Shimada S, Nakazawa M, Matsui M, Nakano A, Tsujimoto M, Natsume M, Abe H, Asami T, Nakano T. Plant J. 2010;61:409–422.10.1111/tpj.2010.61.issue-3
- Ichikawa T, Nakazawa M, Kawashima M, Iizumi H, Kuroda H, Kondou Y, Tsuhara Y, Suzuki K, Ishikawa A, Seki M, Fujita M, Motohashi R, Nagata N, Takagi T, Shinozaki K, Matsui M. Plant J. 2006;48:974–985.10.1111/tpj.2006.48.issue-6
- Nakagawa T. J. Biosci. Bioeng. 2007;104:34–41.10.1263/jbb.104.34
- Goda H, Sasaki E, Akiyama K, Maruyama-Nakashita A, Nakabayashi K, Li W, Ogawa M, Yamauchi Y, Preston J, Aoki K, Kiba T, Takatsuto S, Fujioka S, Asami T, Nakano T, Kato H, Mizuno T, Sakakibara H, Yamaguchi S, Nambara E, Kamiya Y, Takahashi H, Hirai MY, Sakurai T, Shinozaki K, Saito K, Yoshida S, Shimada Y. Plant J. 2008;55:526–542.10.1111/tpj.2008.55.issue-3
- Tanaka R, Tanaka A. Biochim. Biophys. Acta. 2011;1807:968–976.10.1016/j.bbabio.2011.01.002
- Young ND, Debelle F, Oldroyd GE, Geurts R, Cannon SB, Udvardi MK, Benedito VA, Mayer KF, Gouzy J, Schoof H, Van de Peer Y, Proost S, Cook DR, Meyers BC, Spannagl M, Cheung F, De Mita S, Krishnakumar V, Gundlach H, Zhou S, Mudge J, Bharti AK, Murray JD, Naoumkina MA, Rosen B, Silverstein KA, Tang H, Rombauts S, Zhao PX, Zhou P, Barbe V, Bardou P, Bechner M, Bellec A, Berger A, Berges H, Bidwell S, Bisseling T, Choisne N, Couloux A, Denny R, Deshpande S, Dai X, Doyle JJ, Dudez AM, Farmer AD, Fouteau S, Franken C, Gibelin C, Gish J, Goldstein S, Gonzalez AJ, Green PJ, Hallab A, Hartog M, Hua A, Humphray SJ, Jeong DH, Jing Y, Jocker A, Kenton SM, Kim DJ, Klee K, Lai H, Lang C, Lin S, Macmil SL, Magdelenat G, Matthews L, McCorrison J, Monaghan EL, Mun JH, Najar FZ, Nicholson C, Noirot C, O’Bleness M, Paule CR, Poulain J, Prion F, Qin B, Qu C, Retzel EF, Riddle C, Sallet E, Samain S, Samson N, Sanders I, Saurat O, Scarpelli C, Schiex T, Segurens B, Severin AJ, Sherrier DJ, Shi R, Sims S, Singer SR, Sinharoy S, Sterck L, Viollet A, Wang BB, Wang K, Wang M, Wang X, Warfsmann J, Weissenbach J, White DD, White JD, Wiley GB, Wincker P, Xing Y, Yang L, Yao Z, Ying F, Zhai J, Zhou L, Zuber A, Denarie J, Dixon RA, May GD, Schwartz DC, Rogers J, Quetier F, Town CD, Roe BA. Nature. 2011;480:520–524.
- Shreiber U. Ecophysiology of photosynthesis. Vol. 100. Berlin: Springer; 1994.
- Zhu JY, Sae-Seaw J, Wang ZY. Development. 2013;140:1615–1620.10.1242/dev.060590
- Fan XY, Sun Y, Cao DM, Bai MY, Luo XM, Yang HJ, Wei CQ, Zhu SW, Sun Y, Chong K, Wang Z-Y. Mol. Plant. 2012;5:591–600.10.1093/mp/sss041