Abstract
We cloned the mouse mannosyltransferase IV/V gene (mALG11) from FM3A cells by a bioinformatic approach. The ORF contained 1476 bp encoding 492 amino acids. The cloned mALG11 complemented the growth defect of the Saccharomyces cerevisiae ALG11Δ mutant. In addition, we detected a variant cDNA by alternate splicing that had an additional four-nucleotide ATGC insertion at base 276 of the ORF. Consequently the variant cDNA encoded a truncated protein with 92 amino acids, lacking the glycosyltransferase group-1 domain. The variant cDNA occurs in many mouse strains according to EST database searches. Moreover, we detected it in FM3A cDNA, but we did not detect any such variants in the human EST database or in HeLa cDNA, although human ALG11 (hALG11) genomic DNA has the same sequence around the intron–exon boundaries as those of mALG11 genomic DNA. Hence, we concluded that there is different transcriptional control mechanism between mALG11 and hALG11.
Graphical Abstract
We cloned the mouse mannosyltransferase IV/V gene (mALG11) and its splice-variant from mouse FM3A cells. The latter variant was caused by an alternative donor site selection.
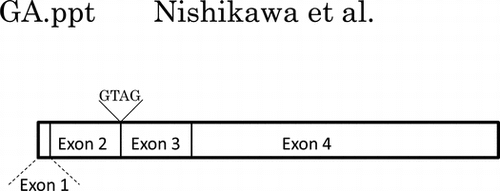
Lipid-linked oligosaccharide Glc3Man9GlcNAc2-P-P-Dolichol (Dol) (Fig. ) serves as a precursor to the asparagine-linked oligosaccharide of glycoproteins.
Fig. 1. Structure of the lipid-linked oligosaccharide.
Notes: The Man residues added by Alg11p are underlined in bold. ManT-4 and ManT-5 are mannosyltransferase IV and mannosyltransferase V, respectively. The mannose residue added by each mannosyltransferase is underlined with a narrow line.
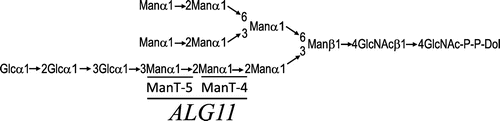
Synthesis of this lipid-linked oligosaccharide is initiated on the cytoplasmic face of the endoplasmic reticulum (ER) membrane, where two N-acetylglucosamine (GlcNAc) residues and five mannose (Man) residues are assembled on the lipid carrier dolichyl phosphate (Dol-P) with UDP-GlcNAc and GDP-Man, respectively, as sugar donors. After translocation of Man5GlcNAc2-P-P-Dol to the luminal face of the ER membrane, four Man residues and three glucose residues are transferred from Dol-P-Man and Dol-P-Glc, respectively, as sugar donors. This process is highly conserved among eukaryotic cells.Citation1)
The biosynthesis of lipid-linked oligosaccharides depends on a supply of Dol-P (de novo synthesis and recycling),Citation2) sugar donors (nucleotide- and dolichol-linked sugars) and acceptors, 11 glycosyltransferase-proteins for 14 steps,Citation1,3,4) and the presence of the Rft1 protein (hypothetical flippase for Man5GlcNAc2-P-P-DolCitation5)) and hypothetical flippase(s) for Dol-P-Man and/or Dol-P-Glc.
We have focused on the first half of the assembly of the lipid-linked oligosaccharide. Previously, we found for the first time that the de novo synthesis of the lipid-linked oligosaccharide is necessary for eukaryotic cells to traverse the G1 phase by finding that the cell growth of the human Burkitt lymphoma cell line Raji was reversibly arrested at the G1 phase of the cell cycle by tunicamycin, which inhibits GlcNAc-1-phosphate transferase.Citation6) Subsequently, these results were confirmed for Saccharomyces cerevisiae (S. cerevisiae).Citation7) The latter study took the initiative in isolation of the alg1 mutant in 1982Citation8) and alg2 to alg6 mutants in 1983Citation9) by the Robbins group.
In 1982, independently we isolated temperature-sensitive mutants possessing a defect in asparagine-linked glycosylation by the tritium suicide method from C3H/He mouse mammary tumor cell line FM3A.Citation10) One of these, the G258 mutant, had a defect in the synthesis of lipid-linked oligosaccharides.Citation11–15) The G258 mutant is temperature-sensitive for both cell growth and the synthesis of lipid-linked oligosaccharidesCitation11,12) due to a single gene mutation.Citation13) As the G258 mutant cannot elongate Man3GlcNAc2-P-P-Dol to Man4GlcNAc2-P-P-Dol at 39 °C, the non-permissive temperature, we concluded that it has a defect in mannosyltransferase IV activity at the non-permissive temperature. Then we isolated transformants by radiation hybridization between mouse G258 mutant cells and X-ray irradiated human HeLa cells. The transformants showed recovery from both forms of temperature sensitivity. The transformants commonly have a human 1.3-kb DNA that is detected by inter-L1Hs PCR.Citation14) Next, we found that the human 1.3-kb sequence is present in the human YAC clone 923f5, derived from human chromosome 13 q14.3, and that human YAC clone 923f5 complemented the defect of the G258 mutant.Citation15) By sequencing both the 1.3-kb DNA and the right end of YAC clone 923f5, and by database analysis, we found that human YAC clone 923f5 contains the human mannosyltransferase V gene (at that time), hALG11. Then Imperiali et al. found that the S. cerevisiae Alg11 protein has not only mannosyltransferase V activity, but also mannosyltransferase IV activity.Citation3) Hence, we suggested that the G258 mutant has a defect in mALG11.Citation16) That study prompted us to isolate mouse ALG11 (mALG11).
The genes used in the assembly of the lipid-linked oligosaccharide were cloned mainly from S. cerevisiae.Citation1) Using the nucleotide sequence of those yeast genes, corresponding mammalian genes, including human genes, were isolated by homology cloning. We isolated human genes encoding GDP-Man-dependent mannosyltransferases (mannosyltransferase I-V), such as human mannosyltransferase-I (hALG1),Citation17) III (hALG2),Citation18) and V (hALG11) (data not shown), which are homologous to S. cerevisiae ALG1,Citation19) ALG2,Citation20) and ALG11,Citation16) respectively, by tBLASTn search. However, as the genes of mannosyltransferase II and IV had not been identified at that time, we tried to isolate the human mannosyltransferase IV gene.Citation13–15)
In the present study, we cloned mouse mannosyltransferase IV/V gene mALG11 from mouse FM3A cells and studied its transcription in FM3A cells. We found that the cloned mALG11 cDNA complemented the temperature-sensitive defect of the S. cerevisiae ALG11Δ mutant. Moreover, we detected its variant cDNA, and we studied its transcription and compared its expression to those in humans.
Materials and methods
Cell strains and culture
Competent Escherichia coli JM109 was used in the cloning study. pYES3/CT vector-transfected or pYES3/CT-mALG11 cDNA construct-transfected E. coli JM101 cells were grown at 37 °C in LB medium supplemented with 50 μg/mL of ampicillin (LB Amp+). In agar plate culture, LB Amp+ supplemented with 1.5% agar was used.
The budding yeast S. cerevisiae mannosyltransferase IV/V gene disruptant (ALG11Δ strain JCY20-1c: ura3-52 his3-Δ200 trp1-Δ901 lys2-801 suc2-Δ9 leu2-3, 112 alg11Δ::URA3) (ALG11Δ)Citation16) was kindly provided by Prof. Neta Dean (State University of New York at Stonybrook). In most cases, ALG11Δ was grown in YPDA medium (polypeptone 20 g, yeast extract 10 g, glucose 20 g, and adenine sulfate 0.02 g in 1 L of double distilled water) supplemented with 0.5 m KCl (0.5 m KCl-YPDA) at 26 °C, and grown on an agar plate containing 2% agar at 26 °C. In most cases, the mALG11- transfected ALG11Δ mutants (clones 23 and 24) were grown at 26 °C in 0.5 m KCl-YPGA medium that contained galactose in place of the glucose of the 0.5 m KCl-YPDA medium, or 0.5 m KCl-SCD Trp- medium (SCD Trp- medium consisting of 20 g of glucose, 1.7 g of yeast nitrogen base, 5 g of ammonium sulfate, and 2.2 g of dropout supplements without tryptophan, pH 5.6, in 1 L of double-distilled water, supplemented with 0.5 m KCl). For the complementation assay, ALG11Δ and mALG11-transfected ALG11Δ were streaked onto SCD Trp- agar plates containing 2% agar (Wako, Osaka, Japan), SCD Trp+ agar plates supplemented with tryptophan (50 μg/mL), or SCG Trp- agar plates containing galactose in place of the glucose of the SCD Trp- agar plate.
The C3H/He mouse mammary carcinoma cell line FM3A clone F28-7Citation21) was routinely maintained at 33 °C in RPMI1640 medium (RPMI1640 no. 2, Nissui, Tokyo) supplemented with 5% heat-inactivated FBS, streptomycin (40 μg/mL), and penicillin G (40 U/mL), and was grown in a humidified 5% CO2 atmosphere in a CO2 incubator. The human HeLa cells were routinely maintained at 37 °C in a DMEM medium (Nissui, Tokyo) supplemented with 10% heat-inactivated FBS, streptomycin (40 μg/mL), and penicillin G (40 U/mL), and were grown as described above. Cell viability was measured by trypan blue exclusion.
Database search
The Entrez Nucleotide database (http://www.ncbi/nlm/nih.gov/entrez/query) was used to obtain the predicted nucleotide sequences of the cDNA and the genomic DNA of mALG11 and hALG11. A BLAST (BLASTn and tBLASTn) search was done using the NCBI (http://www.ncbi.nlm.nih.gov/BLAST/index.shtml) and the DDBJ/EMBL/GenBank databases (http://blast.ddbj.nig.ac.jp/top-e.html).
A protein search was done using the Swissprot and the TrEMBL database (http://us.expasy.org/sprot/). Sequence alignments were done by ClustalW (http://clustalw.ddbj.nig.ac.jp/) and the ALIGN program.Citation22) Structural prediction of mouse mannosyltransferase IV/V was done by means of the TMHMM Server v. 2.0 Program (http://www.cbs.dtu.dk/services/TMHMM/).
Direct sequencing of mALG11 cDNA
Mouse FM3A cells were grown to 1 × 106 cells/mL as described above. Ten milliliter of the culture was centrifuged at 800 rpm for 5 min at 4 °C. The pellet was washed with 5 mL of PBS- (137 mm NaCl, 2.7 mm KCl, 8 mm Na2HPO4, and 1.5 mm NaH2PO4, pH 7.4). FM3A mRNA was prepared with a QuickPrepTMmicro mRNA Purification Kit (GE Healthcare Japan, Tokyo) following the manufacturer’s instructions. FM3A cDNA was prepared with a Transcriptor First Strand cDNA Synthesis Kit (Roche Diagnostics Japan, Tokyo) by means of an Astec Thermal Cycler (PC-700) (Astec, Fukuoka, Japan) following the manufacturer’s instructions. The absorbance at 260 nm was used to measure the concentrations of DNA and RNA.
Primer3 (http://frodo.wi.mit.edu/primer3/) was used to design the primers for PCR cloning of mALG11 cDNA. PCR primers were designed based on the predicted nucleotide sequence of the ORF, including the non-coding region. The nucleotide sequences of primers were blasted against the DDBJ/EMBL/GenBank database to confirm specificity.
FM3A mALG11 cDNA was prepared by the Expand High Fidelity PCR System (Roche Diagnostics Japan) supplemented with 6% DMSO and 0.15 m betaine, with 0.2 μm M5-TF1 (forward primer, 5′-AAGGTACCGATAAGATGGCGGCGGACACTGGG-3′) and 0.2 μm M5-TR1 (reverse primer, 5′-GAAGCACGGGTTCTCCCATGACCT-3′) (Sigma Genosys Japan, Tokyo).
The PCR product was analyzed by 1% agarose gel electrophoresis, and the expected band having a size of approximately1.5 kb was detected. Then the PCR product was purified by agarose gel electrophoresis and an UltraClean DNA Purification Kit (Funakoshi, Tokyo). The purified product was precipitated with 70% ethanol and resuspended in 10 mm Tris–HCl (pH 7.5) and 1 mm EDTA (TE buffer).
The FM3A mALG11 cDNA obtained was sequenced directly by dye-determined cycle sequencing (ABI prism 3100 Automated Sequencer, Applied Biosystems, Foster City, CA) with gene-specific primers (Sigma Genosys Japan, Tokyo), as follows: forward primers M5-TF1, MMAT5SF-8 (5′-GTTAGGCCATTCTCTTAAACTTGTCC-3′) and MMAT5SF-11 (5′-CGACTCAATGGGATACGCCTTCACAC-3′); reverse primers M5-TR1, MMAT5SR-2 (5′-TACTATGCAGACCGATGGTCACTT-3′), MMAT5SR-7 (5′-CAGCATGTCAGTGCTGATCGT-3′).
Sequencing of individual mALG11 cDNAs derived from subclones of mALG11 cDNA-transfected E. coli JM109
Cloning study and other studies were followed mainly as described in Molecular Cloning.Citation23) FM3A cDNA was prepared as described above. The primers for the PCR cloning of FM3A mALG11 cDNA were as follows: forward primer M5FHIN, 5′-AAAAAAGCTTCCACCGCGGCGGACACTGGCAG-3′ (HindIII restriction sequence underlined and initiation codon boxed) based on the sequence around the 5′-terminal region of the ORF, and reverse primer M5RNOT, 5′-AAAAAAAAGCGGCCGCAGTAAGTAACTTCTCCATGG-3′ (NotI restriction sequence underlined) based on the nucleotide sequence of the 3′-downstream region of the ORF (Sigma Genosys Japan, Tokyo). The sequences of both primers were blasted against the DDBJ/EMBL/GenBank database to confirm their specificity. PCR was done by KOD-Plus-Ver.2 (Toyobo, Osaka, Japan), and mouse FM3A cDNA following the manufacturer’s instructions. The PCR product was analyzed by 1% agarose gel electrophoresis, and the expected band, with a size of approximately 1.5 kb, was detected. The PCR products were purified, precipitated with 70% ethanol, and resuspended in 10 mm Tris–HCl (pH 7.5) and 1 mm EDTA (TE buffer). Then the PCR products were digested with HindIII and NotI. The pYES3/CT vector, with the GAL1 promoter and the TRP1 gene (Invitrogen, Carlsbad, CA), was also digested with HindIII and NotI and treated with calf intestinal alkaline phosphatase (Promega, Madison, WI). Next, 300 ng of the digested PCR product and 100 ng of the digested vector were ligated with 1 unit of T4 ligase (Promega) in 10 μL of the ligation mixture at 25 °C for 3 h. After ligation, a 5-μL aliquot of the ligation mixture was mixed with 100 μL of competent E. coli JM109 (5 × 108 cells). Then the transfected E. coli was treated by heat shock at 42 °C for 50 s. After cooling in ice for 5 min, 895 μL of SOC medium (2% polypeptone, 0.5% yeast extract, 0.05% NaCl, 10 mm MgSO4, 10 mm MgCl2, and 4 mm glucose, pH 7.0) and 1 μL of 5% ampicillin solution was added to the mixture, which was incubated further at 37 °C for 1 h. Aliquots (200 μL) of the culture were plated onto the LB Amp+ plate, and the plates were incubated overnight at 37 °C. Then the ampicillin-resistant transfectants were isolated by single-colony isolation on the LB Amp+ plate three times. pYES3/CT-mALG11 cDNA constructs were isolated from the transfectants and digested with HindIII and NotI. The resulting digested materials were analyzed by 1% agarose gel electrophoresis. The sizes of the amplification product were estimated by λ DNA (Takara, Kyoto, Japan) digested with StyI (Roche Diagnostics Japan, Tokyo). Then the constructs obtained with the 1.5-kb insert were subjected to PCR with vector primers, PYES3-F3 (5′-TAATACGACTCACTATAGGG-3′) and PYES3-R2 (5′-AATGTAAGCGTGGAC-3′). The 1.6-kb PCR product from 24 transfectants was purified by agarose gel electrophoresis and an UltraClean DNA Purification Kit, as described above. The purified PCR products were sequenced by dye-determined cycle sequencing with vector primers (PYES3-F3 and PYES3-R2) and gene-specific primers, as follows: forward primers MMAT5SF-8 and MMAT5SF-11; and reverse primers MMAT5SR-2, MMAT5SR-7, MMAT5SR-14 (5′-TGTGAAGTGAGGATACCGGGAATC-3′), and MMAT5SR-26 (5′-GAATAGAGTCAGCATAGCCTTCT-3′) (Sigma Genosys, Tokyo).
The nucleotide sequence around the initiation codon of the mALG11 genomic DNA
We determined the partial nucleotide sequence of the mALG11 genomic DNA, including the 5′-upstream region of the initiation codon, since there was not sufficient information on the 5′-upstream region of the initiation codon of mALG11 cDNA to design forward PCR primers to isolate the entire ORF of the mALG11 cDNA. Hence, the genomic DNA of mouse FM3A cell was prepared by the standard proteinase K/phenol DNA isolation method.Citation23) The DNA was dissolved in TE buffer to a concentration of 100 ng/μL. To determine the nucleotide sequence around the initiation codon of the mALG11 genomic DNA, we designed PCR primers M5G5T-1F (5′-GAGCCAATAGGATAGAAGAACAGG-3′) and M5G5T-2R (5′-TCAACAGATGGTCAAGTAGCAGA-3′) (Sigma Genosys, Tokyo) to amplify the genomic DNA nucleotide sequence (approximately 300 bp) including the 5′-upstream region of the initiation codon. The PCR reaction mixture contained 100 ng of genomic DNA of mouse FM3A cells, 3 mm MgCl2, 200 μm dNTPs, 200 nm PCR primers, 1.75U of Expand High Fidelity PCR Enzyme, and PCR buffer in 25-μL reaction volumes. The PCR thermal cycling conditions were denaturation for 3 min at 94 °C, 30 cycles of denaturation at 94 °C for 1 min, annealing at 55 °C for 1 min, and extension at 68 °C for 1 min, with a final extension at 68 °C for 5 min.
Studies of the possible extra GTAG insertion in the genomic DNA
To determine the possible extra GTAG insertion in the mouse FM3A genomic DNA of the mannosyltransferase IV/V gene region, primers M5GEIB2-1F (5′-GTCTGTGTCGACCAGCAAAAAT-3′) and M5GEIB2-2R (5′-CCAGGTAAGTTGGGATAACAGC-3′) to amplify the region between exon 2 and intron 2 (product size, approximately 240 bp), and primers M5GEI-B3-3F (5′-AGGGATGGAGTCGGTTTAAATTC-3′) and M5GEI-B3-4R (5′-AATCTTCCACAAGGTAGCGTTTC-3′) to amplify the region between intron 2 and exon 3 (product size, approximately 290 bp) were used. The PCR products were sequenced with each primer to determine whether the GTAG insertion was present in the mouse FM3A genomic DNA of the mALG11 region.
To test the possible extra GTAG insertion in the human HeLa hALG11 cDNA region, primers HM5E1B2-1F (5′-TGTCCTTTGGGGAATCAGAC-3′) and HM5E1B2-2R (5′-TGACATTAACATCGCCGGTA-3′) were designed based on the human ALG11 cDNA sequence,Citation16) and were used to amplify the region between exon 2 and exon 3 (product size, approximately 200 bp). The PCR products were sequenced with each primer to test whether the GTAG insertion was present in the human HeLa genomic DNA of the hALG11 region.
Transfection of the construct possessing expression vector pYES3/CT harboring mouse mannosyltransferase IV/V cDNA (mALG11) into the ALG11Δ mutant
The ALG11Δ mutant was precultured for 2 d in 5 mL of 0.5 m KCl-YPDA medium at 26 °C. Then the culture was inoculated into 6 × 106/mL of fresh medium and incubated to an OD660 of 1.0 (2 × 107/mL). One milliliter of the culture was centrifuged at 5000 rpm for 1 min at 4 °C. The pellet was washed with 1 mL of TE buffer and then with 1 mL of 0.02 m lithium acetate, and resuspended in TE buffer to an OD660 of 4.0. Twenty-five microliters of the ALG11Δ suspension was mixed with 140 μL of one-step buffer (100 μL of 70% PEG7000, 20 μL of 2 m lithium acetate, and 20 μL of 1 m dithiothreitol), 25 μL of carrier single-stranded salmon sperm DNA (1 μg/μL), and a ligated construct with expression vector pYES3/CT harboring genuine FM3A mALG11 cDNA (clones 16, 17, 23, and 24) (1 μg/μL), or the pYES3/CT vector alone (1 μg/μL).
This mixture was incubated for 60 min at 26 °C, heat-shocked at 42 °C for 30 min, and then incubated for 5 min at 26 °C. Then 800 μL of cold TE was added to the incubated mixture, and the suspension was centrifuged at 5000 rpm for 1 min at 4 °C. The yeast pellet was resuspended in 500 μL of 0.85% saline. The suspension was plated in 100-μL units on 0.5 m KCl-SCD Trp- agar plates and incubated at 26 °C for 4–5 d. Individual colonies were picked out with a platinum loom and streaked on 0.5 m KCl-SCD Trp-agar plates. This single colony isolation was repeated three times. To check for the presence of mALG11 cDNA by PCR in the candidate transformants, the following vector primers were used: primers PYES-F3 (5′-TAATACGACTCACTATAGGG-3′) and PYES-R2 (5′-GTCACGCTTACATT-3′). The approximate size of the PCR product was 1.6 kb. In this way, mALG11 clone (16, 17, 23, and 24)-transfected ALG11Δ and mock transfectants having only expression vector pYES3/CT were obtained and further analyzed.
Recovery from temperature-sensitive cell growth of ALG11Δ by transfection of mALG11 cDNA
The ALG11Δ, mock transfected ALG11Δ, and mALG11 (clones 23 and 24)-transfected ALG11Δ were streaked onto an SCD Trp+ agar plate or an SCD Trp- agar plate and an SCG Trp- agar plate, and incubated at 26 °C and at 37 °C, respectively.
Results
Direct sequencing of mALG11 cDNA in mouse FM3A cells
First we performed a tBLASTn search against mouse ESTs with the predicted amino acid sequence of S. cerevisiae mannosyltransferase IV/V, Alg11 as query. Then we depicted a predicted nucleotide sequence of mALG11 cDNA to obtain primer sequences for PCR and direct sequencing, based on the consensus nucleotide sequences of EST clones BY015872, BY026656, BY708687, BB645124, CA324828, BU703635, CK637141, BB821551, BU706477, AV319509, and BQ896685 (Fig. ). We designed PCR primers to amplify the cDNAs of the FM3A cells. Although we designed a promising reverse primer, M5-TR1, based on the nucleotide sequence of the 3′-downstream of the ORF, we did not obtain sufficient information on the nucleotide sequence of the 5′-upstream region of the ORF to design forward primers to amplify the cDNA across both ends of the ORF. Hence at first, we used forward primer M5-TF1, which contained the initiation codon. Using these primers, we amplified the FM3A mALG11 cDNA and determined its nucleotide sequence, as described in “Materials and methods.” We detected double peaks in the electropherogram both from position 276 of the ORF of mALG11 cDNA to the 3′-terminal direction of the plus strand, with forward primers (Fig. ), and from the complementary base of position 279 of the ORF to the 3′-terminal direction of the minus strand, with reverse primers (data not shown). We found that they were derived from the extra tetra nucleotide GTAG insertion at position 276 of the ORF of FM3A mALG11 cDNA, yielding variant cDNA containing 275 bases of the 5′-portion of the mALG11 ORF, the GTAG insertion, and the four resulting base-slid 3′-portion of the mALG11 ORF (described below).
Fig. 2. Typical mouse EST clones homologous to the S. cerevisiae ALG11 gene.
Notes: EST clones BB64514 and BV703635 showed a 130-bp-gap and a 122-bp-gap, respectively.▼ indicates the site of GTAG insertion. Ten of 24 clones had the extra GTAG insertion.
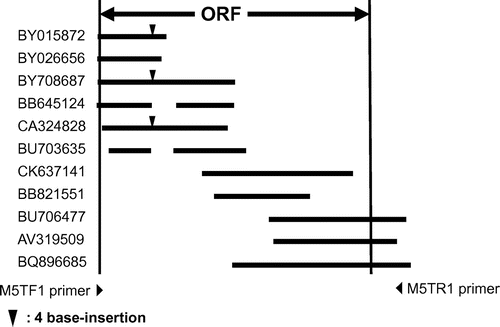
Fig. 3. Electropherogram of the ORF (270–303) of mALG11- and its variant-cDNA.
Note: Black arrow indicates the site of GTAG insertion in the variant cDNA.
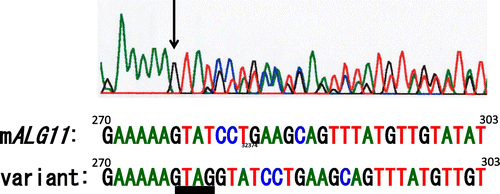
Next we designed a forward primer to amplify the cDNA across both ends of the ORFs of the FM3A mALG11 cDNA. For this purpose, we used the predicted genomic DNA sequence, including the 5′-upstream region of the initiation codon, by BLASTn search, referring to the position of the initiation codon of human ALG11 (hALG11), to design PCR primers to confirm the nucleotide sequence around the initiation codon. Thus, the nucleotide sequence of the FM3A mALG11 cDNA was determined as described above in “Materials and methods,” and deposited at the DDBJ/GenBank/EMBL DNA database (DDBJ/EMBL/GenBank Accession no. AB288237). The nucleotide sequence of the ORF of FM3A mALG11 has a G/C SNP (synonymous mutation) at position 36.
The predicted amino acid sequence of the mouse Alg11 protein was compared to those of the S. cerevisiae, Schizosaccharomyces pombe, Caenorhabditis elegans, and human Alg11 proteins by ClustalW alignment (Fig. ). There were conserved domains among them, including FFHPYCNAGGGGER/KVLWXAL/I/V, PXV/IY/F/WIDS/TMGYA/PF/LT/S, V/IXS/A/TYV/THYPXI/MSXDML, I/VV/IY/FPPC, QXRPEKXH, RXK/ED/E/QD, MXNEHFGIG/S/AVVEXMAA/S, and H/NXSG/AGPXL/M/FDIV (X, not conserved). The alignment score for the mouse Alg11 protein and the human Alg11 protein was 86, and that for the mouse Alg11 protein and the S. cerevisiae Alg11 protein was 31.
Fig. 4. ClustalW sequence alignment of the predicted amino acid sequences of mouse-, human-, C. elegans-, S. pombe-, and S. cerevisiae- mannosyltransferase IV/V.
Symbols: (*) identical residues; (:) conserved substitutions; (.) semi-conserved substitutions. Transmembrane regions are boxed.
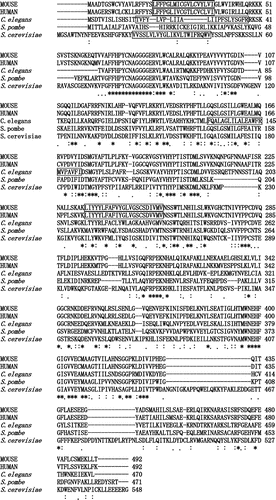
Based on structural prediction by the TMHMM Server v. 2.0 program, the amino and carboxyl terminal regions of the mouse Alg11 protein are oriented in the cytoplasmic site, and there are two membrane-spanning regions between them: from 20L to 42I, and from 234L to 256V (Fig. , boxed). There is a glycosyltransferase group-1 domainCitation24,25) in the carboxyl terminal region that faces the cytoplasmic site.
Also, in mouse Alg11 (mannosyltransferase IV/V) and mouse Alg2 (mannosyltransferase II/III) proteins such as retaining-type mannosyltransferases, which form α-linkages to acceptors with GDP-Man as substrate, there are conserved sequences, such as NEHFGIV/GV/PV/LEC/AM, I/VTGFLA/CE, and AV/HNN/SGGPL/KE/LS/DIV in the glycosyltransferase group-1 domain.
Moreover, by BLASTn search of the entire coding sequence of the mALG11 cDNA, we detected genomic clones AC117665 and AC163439, which were localized in chromosome 8 region A2 (10.92 cM). By comparing the nucleotide sequences of the ORF of mALG11 and its genomic clones, we found that the genomic DNA that encodes the ORF of mALG11 was 7.5 kb in length, that the ORF was separated into four exons by three introns (Figs. and (A)), and that the nucleotide sequences of the splicing sites were consistent with the GT-AG rule.
Fig. 5. Possible mechanism of GTAG insertion in the variant of mALG11 cDNA.
Notes: Partial nucleotide sequences of the exon 2–intron 2 boundary region and the intron 2–exon 3 boundary region of mouse genomic clone AC117665 are shown. Black letters indicate nucleotides in the 3′-terminal region of exon 2 and the 5′-terminal region of exon 3, and gray letters indicate nucleotides in the 5′-terminal region and the 3′-terminal region of intron 2. The variant cDNA contains an extra four nucleotide insertion derived from 5′ end of intron 2 of mALG11 genomic DNA.
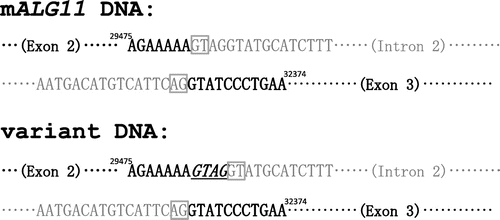
Fig. 6. Comparison of the genomic DNA structure and partial cDNA of sequences of mALG11 (A) and hALG11 (B).
Notes: Left panels show the exons (white boxes: exon 1 (1–44; 44 bp), exon 2 (45–275; 231 bp), exon 3 (276–490; 215 bp), and exon 4 (491–1476; 986 bp)), and introns (black boxes) those of mALG11 ORF and hALG11 ORF, which were obtained in our study and from the DDBJ/EMBL/GenBANK database. The nucleotide sequences (141–350) of the ORF of mALG11 and hALG11 cDNA are shown in the right panel. Underlined italics are non-identical nucleotides between the mALG11 and the hALG11 cDNA. Lines show the boundary region between exon 2 and exon 3 of mouse and of human ALG11 cDNA.
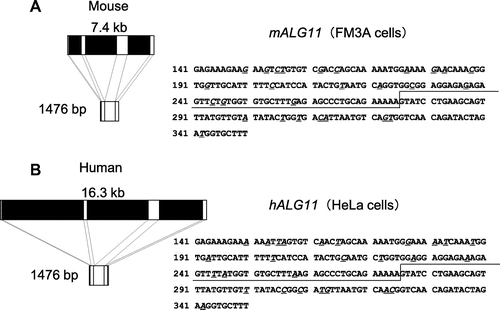
Occurrence of a variant of mALG11
As described above, during the direct sequencing study, we detected double peaks from position 276 of the ORF of mAlg11 cDNA (Figs. and ). In an extensive BLASTn search of mouse ESTs with the nucleotide sequence of S. cerevisiae ALG11, we noticed that there were some variants that had the extra GTAG tetra-nucleotide insertion at position 276 of the ORF of mALG11 cDNA in the ESTs (8 out of 19 clones, 42.1%) (Fig. ). The occurrence of the variant is universal in the mouse irrespective of the strain of mouse, such as C3H/He, C57BL/6, and Chinese domestic mouse Jyg-MC. Moreover, to obtain a probe to express the mALG11 cDNA in ALG11Δ and study the frequency of the GTAG insertion in the mALG11 cDNA, we subcloned the FM3A mALG11 cDNA into the pYES3/CT vector, as described above in “Materials and methods.” In this way, 24 subclones were isolated in E. coli JM101. Among these, 14 clones (58.3%) had the same nucleotide sequence as the FM3A mALG11 cDNA, as described above. However, a further 10 clones (41.7%) had the GTAG insertion in the cloned cDNAs, as described below. Hence, we were able to ascertain that the variant occurs constantly and frequently in the mouse. The nucleotide sequence of the variant was deposited in the DDBJ/GenBank/EMBL DNA database (DDBJ/EMBL/GenBank Accession no. AB288238).
To analyze the cause of the occurrence of the variant, we compared the nucleotide sequences of the genomic DNA and the ORFs of mALG11 cDNA and its variant cDNA. In a BLASTn search of the entire coding sequence of the mALG11 cDNA, we detected genomic clones AC117665 and AC163439, which were localized in chromosome 8 region A2 (10.92 cM). By comparing the nucleotide sequence of the ORF of mALG11 and its genomic clones, we found that the genomic DNA that encodes the ORF of mALG11 was 7.5 kb in length, that the ORF was separated into four exons (exons 1–4) by three introns (introns 1–3) (Fig. (A)), and that the nucleotide sequences of the splicing sites were consistent with the GT-AG rule. The insertion of GTAG in the variant cDNA is localized in the boundary region between exon 2 and exon 3 of the ORF of the cDNA. The nucleotide sequence in the boundary region between exon 2 and exon 3 was … AGAAAAAGTATGC … in the mALG11 cDNA and … AGAAAAAGTAGGTATGC … in its variant cDNA (Fig. ), but there were no extra GTAG insertions in the corresponding site of the genomic DNA of the mALG11 regions of the FM3A cells, the boundary region between exon 2 and intron 2, or the boundary region between intron 2 and exon 3 (Fig. (A)). Taking into consideration the GT-AG rule for splicing, the nucleotide sequence of the genomic DNA and the cDNA of mALG11, and the occurrence of the GTAG sequence before the donor site of intron 2 in the variant cDNA, the GTAG insertion may have derived from a splicing variation from the normal donor site GT of intron 2 to the neighboring GT 4 bases downstream of the normal donor site GT of the intron 2 (from … AGAAAAAAGGTATGC … to … AGAAAAAGTAG
ATGC …) by alternative donor site selectionCitation26,27) (Fig. (B)). As a result, exon 2 gets longer by 4 bases, and this results in a frame shift. The ORF of the variant has termination codon 277TA279G, and this result in the encoding of a peptide containing 92 amino acids. Therefore, the variant peptide does not contain the glycosyltransferase group-1 domain.
Recovery of the cell growth of ALG11Δ due to transformation with mALG11 cDNA
As described above, we cloned the FM3A cDNA, encoding the mALG11 gene region. Fourteen out of 24 cloned cDNAs were genuine mALG11 that did not contain the GTAG insertion. Next, we transfected the cloned genuine FM3A mALG11 cDNA (clones 16, 17, 23, and 24) into ALG11Δ, as described above in “Materials and methods,” and obtained transformants of it with each of the four mALG11 cDNA clones.
Next, we studied the cell growth of mALG11 (clones 23 and 24)-transfected ALG11Δ in comparison with those of the parental ALG11Δ and the mock-transfected ALG11Δ. The ALG11Δ mutant is temperature-sensitive as to cell growth. ALG11Δ grows at 25 °C in spite of a defect in the synthesis of the lipid-linked oligosaccharide and asparagine-linked glycosylation of glycoprotein due to lack of the ALG11 gene, but at 37 °C it does not grow.Citation16) Parental ALG11Δ, mock-transfected ALG11Δ, and mALG11-transfected ALG11Δ (clones 23 and 24) were streaked onto SCD Trp- agar plates and SCG Trp- agar plates, and incubated at 26 or 37 °C. As shown in Fig. , ALG11Δ, mock-transfected ALG11Δ, and mALG11-transfected ALG11Δ grew in SCD Trp+ agar plates at 26 °C (Fig. (A)). This result is constituent with results reported by Cipollo et al.Citation16)
Fig. 7. Recovery of temperature-sensitive growth of the S. cerevisiae ALG11Δ Mutant by Transfection of mALG11 cDNA.
Notes: Parental ALG11Δ, mock-transfected ALG11Δ, and mALG11 (clones 23 and 24)-transfected ALG11Δ were plated on an SCD Trp+ agar plate and grown at 26 °C (A), plated on an SCD Trp- agar plate and grown at 26 °C (B), plated on an SCG Trp- agar plate and grown at 26 °C (C), plated on an SCD Trp- agar plate and grown at 37 °C (D), or plated on an SCG Trp- agar plate and grown at 37 °C (E).
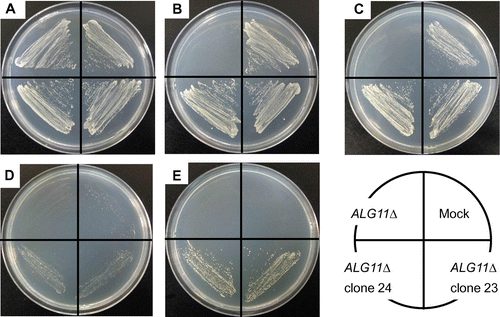
Next ALG11Δ, mock-transfected ALG11Δ, and mALG11-transfected ALG11Δ were plated on SCD Trp- (Fig. (B)) and SCG Trp- agar plates (Fig. (C)), and grown at 26 °C. ALG11Δ did not grow on either plate due to the absence of tryptophan in the medium, but the mock-transfected ALG11Δ and the mALG11-transfected ALG11Δ grew on both plates due to the presence of TRP1 in the pYES3/CT vector. At 37 °C, ALG11Δ and the mock-transfected ALG11Δ did not grow on either agar plate due to the defect in the lipid-linked oligosaccharide synthesis due to a lack of ALG11 (Fig. (D)), while the mALG11-transfected ALG11Δ grew weakly on the SCD Trp- agar plate at 37 °C due to leakiness of the repression of mALG11 by the GAL1 promoter in the presence of glucose (Fig. (D)). However, the mALG11-transfected ALG11Δ grew significantly on an SCG Trp- agar plate at 37 °C (Fig. (E)) due to derepression of mALG11 by the GAL1 promoter in the presence of galactose than that plated on an SCD Trp- agar plate at 37 °C (under the repressed condition) (Fig. (D)). Hence, the defect in cell growth at 37 °C of ALG11Δ was complemented by transfection with mALG11 cDNA.
Comparison of the transcription of ALG11 and its variant between mouse and human
We compared the nucleotide sequence and the transcripts of the genomic DNA of the mALG11 region to those of the human mannosyltransferase IV/V gene (hALG11) region (AL139082). The size of the ORFs of mALG11 and hALG11 is 1476 bp, and the size of the each exon of the ORF is exactly the same in mALG11 and hALG11 (44, 231, 215, and 986 bp, respectively). In other words, the introns, including donor sites and acceptor sites, are present at identical relative positions in both mouse and human. However, the size of the genomic DNA encoding the entire ORF of ALG11 is approximately twofold longer in hALG11 than in mALG11 (7.4 kb in mALG11 and 16.3 kb in hALG11). Hence, the sizes of the introns are different.
We performed a tBLASTn search against the human EST database with the predicted amino acid sequence of S. cerevisiae mannosyltransferase IV/V, Alg11 as query to study whether the hALG11 region would yield a variant similar to that detected in the mouse, but we did not detect any splicing variants with the GTAG insertion in human ESTs showing homology to S. cerevisiae ALG11.
Next, we performed PCR to amplify the exon 2/the exon 3 boundary region of the ORF of hALG11 cDNA in human HeLa cells, but we did not detect any transcripts with the GTAG insertion. Hence, it appears that human does not yield such a splicing variant with the GTAG insertion between exon 2 and exon 3 of the ORF of hALG11 cDNA (data not shown).
Discussion
In the course of the present study, in the mouse EST database, we detected mouse EST clones that were homologous to the predicted S. cerevisiae ALG11 cDNA (Fig. ). We cloned the cDNA of mALG11 from mouse FM3A cells and sequenced its mALG11 cDNA. The deduced amino acid sequence of the mouse Alg11 protein indicated that the mouse Alg11 protein has many conserved domains, ones common to S. cerevisiae-, S. pombe-, C. elegans-, and the human-Alg11 protein (Fig. ). Moreover, we found that cloned mALG11 complemented the defect of the ALG11Δ mutant (Fig. ).
During our tBLASTn search of the mouse EST database with the amino acid sequence of the S. cerevisiae Alg11 protein, we frequently detected the GTAG insertion in mouse ESTs (Fig. ), and found that it was present irrespective of the mouse strain. We also studied its transcription in FM3A cells by cloning each PCR-amplified mALG11 cDNA, and confirmed that the FM3A cells also yielded GTAG-inserted transcripts (Figs. and ). Our cloning and sequencing study of PCR-amplified mALG11 cDNA from FM3A cells indicated that the frequency of the GTAG insertion in mALG11 cDNA was 41.7%. We found that the GTAG insertion was localized between exon 2 and exon 3 of the ORF of the cDNA (Figs. (B) and 6). PCR analysis revealed that there were no GTAG insertions in the mouse genomic DNA (data not shown). Hence, we concluded the GTAG insertion was derived from alternative donor site selection during the splicing event, with the neighboring GT sequence as donor (Fig. (B)). The variant cDNA encoded a peptide that contains the amino-terminal 92-amino acid and lacked a region encoding the glycosyltransferase group-1 domain in the mouse Alg11 protein. Hence, the variant protein might not have any mannosyltransferase activities. Although the alternative donor site selection event might reflect biological significance,Citation26,27) the function of the variant is unknown at present.
The hALG11 has a similar ORF sequence and shows a distribution of exons similar to those of mALG11, and such variants are not found in the human EST database. Moreover, we did not detect any expression of the variant mRNA in human HeLa cells. Therefore, the control mechanism of the transcription of ALG11 region is different as between mouse and human.
Supplemental material
The supplemental material for this paper is available at http://dx.doi.org/10.1080/09168451.2014.890026.
Supplementary Fig. 1 caption
Download MS Word (12 KB)Supplementary Fig. 1
Download MS Power Point (85.5 KB)Acknowledgments
This study was supported in part by grants-in-aid from the Ministry of Education, Culture, Sports, Science, and Technology of Japan, the Kihara Memorial Foundation, the Astellas Foundation for Research on Medicinal Resources (formerly the Fujisawa Foundation on Medical Resources), and by Tokai University by means of a grant-in-aid from the High Technology Research Program (to Y.N.). We would like to thank Prof. Neta Dean (State University of New York at Stony Brook) and Dr Xiao-Dong Gao (Hokkaido University) for providing us the ALG11Δ mutant, Prof. Akinori Ohta (University of Tokyo) for helpful discussion, Prof. Yoshitaka Nagai (Riken, Wako, Japan), Prof. Naoyuki Taniguchi (Osaka University School of Medicine, Osaka, Japan, and Prof. Kunihiko Suzuki and Prof. Akemi Suzuki (Institute of Glycoscience, Tokai University) for their encouragement.
Notes
Abbreviations: FBS, fetal bovine serum; Dol-P, dolichyl phosphate; PBS-, Ca2+, Mg2+ free-phosphate buffered saline; mALG11, the mouse ALG11 gene; hALG11, the human ALG11 gene; ALG11Δ, the Saccharomyces cerevisiae ALG11 gene disruptant.
References
- Burda P, Aebi M. The dolichol pathway of N-linked glycosylation. Biochim. Biophys. Acta. 1999;1426:239–257.10.1016/S0304-4165(98)00127-5
- Schenk B, Fernandez F, Waechter CJ. The in(side) and out(side) of dolichol phosphate biosynthesis and recycling in the endoplasmic reticulum. Glycobiology. 2001;11:61R–70R.10.1093/glycob/11.5.61R
- O’Reilly MK, Zhang G. In vitro evidence for the dual function of Alg2 and Alg11: essential mannosyltransferases in N-linked glycoprotein synthesis. Imperiali B. Biochemistry. 2006;45:9593–9603.10.1021/bi060878o
- Frank CG, Aebi M. ALG9 mannosyltransferase is involved in two different steps of lipid-linked oligosaccharide biosynthesis. Glycobiology. 2005;15:1156–1163.10.1093/glycob/cwj002
- Helenius J, Ng DTW, Marolda CL, Walter P, Valvano MA, Aebi M. Translocation of lipid-linked oligosaccharides across the ER membrane requires Rft1 protein. Nature. 2002;415:447–450.10.1038/415447a
- Nishikawa Y, Yamamoto Y, Kaji K, Mitsui H. Reversible G1 arrest of a human Burkitt Lymphoma cell line (Raji) induced by tunicamycin. Biochem. Biophys. Res. Commun. 1991;97:1296–1303.
- Arnold E, Tanner W. An obligatory role of protein glycosylation in the life cycle of yeast cells. FEBS Lett. 1982;148:49–53.10.1016/0014-5793(82)81240-4
- Huffaker TC, Robbins PW. Temperature-sensitive yeast mutants deficient in asparagine-linked glycosylation. J. Biol. Chem. 1982;257:3203–3210.
- Huffaker TC, Robbins PW. Yeast mutants deficient in protein glycosylation. Proc. Nat. Acad. Sci. USA. 1983;80:7466–7470.10.1073/pnas.80.24.7466
- Nishikawa Y, Mitsui H. Isolation of revertants from a murine temperature-sensitive mutant, deficient in asparagine-linked glycosylation. Proceedings of the 41st Annual Meeting of the Japanese Cancer Association. Vol. 41, 1982; p. 184. Japanese.
- Nishikawa Y, Mitsui H. Isolation of spontaneous revertants from G258, a temperature-sensitive FM3A mutant, deficient in asparagine-linked glycosylation. Proceedings of the 31st Annual Meeting of the Japan Society for Cell Biology. 1982; p. 101; Cell Struct. Funct. 1983;7:412. Japanese.
- Nishikawa Y. Isolation of a temperature-sensitive FM3A mutant deficient in asparagine-linked glycosylation by selecting for resistance to tritiated mannose suicide. J. Cell. Physiol. 1984;119:260–266.10.1002/(ISSN)1097-4652
- Nishikawa Y. An FM3A mutant, G258, with a mutation that affects both cell growth and oligosaccharide-lipid synthesis. Biochim. Biophys. Acta. 1991;1091:135–140.
- Kataoka K, Takahashi T, Ayusawa D, Nishikawa Y. Characterization of a human genomic DNA fragment which rescues defective lipid-linked oligosaccharide synthesis in a mutant G258 cell line isolated from the FM3A mouse mammary carcinoma cell line. Somat. Cell Mol. Genet. 1998;24:235–243.10.1023/B:SCAM.0000007125.41715.8d
- Masuda T, Kataoka K, Moriya M, Kamiguchi H, and Nishikawa Y. Complementation of a defect in the asparagine-linked glycosylation of a mouse FM3A mutant G258 cell line by spheroplast fusion of a human mega YAC clone 923f5. Biosci. Biotechnol., Biochem. 2012;76:108–114.10.1271/bbb.110569
- Cipollo JF, Trimble RB, Chi JH, Yan Q, Dean N. The yeast ALG11 gene specifies addition of the terminal α1,2-Man to the Man5GlcNAc2-PP-dolichol N-glycosylation intermediate formed on the cytosolic side of the endoplasmic reticulum. J. Biol. Chem. 2001;276:21828–21840.10.1074/jbc.M010896200
- Takahashi T, Honda R, Nishikawa Y. Cloning the human cDNA which can complement the defect of the yeast mannosyltransferase I-deficient mutant alg1.Glycobiology. 2000;10:321–327.10.1093/glycob/10.3.321
- Takahashi T, Katoh R, Okutomi S, Suzuki Y, HMori, Takizawa Y, Nishikawa Y, Homo sapiens ALG2 mRNA for asparagine-linked glycosylation 2, complete cds. DDBJ/EMBL/GenBank Accession AB161356;2004.
- Albright CF, Robbins PW. The sequence and transcript heterogeneity of the yeast gene ALG1, an essential mannosyltransferase involved in N-glycosylation. J. Biol. Chem. 1990;265:7042–7049.
- Jackson BJ, Kukuruzinska MA, Robbins P. Biosynthesis of lipid-linked oligosaccharides in Saccharomyces cerevisiae: the alg2 mutation. Glycobiology. 1993;3:357–364.10.1093/glycob/3.4.357
- Koyama H, Kodama H. Adenine phosphoribosyltransferase deficiency in cultured mouse mammary tumor FM3A cells resistant to 4-carbamoylimidazolium 5-olate. Cancer Res. 1982;42:4210–4214.
- Pearson WR, Wood T, Zhang Z, Miller W. Comparison of DNA sequences with protein sequences. Genomics. 1997;46:24–36.
- Sambrook J, Russell DW, editors. Molecular cloning: a laboratory manual. 3rd ed. Cold Spring Harbor, NY: Cold Spring Harbor Laboratory Press; 2001.
- Campbell JE, Davies GJ, Bulone V, Henrissat B. A classification of nucleotide-diphospho-sugar glycosyltransferases based on amino acid sequence similarities. Biochem. J. 1996;326:929–942.
- Kapitonov D, Yu RK. Conserved domains of glycosyltransferases. Glycobiology. 1999;9:961–978.10.1093/glycob/9.10.961
- Black DL. Mechanisms of alternative pre-mesenger RNA splicing. Annu. Rev. Biochem. 2003;72:291–336.10.1146/annurev.biochem.72.121801.161720
- Hiller M, Szafranski K, Sinha R, Huse K, Nikolajewa S, Rosenstiel P, Schreiber A, Platzer M. Assessing the fraction of short-distance tandem splice sites under purifying selection. RNA. 2008;14:616–629.