Abstract
We evaluated in vitro anti-diabetic activities of 497 native plants of Jeju Island (South Korea) by measuring the induction of adipocyte differentiation. Among the plants, Daphniphyllum macropodum fruit extract (DME) had the highest peroxisome proliferator-activated receptor γ (PPARγ) agonist activity and was therefore selected as a potential source of anti-diabetic agents. To elucidate the active components of DME, constituent compounds were purified and their effects on the adipocyte differentiation were studied. Using activity-guided fractionation, four compounds were isolated from DME and their adipogenic effects were evaluated. Among the compounds isolated, 5,7-dihydroxychromone potently induced the differentiation of mouse 3T3-L1 preadipocytes. DME and 5,7-dihydroxychromone increased PPARγ and liver X receptor α (LXRα) mRNA expression levels. To determine whether the adipogenic effects we observed might affect serum glucose levels, we undertook in vivo experiment using streptozotocin-/high-fat diet-induced type 2 diabetes mouse model. DME supplementation reduced serum glucose, total cholesterol, and triacylglycerol levels in diabetes mice. These results suggest that DME may be useful for the prevention and treatment of type 2 diabetes mellitus. Moreover, it was proposed that 5,7-dihydroxychromone isolated from DME is one of the active compounds that may contribute to regulate blood glucose levels.
Graphical Abstract
Daphniphyllum macropodum fruit and its component, 5,7-dihydroxychromone have anti-diabetic properties and can be used in the treatment of diabetes.
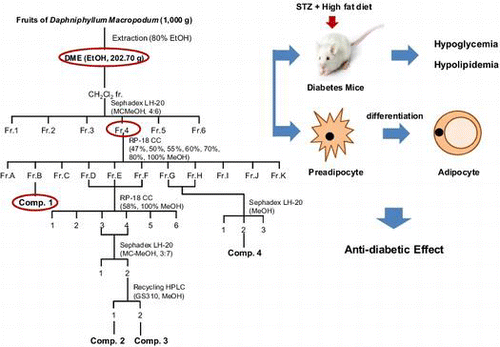
Introduction
Type 2 diabetes mellitus (T2DM) is a common metabolic disorder characterized by the resistance of target tissues to insulin stimulation.Citation1) It may result in severe complications, including renal failure, blindness, slow-healing wounds, and arterial diseases.Citation2) Peroxisome proliferator-activated receptors (PPARs) are members of the nuclear receptor family; they are known to play essential roles in the ligand-mediated activation of transcription factors. PPAR isoforms (α, β/δ, and γ) have been identified as key regulators of glucose absorption, lipid metabolism, proliferation, and cellular differentiation.Citation3,4) Recent clinical trials of the peroxisome proliferator-activated receptor γ (PPARγ) agonist thiazolidinedione have shown a significant improvement of tissue (muscle, adipose tissue, and liver) sensitivity to the effects of insulin, which is used in the treatment of T2DM.Citation3)
There are numerous animal models for the study of T2DM and the ideal pathological models are considered as the developing process and metabolic characteristics of human T2DM.Citation5) Although genetic obese diabetic animals are commonly used, T2DM is a complex polygenic disease and interaction with environment influences, such as high-caloric fat diets. There have been reported that low-dose streptozotocin (STZ) induce mild impairment of insulin secretion and high-fat diet develop insulin resistance.Citation6,7) Therefore, several studies developed animal model by feeding with high-fat diet following low-dose STZ that would closely mimic the metabolic characteristics of human T2DM.Citation6)
Daphniphyllum macropodum is an evergreen shrub that grows in China, Japan, and Korea. It has been shown to be an anthocyanin-rich, biofunctional food that contains various alkaloid compounds.Citation8–13) Its leaf and stalk have been traditionally used in China and Korea as a crude drug for treating wet pleurisy and peritonitis, and also as a diuretic. However, few reports have described the biological activities of D. macropodum, and in particular, none have described any anti-diabetic effects. Therefore, in the present study, we used bioactivity-guided fractionation to identify the main constituent compounds of D. macropodum with anti-diabetic properties. Moreover, we evaluated the anti-diabetic effects of D. macropodum fruit extract (DME) and isolated fractions by using an in vitro adipocyte differentiation assay and an in vivo mouse model of STZ-/high-fat diet-induced T2DM.
Materials and methods
Reagents
Unless otherwise indicated, all chemicals used were purchased from Sigma Chemical Co. (St Louis, MO). AdipoRedTM Assay Reagent was obtained from Lonza Inc. (Walkersville, MD). Dexamethasone (DEX), 3-isobutyl-1-methylxanthine (IBMX), and rosiglitazone were also purchased from Sigma (St Louis, MO).
Preparation of plant extracts
Plants were collected from Jeju Island (Jeju, Korea) and were authenticated on the basis of their microscopic and macroscopic characteristics. Voucher specimens (No. JBR001–JBR08100) were deposited at the herbarium of the Department of Life Science, Gachon University. The dried plant extracts (1000 g) were extracted twice with 80% ethanol by magnetic stirring for 48 h at room temperature, and extracts were then obtained by concentration under reduced pressure at 40 °C by using a rotary evaporator. These extracts were stored at −20 °C until further use.
Fractionation and isolation of D. macropodum
Extracts of the dried fruits of D. macropodum (1000 g) were extracted twice with 80% ethanol (EtOH extract, DME) at room temperature. DME was then concentrated under reduced pressure at 40 °C by using a rotary evaporator and stored at 4 °C until use. This crude extract (DME) was suspended in distilled water and sequentially partitioned with n-hexane, CH2Cl2, EtOAc, and n-BuOH. The CH2Cl2 fraction (Fr.) was subjected to Sephadex LH-20 column chromatography (CC; CH2Cl2–MeOH, 4:6) to yield 6 sub-fractions (Fr.1–Fr.6). Fr.4, which showed comparatively higher adipocyte differentiation activity than the other fractions, was further fractionated by RP-18 CC (47–100% MeOH) to yield 11 sub-fractions (Fr.A–Fr.K). Fr.B was used to produce compound 1. Fr.D, Fr.E, and Fr.F were re-fractionated using RP-18 CC (58 and 100% MeOH) to yield 5 sub-fractions. Fr.DEF3 and 4 were applied to a Sephadex LH-20 MC–MeOH (3:7) column and then purified by recycling High performance liquid chromatography (HPLC; JAIGEL-GS310 column, UV detector 254 nm) to produce compounds 2 and 3. Fr.G and Fr.H were further fractionated using a Sephadex LH-20 (MeOH) column to yield three sub-fractions and then purified to produce compound 4.
5,7-Dihydroxychromone (1): 1H NMR (DMSO-d6, 500 MHz) δ 6.19 (1H, d, J = 2.5 Hz, H-6), 6.26 (1H, d, J = 5.5 Hz, H-3), 6.35 (1H, d, J = 2.5 Hz, H-8), 8.16 (1H, d, J = 6.0 Hz, H-2), 12.68 (1H, s, 5-OH); 13C NMR (DMSO-d6, 125 MHz) δ 94.7 (C-8), 99.7 (C-6), 105.6 (C-10), 111.2 (C-3), 158.1 (C-2), 158.5 (C-9), 162.3 (C-5), 165.0 (C-7), 182.0 (C-4); ESI MS (positive) m/z: 201 [M + Na]+; ESI MS (negative) m/z: 177 [M−H]−.
Chrysoeriol (2): 1H NMR (DMSO-d6, 500 MHz) δ 3.87 (3H, s, 3′-OMe), 6.18 (1H, d, J = 2.0 Hz, H-6), 6.45 (1H, d, J = 2.0 Hz, H-8), 6.85 (1H, s, H-3), 6.90 (1H, d, J = 8.5 Hz, H-5′), 7.56 (2H, m, H-2′, 6′), 12.94 (1H, s, 5-OH); ESI MS (positive) m/z: 323 [M + Na]+; ESI MS (negative) m/z: 299 [M−H]−.
Apigenin (3): 1H NMR (DMSO-d6, 500 MHz) δ 6.19 (1H, d, J = 2.0 Hz, H-6), 6.42 (1H, d, J = 2.0 Hz, H-8), 6.78 (1H, s, H-3), 6.92 (2H, d, J = 9.0 Hz, H-3′, 5′), 7.91 (2H, d, J = 9.0 Hz, H-2′, 6′), 12.94 (1H, s, 5-OH); ESI MS (positive) m/z: 293 [M + Na]+; ESI MS (negative) m/z: 269 [M−H]−.
Chrysoeriol 7-glucoside (4): 1H NMR (DMSO-d6, 500 MHz) δ 3.10–3.70 (6H, m, Glc-H), 3.76 (3H, s, 3′-OMe), 5.01 (1H, d, J = 7.5 Hz, H-1′′), 6.18 (1H, d, J = 2.0 Hz, H-6), 6.50 (1H, d, J = 2.0 Hz, H-8), 6.86 (1H, s, H-3), 7.07 (1H, d, J = 8.5 Hz, H-5′), 7.60 (1H, dd, J = 8.5, 2.0 Hz, H-6′), 7.65 (1H, d, J = 2.0 Hz, H-2′), 12.89 (1H, brs, 5-OH); ESI MS (positive) m/z: 485 [M + Na]+; ESI MS (negative) m/z: 461 [M−H]−.
HPLC analysis for the extract of D. macropodum
Quantitative chromatographic analysis of the major component, 5,7-dihydroxychromone, in the 80% ethanol extract of D. macropodum fruits was performed using the HPLC system that consisted of a Knauer Smartline manager 5000, two Knauer Smartline Pump 1000, and a Knauer Smartline UV detector 2500 equipped with a phenomenex Gemini-NX 5 μ C18 110A column (150 × 4.60 mm). The quantitative determination of an active constituent, 5,7-dihydroxychromone, in the extract was carried out using a gradient elution. The eluent consisted of methanol (A) and 0.2% acetic acid in water (B). The gradient program was used as follows: 0–25 min, linear change from 40% to 70% A in B; 25–30 min, linear change from 70% to 100% A in B; 30–35 min, linear change from 100% to 40% A in B. UV absorption was measured at 295 nm, and flow rate was 1 mL/min.
Serum 5,7-dihydroxychromone levels in the DME-treated mice
Mice received single oral dose (50 mg/kg b.w.) of DME by gavage and blood samples were collected at 0, 1, 2, 4, 6, 12, and 24 h after dosing. UPLC was performed for analysis serum 5,7-dihydroxychromone concentration in the DME-treated mice. A Shimadzu model UPLC equipped with quaternary LC-20AD pumps variable wavelength programmable PDA detector SPD-M20A column oven (Shimadzu), CBM-20A system controller (Shimadzu). The chromatographic separation was achieved with an Eurospher 100-5 NH2 column (4.6× 250mm, 5 mm, Knauer) operating at 35 °C. The mobile phase was 85% acetonitrile with the flow rate of 1 mL/min. The injection volume was 10 μL and elute was analyzed at a wavelength of 210 nm.
Cell culture and adipocyte differentiation
Mouse 3T3-L1 preadipocytes were obtained from the American Type Culture Collection (Manassas, VA) and were grown in Dulbecco’s modified Eagle’s medium (DMEM) (Gibco BRL, NY) supplemented with 10% bovine calf serum (Gibco BRL, NY) and the antibiotics 100 U/mL penicillin and 100 mg/mL streptomycin (Gibco BRL, NY) in an incubator with a humidified atmosphere of 5% CO2 at 37 °C. After reaching confluency, the cells were incubated in DMEM containing 0.5 mM IBMX, 10 μg/mL insulin, 0.25 μM DEX, and 10% FBS. After 2 days, the culture medium was changed to DMEM containing only 10% FBS. The adipocytes were used 8 days after the initiation of differentiation. For adipogenesis assays, 3T3-L1 preadipocytes were treated with test samples for 9 days and then compared to untreated control cells and cells treated with 0.1 μM rosiglitazone. Fresh medium containing 10% FBS with or without samples was replaced every 3 days. Differentiation of adipocytes was measured using AdipoRedTM Assay Reagent (Lonza, Walkersville, MD) according to the manufacturer’s protocol. Briefly, the cells were fixed with 3% formaldehyde in PBS for 1 h at room temperature, washed three times with PBS, and then stained with AdipoRedTM Assay Reagent for 10 min at room temperature. Fluorescence was measured with excitation at 485 nm and emission at 572 nm.
Quantitative RT-PCR analysis
Total RNA was extracted from 3T3-L1 adipocytes by using PureLinkTM RNA Mini Kit (Ambion, USA). Total RNA (1 μg) was reverse transcribed in a 20-μL volume by using oligo (dT) primers with the enzyme and buffer supplied in the PrimeScript II 1st strand cDNA Synthesis kit (Takara, Japan). Quantitative real-time PCR was performed using MX3005P (Stratagene, USA) with the following primers: PPARγ, 5′-CAAGAATACCAAAGTGCGATCAA-3′, 5′-GAGCTGGGTCTTTTCAGAATAATAAG-3′; liver X receptor α (LXRα), 5′-CTCAATGCCTGATGTT TCTCCT-3′, 5′-TCCAACCCTATCCCTAAAGCAA-3′; and β-actin, 5′-GAGACCTTCAACACCCC-3′, 5′-GTGGTGGTGAAGCTGTAGCC-3′. For real-time PCR, SYBR Premix Ex Taq II (Takara, Japan) was used. The final volume of the reaction was 25 μL, containing 2 μL of cDNA template, 12.5 μL of Master Mix, 1 μL of each primer (10 μM stock solution), and 8.5 μL of sterile distilled water. The thermal cycling profile consisted of a pre-incubation step at 95 °C for 10 min, followed by 40 cycles of 95 °C (15 s) and 60 °C (60 s). A relative quantitative evaluation of PPARγ and LXRα gene expression levels was performed using the comparative cycle threshold (CT) method.Citation14)
Animals
Four-week-old male ICR mice were obtained from SLC Inc. (Shizuoka, Japan) and were maintained for 1 week before experiments. They were housed in an air-conditioned animal room with a 12-h light/12-h dark cycle at a temperature of 22 ± 1 °C and humidity of 50 ± 10%. The mice were provided with a laboratory diet and water ad libitum. All experimental protocols involving the use of animals were conducted in accordance with National Institutes of Health guidelines and approved by the Committee on Animal Care, Gachon University.
Induction of T2DM and dyslipidemia
After acclimatization, the mice that had been fasted for 4 h were singly injected with either vehicle or low-dose STZ (100 mg/kg, i.p. in 0.1 M citrate–phosphate buffer, pH 4.5). After 3 weeks, the vehicle-injected mice were randomly divided into 2 groups (n = 6 per group) and fed either a normal diet (ND) or a high-fat/high-cholesterol diet (HFD). The STZ-injected mice with similar hyperglycemia levels were also randomly divided into 3 groups (n = 6 per group) and fed ND (STZ/ND) or HFD (STZ/HFD) for 5 weeks. Subsequently, each group of mice was fed the respective diet and 1 group was fed HFD plus DME (STZ/HFD/DME) for 2 weeks.
Measurements of serum glucose, total cholesterol, and triglyceride levels
At the end of the study, all animals were fasted for 6 h and blood was collected from the abdominal vena cava under anesthesia with diethyl ether. Blood was centrifuged at 3000 × g for 15 min at 4 °C to obtain serum. The serum concentrations of glucose, total cholesterol, and triglyceride were enzymatically measured using commercial kits (EnzyChromTM Cholesterol Assay Kit, EnzyChromTM Triglyceride Assay Kit, and mouse insulin ELISA kit, BioAssay Systems, Hayward, CA, USA.).
Statistical analysis
Results are expressed as mean ± standard error of the mean (SEM). Group differences were determined by one-way analysis of variance followed by a modified t-test with Bonferroni correction for comparisons between individual groups; p < 0.05 was considered significant.
Results and discussion
T2DM is a chronic metabolic disorder that is strongly associated with obesity. It results in insulin resistance and is accompanied by pancreatic beta-cell dysfunction. The present study was designed to screen 497 native plants collected from Jeju Island in South Korea for their anti-diabetic effect. Jeju is known for the rich indigenous plants that can be used for medicinal materials because of its mild oceanic climate and unique ecosystem. We assessed the effects of the substances on the adipocyte differentiation. PPARγ agonists are commonly used as insulin sensitizers for the treatment of T2DM.Citation15,16) PPARγ is predominantly expressed in adipose tissue, where it plays a central role in adipose tissue function, such as cell differentiation, maintenance, and survival through the activation of adipogenic genes.Citation17,18) Among the extracts examined (100 μg/mL), 7 significantly induced adipocyte differentiation and only 4 exhibited no apparent negative effects on cell viability (at concentrations >200 μg/mL) (Table ). Of these extracts, DME showed the most potent effect on the adipocyte differentiation (148 ± 3.1%) (Table ).
Table 1. Effects of selected medicinal plant extracts on 3T3-L1 adipocyte differentiation and cell viability.
Therefore, we attempted to isolate the active compound from DME. The crude EtOH extract (202.70 g) was dissolved in distilled water and partitioned with n-hexane (hexane fr., 13.68 g). Subsequently, the H2O layer was partitioned with dichloromethane (CH2Cl2 fr., 1.22 g). The subsequent consecutive partition step produced 3 fractions: ethyl acetate (EtOAc fr., 1.90 g), n-butanol (n-BuOH fr., 26.78 g), and water (H2O layer, 158.60 g) (Fig. (A)). To obtain the principle components of DME, the fractions were tested for their effects on adipocyte differentiation. Among these, the CH2Cl2 fr. exhibited the most profound effects on the adipocyte differentiation (Fig. (B)). Adipocytes treated with higher concentrations (50 and 100 μg/mL) of the CH2Cl2 fr. showed significant (p < 0.05) differentiation compared to that observed in the untreated control cells (Fig. (B)). Further fractionation and purification of the CH2Cl2 fr. led to the isolation of 6 sub-fractions (Fr.1–Fr.6) (Fig. (A)). We confirmed that the fourth sub-fraction (Fr.4) induced significant (p < 0.05) adipocyte differentiation compared to that induced by the control treatment (Fig. (B)) and thus, the fraction was subjected to further purification. Final purification of Fr.B as an active component was achieved by separation using reverse-phase HPLC to yield compound 1. The structure of compound 1 was identified as 5,7-dihydroxychromone (1)Citation19,20) by analysis of spectroscopic data, including data obtained from 13C NMR spectroscopy, as well as ESI-MS data.
Fig. 1. Effects of crude extract (DME) and solvent fractions on the differentiation of 3T3-L1 preadipocytes.
Notes: (A) Extraction scheme for determining the anti-diabetic properties of DME. (B) Activity-guided fractionation of DME by assessment of the differentiation of 3T3-L1 preadipocytes as the outcome measure (A and B). All values are represented as the means ± SEM (n = 3). *p < 0.05, **p < 0.01, compared to control. Rosi., rosiglitazone (0.1 μM).
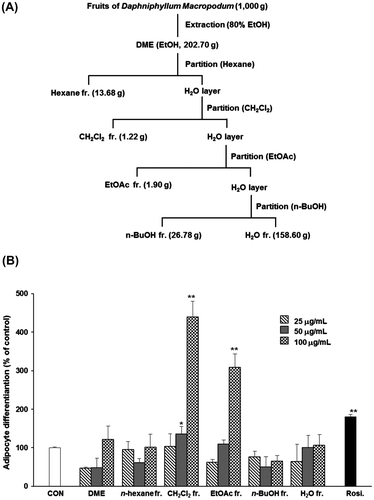
Fig. 2. Effects of sub-fractions from the dichloromethane (CH2Cl2) fraction of DME on the differentiation of 3T3-L1 preadipocytes.
Notes: (A) Separation scheme for determining the anti-diabetic properties of the CH2Cl2 fraction. (B) Activities of the fractions produced at each step are shown. All values are represented as the means ± SEM (n = 3). *p < 0.05, **p < 0.01, compared to control. Rosi., rosiglitazone (0.1 μM).
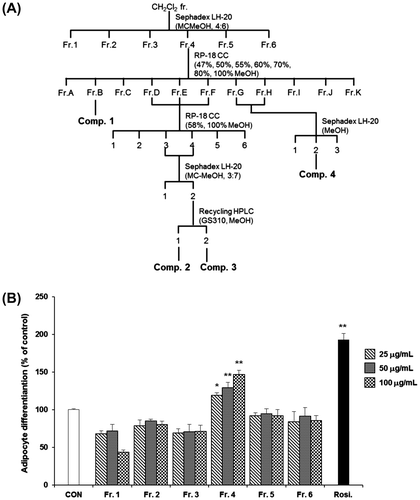
The other compounds isolated from the CH2Cl2 soluble sub-fractions were identified as chrysoeriol (2),Citation19) apigenin (3),Citation21,22) and chrysoeriol 7-glucoside (4)Citation23) by comparison of their spectral data with their respective spectral values reported in the literature. To determine whether these compounds could affect adipocyte differentiation, cells were treated with 1, 5 and 10 μg/mL of each compound. Interestingly, compound 1 (5,7-dihydroxychromone) induced adipocyte differentiation in a concentration-dependent manner (Fig. (A)). However, compounds 2 and 3 from Fr.D, Fr.E, and Fr.F, and compound 4 from Fr.G and Fr.H showed no significant effects on adipocyte differentiation (Fig. (B)–(D)).
Fig. 3. Effects of compounds from DME on the differentiation of 3T3-L1 preadipocytes.
Notes: Cells were treated with various concentrations of compound 1 (A), 2 (B), 3 (C), or 4 (D), and differentiation of adipocytes was measured using AdipoRedTM Assay Reagent. All values are represented as the means ± SEM (n = 3). *p < 0.05, **p < 0.01, compared to control. Rosi., rosiglitazone (0.1 μM).
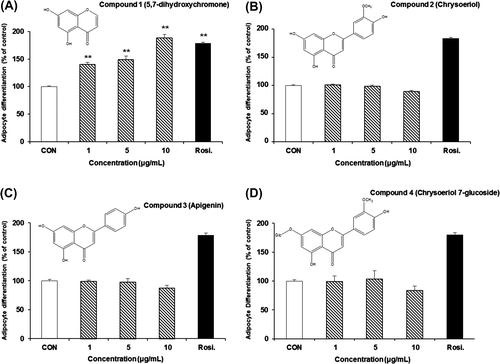
We also assessed whether DME and 5,7-dihydroxychromone could affect PPARγ and LXRα expression in 3T3-L1 cells by using real-time PCR (Fig. ). We decided the experimental concentration range of 5,7-dihydroxychromone such as 1, 5, and 10 μg/mL with references to several in vitro studies.Citation24,25) LXRα is a target gene of the PPARγ, which has been recognized as a potential target for the treatment of T2DM because of its crucial role in regulating glucose metabolism.Citation26) Therefore, the activation of PPARγ/LXRα has been considered as a strategy for treatment of T2DM. Both DME and 5,7-dihydroxychromone increased PPARγ (Fig. (A)) and LXRα (Fig. (B)) mRNA expression levels in a dose-dependent manner, with 5,7-dihydroxychromone showing greater potency for this effect.
Fig. 4. Effects of DME and 5,7-dihydroxychromone on mRNA expressions of (A) PPARγ and (B) LXRα.
Notes: Cells were treated with DME (25, 50, and 100 μg/mL) or 5,7-dihydroxychromone (1, 5, and 10 μg/mL), and total RNA was extracted. Quantitative real-time PCR was performed to measure mRNA levels of PPARγ and LXRα, respectively. A relative quantitative evaluation of PPARγ and LXRα gene expression levels was performed using the comparative CT method. *p < 0.05, **p < 0.01, compared to control.
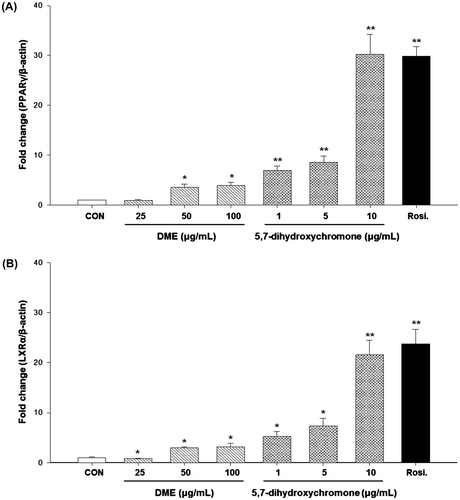
We evaluated the level of an active constituent, 5,7-dihydroxychromone from DME by reversed-phase HPLC analysis with a gradient elution and UV 295 nm detection (Fig. ). Using a standard curve, the concentration of 5,7-dihydroxychromone identified in DME was calculated to be 0.20 μg/mg.
Fig. 5. HPLC chromatograms of (A) 5,7-dihydroxychromone standard and (B) DME sample detected at 295 nm.
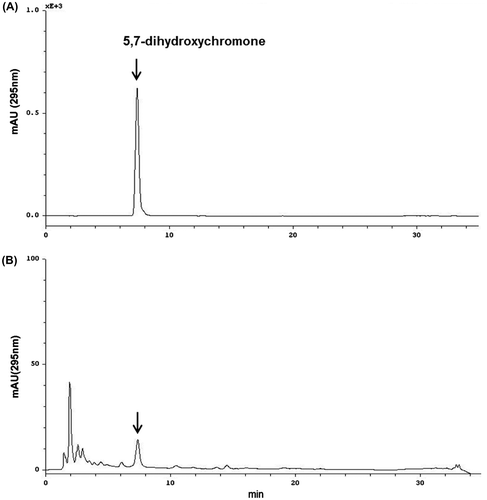
To confirm these in vitro effects of DME, an in vivo study was undertaken using a well-established mouse model of T2DM, which was induced using a low-dose STZ-/high-fat diet. Several lines of research have reported that this model displays insulin resistance, as well as mildly impaired insulin secretion, both of which are distinct characteristics of the metabolic disturbances observed in patients with T2DM compared to the characteristics observed in monogenetic rodent models of diabetes/obesity or dietary-induced obese rats and mice.Citation27,28) Compared to the control mice, the mice with STZ-/HFD-induced T2DM showed elevated blood glucose levels; however, treatment with DME (5, 25, or 50 mg/kg) decreased blood glucose levels in a dose-dependent manner (Table ). Further, compared to the controls, the mice with T2DM showed significantly elevated serum total cholesterol and triglyceride levels. DME (25 or 50 mg/kg) administration also significantly (p < 0.05) reduced serum total cholesterol and triglyceride levels. Furthermore, DME treatment significantly decreased body weight gain in the mice (Table ). There was no significant difference in the total food intake between STZ/HFD mice and DME-treated STZ/HFD mice, and both HFD and STZ/HFD mice consumed significantly (p < 0.05) more food than the control group did. These results demonstrate that DME has recovery effects on the serum levels of cholesterol and triglyceride in STZ-/HFD-induced T2DM.
Table 2. Effects of ethanol extract of D. macropodum fruit (DME) on plasma Glucose, lipid, and body weight in STZ-/HFD-induced mice.
The present results suggest that DME has potent hypoglycemic and hypolipidemic activity in mice with STZ-/HFD-induced T2DM. This discovery of the regulatory agents of glucose levels and lipid metabolism from medicinal plants has been considered more and more important in the treatment of diabetes and other metabolic diseases, since several side effects of many synthetic drugs have been reported.Citation29)
We investigated whether DME treatment resulted in altered expression of PPARγ and LXRα in the liver of STZ-/HFD-induced diabetic mice. As shown in Fig. , the expression levels of PPARγ (Fig. (A)) and LXRα (Fig. (B)) were significantly increased in the high and middle dose DME (25 and 50 mg/kg b.w.) groups when compared with the levels in the control group. In addition, we determined the serum concentration of 5,7-dihydroxychromone after single oral dose of DME 50 mg/kg b.w. in mice. Maximum serum concentrations (96.85 ng/mL) are attained 4 to 6 h after oral dosing (Fig. ).
Fig. 6. Effects of DME on mRNA expressions of (A) PPARγ and (B) LXRα in the liver of STZ-/HFD-induced diabetic mice.
Notes: Quantitative real-time PCR was performed to measure mRNA levels of PPARγ and LXRα, respectively. A relative quantitative evaluation of PPARγ and LXRα gene expression levels was performed using the comparative CT method. *p < 0.05, **p < 0.01, compared to control.
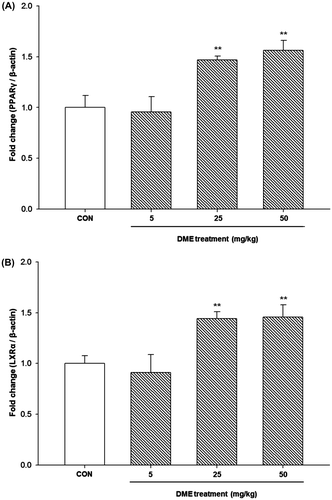
Fig. 7. Serum 5,7-dihydroxychromone concentrations after administration of single dose of DME 50 mg/kg b.w. in mice. Each symbol represents the mean ± SEM (n = 3).
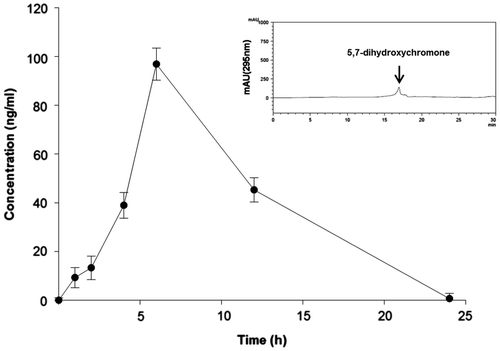
Many studies demonstrated the correlation and efficacy of drug concentrations between in vitro and in vivo. The concentrations of drug used in vitro were much higher than maximal plasma concentrations because the mechanisms in vivo were different than those in vitro.Citation30–32)
In the present study, we found serum level (96.85 ng/mL) of 5,7-dihydroxychromone after oral administration of DME to be much lower than in the concentrations which achieved in vitro effectiveness on adipocyte differentiation. The in vitro results (Fig. (A)) indicated that 5,7-dihydroxychromone at a concentration of 1 μg/mL potently (40.69%) increased adipocyte differentiation and concentrations lower than 1 μg/mL may have the ability to induce adipocyte differentiation. Moreover, we observed that DME administration in vivo and 5,7-dihydroxychromone treatment in vitro dose dependently increased the mRNA expressions of PPARγ and LXRα. In particular, treatment with 1 μg/mL of 5,7-dihydroxychromone induced 7- and 5-fold increases in the levels of PPARγ and LXRα, respectively, in the adipocytes. Although it was higher level of active compound than those after in vivo administration with 50 mg/kg b.w. of DME, this suggests that lower concentrations of 5,7-dihydroxychromone also could affect the expression levels of PPARγ and LXRα. In regard to the anti-diabetic effect of DME, it is explained that 5,7-dihydroxychromone may have the property of lowering blood glucose levels and could be used for therapeutic applications of T2DM.
PPARγ has been identified as a therapeutic target for the prevention and treatment of both insulin resistance and metabolic syndrome. The results of the present study revealed that D. macropodum and its major component, 5,7-dihydroxychromone, efficiently induced adipocyte differentiation, suggesting that it functions as a PPARγ agonist. Although further studies are required to elucidate its mechanism of action, this compound is suspected to be a PPARγ agonist. The results of the present study suggest that 5,7-dihydroxychromone from D. macropodum has potential for clinical applications. Furthermore, these findings suggest that the anti-diabetic effects of DME against chronic STZ/HFD-induced T2DM are attributable to its PPARγ-activating properties.
Funding
This work was supported by the Gachon University research fund of 2012 [GCU-2012-R030].
References
- Rathmann W, Giani G. Diabetes Care. 2004;27:2568–2569.10.2337/diacare.27.10.2568
- Lin Y, Sun Z. J. Endocrinol. 2010;204:1–11.10.1677/JOE-09-0260
- Bermúdez V, Finol F, Parra N, Parra M, Pérez A, Peñaranda L, Vílchez D, Rojas J, Arráiz N, Velasco M. Am. J. Ther. 2010;17:274–283.10.1097/MJT.0b013e3181c08081
- Escher P, Wahli W. Mutat. Res. 2000;448:121–138.10.1016/S0027-5107(99)00231-6
- Luo J, Quan J, Tsai J, Hobensack CK, Sullivan C, Hector R, Reaven GM. Metabolism. 1998;47:663–668.10.1016/S0026-0495(98)90027-0
- Srinivasan K, Viswanad B, Asrat L, Kaul CL, Ramarao P. Pharmacol. Res. 2005;52:313–320.10.1016/j.phrs.2005.05.004
- Reed MJ, Meszaros K, Entes LJ, Claypool MD, Pinkett JG, Gadbois TM, Reaven GM. Metabolism. 2000;49:1390–1394.10.1053/meta.2000.17721
- Cao M, Zhang Y, He H, Li S, Huang S, Chen D, Tang G, Li S, Di Y, Hao X. J. Nat. Prod. 2012;75:1076–1082.10.1021/np200960z
- Chen X, Zhan ZJ, Yue JM. Chem. Biodivers. 2004;1:1513–1518.10.1002/(ISSN)1612-1880
- He X, Wang S, Zhao Y. Zhongguo Zhong Yao Za Zhi. 2011;36:3134–3136.
- Li ZY, Xu HG, Zhao ZZ, Guo YW. J. Asian Nat. Prod. Res. 2009;11:153–158.10.1080/10286020802618837
- Matsuno Y, Okamoto M, Hirasawa Y, Kawahara N, Goda Y, Shiro M, Morita H. J. Nat. Prod. 2007;70:1516–1518.10.1021/np070226g
- Mu SZ, Wang JS, Yang XS, He HP, Li CS, Di YT, Wang Y, Zhang Y, Fang X, Huang LJ, Hao XJ. J. Nat. Prod. 2008;71:564–569.10.1021/np070512s
- Livak kJ, Schmittgen TD. Methods. 2001;25:402–408.10.1006/meth.2001.1262
- Koh YJ, Park BH, Park JH, Han J, Lee IK, Park JW, Koh GY. Exp. Mol. Med. 2009;41:880–895.10.3858/emm.2009.41.12.094
- Powell E, Kuhn P, Xu W. PPAR Res. 2007;2007:53843.
- Anghel SI, Bedu E, Vivier CD, Descombes P, Desvergne B, Wahli W. J. Biol. Chem. 2007;282:29946–29957.10.1074/jbc.M702490200
- Rangwala SM, Lazar MA. Trends Pharmacol. Sci. 2004;25:331–336.10.1016/j.tips.2004.03.012
- Simon A, Chulia AJ, Kaouadji M, Delage C. Phytochemistry. 1994;36:1043–1045.10.1016/S0031-9422(00)90488-6
- Chen HJ, Chung CP, Chiang W, Lin YL. Food Chem. 2011;126:1741–1748.10.1016/j.foodchem.2010.12.074
- Kim CW, Choi KS. Korean J. Pharmacogn. 1993;24:26–31.
- Piao MS, Kim MR, Lee DG, Park Y, Hahm KS, Moon YH, Woo ER. Arch. Pharm. Res. 2003;26:453–457.
- Yuping T, Weiping Z, Fengchang L, Yanfang L, Jinghua W. J. Chin. Pharmaceut. Sci. 2000;9:119–121.
- Liu H, Jiang C, Xiong C, Ruan J. Toxicol. In Vitro. 2012;26:16–23.10.1016/j.tiv.2011.10.002
- Wu PL, Lin FW, Wu TS, Kuoh CS, Lee KH, Lee SJ. Chem. Pharm. Bull. 2004;52:345–349.10.1248/cpb.52.345
- Steffensen KR, Gustafsson JA. Diabetes. 2004;53:S36–S42.10.2337/diabetes.53.2007.S36
- Zhang M, Lv XY, Li J, Xu ZG, Chen L. Exp. Diabetes Res. 2008;2008:704045.
- Zhao S, Chu Y, Zhang C, Lin Y, Xu K, Yang P, Fan J, Liu E. J. Anim. Physiol. Anim. Nutr. (Berl.). 2008;92:105–111.
- Hasani-Ranjbar S, Jouyandeh Z, Abdollahi M. J. Diabetes Metab. Disord. 2013;12:28.10.1186/2251-6581-12-28
- Tian J, Xu Z, Smith JS, Hofherr SE, Barry MA, Byrnes AP. J. Virol. 2009;83:5648–5658.10.1128/JVI.00082-09
- Jia L, Liu X. Curr. Drug Metab. 2007;8:822–829.10.2174/138920007782798207
- Yuan R, Parmelee T, Balian JD, Uppoor RS, Ajayi F, Burnett A, Lesko LJ, Marroum P. Clin. Pharmacol. Ther. 1999;66:9–15.10.1016/S0009-9236(99)70048-2