Abstract
Cysteine residues are absolutely indispensable for the reactions of almost all enzymes involved in the dissimilatory oxidation pathways of reduced inorganic sulfur compounds. Tetrathionate hydrolase from the acidophilic iron- and sulfur-oxidizing bacterium Acidithiobacillus ferrooxidans (Af-Tth) catalyzes tetrathionate hydrolysis to generate elemental sulfur, thiosulfate, and sulfate. Af-Tth is a key enzyme in the dissimilatory sulfur oxidation pathway in this bacterium. Only one cysteine residue (Cys301) has been identified in the deduced amino acid sequence of the Af-Tth gene. In order to clarify the role of the sole cysteine residue, a site-specific mutant enzyme (C301A) was generated. No difference was observed in the retention volumes of the wild-type and mutant Af-Tth enzymes by gel-filtration column chromatography, and surprisingly the enzyme activities measured in the cysteine-deficient and wild-type enzymes were the same. These results suggest that the sole cysteine residue (Cys301) in Af-Tth is involved in neither the tetrathionate hydrolysis reaction nor the subunit assembly. Af-Tth may thus have a novel cysteine-independent reaction mechanism.
Graphical Abstract
The sole cysteine residue (Cys301) in Af-Tth is not involved in neither the tetrathionate hydrolysis reaction nor subunit assembly.
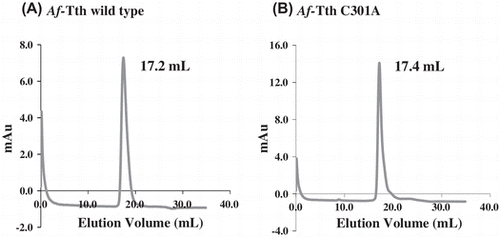
The biological oxidation of reduced inorganic sulfur compounds (RISCs) is one of the most important processes in the environmental sulfur cycle. For example, in aquatic habitats anaerobic photosynthetic purple and green sulfur bacteria are capable of oxidizing hydrogen sulfide generated by anaerobic sulfate-reducing micro-organisms.Citation1) Elemental sulfur from anoxygenic photosynthesis is further oxidized to sulfate by these bacterial species or aerobic sulfur oxidizers such as members of the Paracoccus and Starkeya genera.Citation2,3) RISCs are abundant in acidic environments such as volcanic and geothermal areas and also in acid mine drainage due to human activities. Chemolithoautotrophic RISCs’ oxidizers play a pivotal role as primary producers in such acidic environments. The biological oxidation of RISCs is thus important not only in acidic ecosystems but also in applied microbiology fields such as biomining.Citation4–6)
At least two major dissimilatory RISCs oxidation pathways have been proposed, and various enzymes involved in RISCs oxidation metabolism have been identified and characterized.Citation2,3,7–10) One of the most common pathways for RISCs’ oxidation is the sulfur-oxidizing (Sox) system which is found in both phototrophic and chemolithotrophic sulfur-oxidizing bacteria.Citation2,11,12) This pathway has been extensively investigated in the neutrophilic thiosulfate-oxidizing bacterium Paracoccus pantotrophus.Citation11) The multi-enzyme system required for thiosulfate oxidation in the Sox pathway consists of the SoxXA, SoxB, and the SoxYZ complex.Citation13,14) The C-terminal cysteine residue of SoxY plays the crucial role of substrate carrier. Thiosulfate is oxidized by SoxXA to generate SoxY-thiocysteine-S-sulfate in the first step of the pathway. The thiocysteine-S-sulfate residue of SoxY is then hydrolyzed by SoxB to yield SoxY-bound S-thiocysteine residue, sulfate, and 2H+.Citation15) Subsequently, SoxCD oxidizes the S-thiocysteine residue to SoxY-cysteine-S-sulfate, which is then hydrolyzed by SoxB to remove sulfate from SoxY-cysteine-S-sulfate.Citation15) The SoxCD complex is not required for sulfite oxidationCitation11); however, the cysteine residues in SoxXA and SoxCD are necessary for each reaction.Citation2,11)
A second dissimilatory RISCs oxidation pathway, the S4 intermediate (S4I) pathway, involves the thiosulfate dehydrogenase (Tsd) and thiosulfate:quinone oxidoreductase (TQO) enzymes.Citation3) Both the enzymes catalyze the oxidation of the two molecules of thiosulfate to generate tetrathionate. Tsd gene homologues are widespread in α-, β-, γ-, δ-, and ε-Proteobacteria.Citation16) The gene encoding Tsd in the purple sulfur bacterium Allochromatium vinosum (tsdA) has been identified and characterized. A mutant Tsd protein in which a conserved cysteine residue (Cys123 in Al. vinosum TsdA) was replaced with glycine was completely inactive, suggesting that Cys123 is essential to the enzyme’s activity.Citation15) Recently, we have characterized unique Tsd from the iron- and sulfur-oxidizing bacterium Acidithiobacillus ferrooxidans (Af-Tsd).Citation17) The primary structure of Af-Tsd encoded by AFE_0042 is different from that of Al. vinosum Tsd. A sole cysteine residue in Af-Tsd is conserved in a multiple alignment of Tsd homologues.Citation17) This cysteine residue may be necessary for the activity. TQO, which consists of two subunits, has been purified from the thermoacidophilic archaeon Acidianus ambivalens, in which the enzyme is encoded by the doxDA genes.Citation8) Both subunit genes have been detected as a single open reading frame (ORF) in the genome of At. ferrooxidans. A multiple sequence alignment of the combined Ad. ambivalens DoxD and DoxA amino acid sequences with homologues from other species showed a single conserved cysteine residue which is a putative thiosulfate binding site.Citation8) Sulfur oxygenase reductases (SORs) catalyze oxygen-dependent sulfur disproportionation, which is the initial step of sulfur metabolism in some thermophilic archaea and bacteria. X-ray crystallography and site-directed mutagenesis experiments carried out on SOR from Ad. ambivalens reveal that Cys31 forms part of the core active site and, along with Cys101 and Cys104, is required for optimum enzyme activity.Citation9,18,19) Sulfide:quinone oxidoreductase (SQR) is involved in sulfide metabolism in many phototrophic and chemolithotrophic prokaryotes, and the crystal structure of this enzyme from At. ferrooxidans was recently determined.Citation10) Two cysteine residues (Cys160 and Cys356) in the SQR catalytic center form a branched polysulfide bridge between the substrate (S2–) and the FAD cofactor. The third cysteine persulfide residue (Cys128-S-S–) attacks the Cys160 residue to release the polysulfur (S8) reaction products.Citation10) All the above-mentioned enzymes catalyze the core reactions in the microbial dissimilatory sulfur metabolism, and cysteine residues are vital for the reactions in almost all of these enzymes.
Tetrathionate hydrolase (4THase) is one of the unique enzymes involved in S4I pathways in acidophilic prokaryotes.Citation3) In addition to utilizing tetrathionate as an energy substrate, 4THase functions to hydrolyze tetrathionate which is the product of Tsd or TQO reactions to regenerate thiosulfate in this pathway. The enzyme has been characterized in the genus Acidithiobacillus (At. ferrooxidans, At. thiooxidans, and At. caldus),Citation20–23) in Acidiphilium acidophilum,Citation24) and in the thermoacidophilic archaeon Ad. ambivalens.Citation25) 4THase of At. ferrooxidans (Af-Tth) catalyzes tetrathionate hydrolysis to generate elemental sulfur, thiosulfate, and sulfate.Citation23) Although 4THase is synthesized in only tetrathionate-grown cells of At. caldus and Ad. ambivalens,Citation22,25) Af-Tth is synthesized in both tetrathionate- and sulfur-grown cells.Citation23) Af-Tth thus seems to play a crucial role in the S4I pathway in At. ferrooxidans. Only one cysteine residue (Cys301) was identified in the deduced amino acid sequence from the Af-tth gene. Since cysteine residues are essential for almost all enzymes involved in RISCs oxidation pathways, the aim of this study is to investigate the role of the sole cysteine residue (Cys301) of Af-Tth.
Materials and methods
Bacterial strains, plasmids, and growth media
At. ferrooxidans (ATCC23270) for genomic DNA isolation was aerobically grown on mineral salt medium (pH 3.0) supplemented with trace elements and 5 mM K2S4O6 as an energy source.Citation23) The cloning vector pUC18 and host cells Escherichia coli DH5α (Invitrogen, Carlsbad, CA, USA) were used for DNA manipulation. E. coli cells were grown in Luria-Bertani (LB) medium supplemented with ampicillin (50 μg mL−1). The expression vector pET21a and host cells E. coli BL21 Star™(DE3) (Novagen, Madison, WI, USA) were utilized to obtain the recombinant proteins.
Construction of the C301A Af-Tth mutant expression plasmid
In order to subclone the truncated Af-tth gene without the region of putative signal peptide, pET4TH Citation23) was digested with XbaI and EcoRI, and the excised sequence was ligated into the pUC18 vector digested with the same restriction enzymes. E. coli DH5α cells were transformed with this ligation mixture. A single white colony was picked and the plasmid was isolated from the cells to confirm the insertion of the Af-tth gene from the pET4TH vector. The pUC18 plasmid containing the Af-tth gene was named pUC4TH. Site-directed mutagenesis was performed using the KOD -Plus- Mutagenesis Kit (Toyobo, Osaka, Japan) utilizing the overlap extension method. Two complementary oligonucleotide primers were designed (primer 1: 5′-CTATCAAGCCATATCTTTATGCCCTGAATGAATCCAACG-3′; complementary primer 2: 5′-CGTTGGATTCATTCAGGGCATAAAGATATGGCTTGATAG-3′), and the codon for Cys301 (TGT) was replaced with an alanine codon (GCC; underlined). Experiments were carried out in accordance with the standard protocol provided by manufacturer. The introduction of the correct point mutation and the absence of unintended mutations were confirmed by DNA sequencing. After confirmation of the DNA sequence, the plasmid containing the point mutation, named pUC4TH-C301A, was digested with XbaI and EcoRI. The insert containing the mutation was inserted into a pET21a expression vector using the same restriction enzyme sites.
Protein expression and refolding
The pET4TH expression vector was constructed and introduced into E. coli BL21 Star™(DE3) cells to obtain recombinant Af-Tth as previously described.Citation23) E. coli BL21 Star™(DE3) cells harboring the pET4TH plasmid were cultured in modified Terrific Broth (TB) medium supplemented with 50 μg mL−1 ampicillin at 37 °C to an OD660 nm of 1.0. The expression of Af-Tth in pET4TH was induced by the addition of 1 mM isopropyl β-D-1-thiogalactopyranoside (IPTG) to the culture, followed by incubation at 20 °C for 36 h. The recombinant protein was produced in inclusion bodies in an inactive form. Inclusion bodies were collected by centrifugation at 10,000 × g and washed three times with 100 mM potassium phosphate buffer (KPB) (pH 7.0) containing 4% (v/v) Triton X-100, after which the residual detergent was removed by washing with sterilized, distilled water. The refolding protocol used to solubilize and reactivate Af-Tth has previously been described in detail.Citation26) Purity of the proteins was confirmed by sodium dodecyl sulfate-polyacrylamide gel electrophoresis (SDS-PAGE). The 4THase activity of Af-Tth was measured using a modified cyanolysis assay,Citation23) and the protein concentration of purified Af-Tth was determined by the Bradford method using bovine serum albumin as a standard. One unit (U) of the activity was defined as the amount of enzyme required for the hydrolysis of 1 μmol tetrathionate per min. The expression, refolding, and characterization of the C301A mutant Af-Tth were performed as described above for the wild-type Af-Tth.
Determination of the native molecular mass of wild-type and mutant Af-Tth
The native molecular mass of Af-Tth was determined by gel-filtration column chromatography using an ÄKTA prime plus apparatus (GE Healthcare, Buckinghamshire, England). The purified enzyme (15 ± 6 μg) was applied onto TSKgel G3000SW gel-filtration column (Tosoh, Tokyo, Japan) equilibrated with 20 mM β-alanine sulfate buffer (pH 4.0) containing 0.4 M ammonium sulfate and 2 mM dithiothreitol. The enzyme was eluted with the same buffer at a flow rate of 0.2 mL min−1. Proteins were monitored by absorbance at 280 nm. The protein molecular mass was calculated by this column chromatography using aldolase (158 kDa), albumin (67 kDa), ovalbumin (43 kDa), and chymotrypsinogen (25 kDa) as size standards. The column volume (Vc) was determined with the elution volume of cyanocobalamin (VB12, MW = 1355).
Circular dichroism
The circular dichroism (CD) spectra were measured on a J-805 spectropolarimeter (JASCO, Tokyo, Japan) using a quartz cell of 1 mm optical path. Because high concentration of salts (e.g. 400 mM ammonium sulfate) had prevented the analysis on CD, both refolded Af-Tth proteins (wild-type and C301A variant) were dialyzed against 20 mM β-alanine sulfate buffer (pH 4.0) containing 1.2% (w/v) n-dodecyl-β-D-maltopyranoside to remove ammonium sulfate. Far-UV CD spectra of the proteins were measured at room temperature.
Results and discussion
Comparison of deduced amino acid sequences of 4THase genes from three species
To date, three 4THase genes have been experimentally identified in At. ferrooxidans, At. caldus, and Ad. ambivalens.Citation23,25,27) Fig. shows the alignment of the primary structure of the three 4THases excluding their putative signal peptides. The primary structure of both 4THases from the Acidithiobacillus species shares 59.1% (276/467) identity and 75.0% (350/467) similarity, while Af-Tth shares 36% (168/467) identity and 54.4% (254/467) similarity with the mature 4THase from Ad. ambivalens. In contrast to 12 cysteine residues in the enzyme from Ad. ambivalens, only one cysteine residue was identified in the 4THases from At. ferrooxidans (Cys301) and At. caldus (Cys217). Because cysteine residues are indispensable for the activities of the enzymes involved in dissimilatory sulfur oxidation pathways such as Sox system, Tsd, SQR, and SOR, the cysteine residues were normally conserved in each enzyme among different sulfur-oxidizers. However, the cysteine residues were not conserved between the three 4THases. This suggested that the sole cysteine residue may not involve in the catalytic center and it implied the possibility of a new cysteine-independent reaction in 4THase. Therefore, it is of interest to investigate the role of the sole cysteine residue (Cys301) in Af-Tth.
Fig. 1. Alignment of deduced amino acid sequence of 4THases.
Notes: Putative signal peptides are omitted in each sequence. Asterisks indicate conserved amino acid residues between the three 4THases, and colons and periods indicate strongly and weakly similar residues between the sequences, respectively. Cysteine residues are indicated in reverse. Af-Tth: 4THase from At. ferrooxidans; Ac-TetH: 4THase from At. caldus; and Aam-TTH: 4THase from Ad. ambivalens.
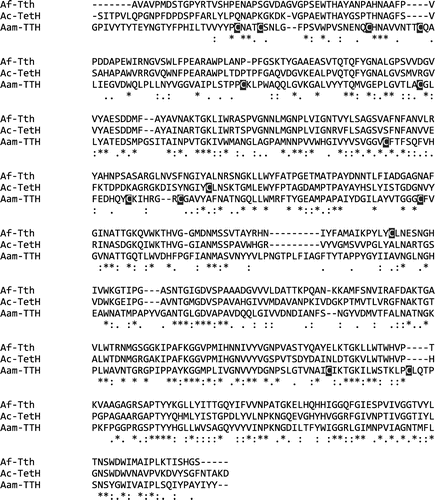
Characterization of C301A mutant Af-Tth enzyme
In order to clarify the role of the sole cysteine residue (Cys301) in Af-Tth, a mutant gene was engineered in which Cys301 was replaced with alanine. The mutated gene was subsequently expressed in E. coli BL21 Star™ (DE3) cell, and the C301A mutant protein was successfully solubilized by refolding. SDS-PAGE analysis showed that no significant difference was observed in the recombinant expression and during the refolding treatments between the two proteins (Fig. ). 4THase activity was detected in the C301A mutant protein (2.6 ± 0.48 U mg−1) at the same level as in the wild-type Af-Tth enzyme (2.5 ± 0.18 U mg−1), indicating that the sole cysteine residue is not involved in the activity of the enzyme. As mentioned in the introduction, cysteine residues are indispensable to the reactions of almost all enzymes involved in dissimilatory RISCs oxidation pathways. One exception has been reported: SoxB from the thermophilic bacterium Thermus thermophilus has cysteine-independent reaction mechanism as determined by X-ray crystallographic analysis.Citation15) The reaction catalyzed by SoxB in the bacterial Sox sulfur oxidation pathway is the hydrolysis of SoxY-thiocysteine-S-sulfate and/or SoxY-cysteine-S-sulfate to generate a sulfate ion. Two manganese ions (Mn2+) chelated by His and Asp residues, Trp and Arg residues in the catalytic center of SoxB interact with the substrate of the C-terminal peptide of SoxY bearing the cysteine-conjugated sulfonate. The nucleophilic hydroxyl ion then attacks the sulfone sulfur atom in the SN2 reaction. The final step of the reaction is the replacement of the sulfate ion product in the catalytic center of SoxB with a hydroxyl ion. A sequence alignment of SoxB and Af-Tth showed that the amino acid residues corresponding to the catalytic center of SoxB were not conserved in Af-Tth (data not shown), suggesting that Af-Tth uses a different mechanism to break sulfur–sulfur bonds in tetrathionate hydrolysis. Furthermore, the reaction of SoxB is not efficient under acidic conditions due to the importance of hydroxyl ions in the reaction. Hydroxyl ions at pH 3.0, the optimum pH of the Af-Tth reaction, are far less abundant (10−4) than at neutral pH.
Fig. 2. SDS-PAGE of wild-type and mutant Af-Tth.
Notes: Lanes 1 and 2, soluble and insoluble fractions of E. coli BL21 Star™(DE3) harboring pET4TH or expression plasmid encoding C301A variant after induction by IPTG, respectively. Lane 3, the fraction of inclusion bodies after washing with Triton X-100. Lane 4, soluble recombinant proteins after refolding treatment. Bars A and B indicate wild-type and C301A variant, respectively. Lane M, molecular mass markers. Molecular masses (kDa) are indicated on the left of the panel.
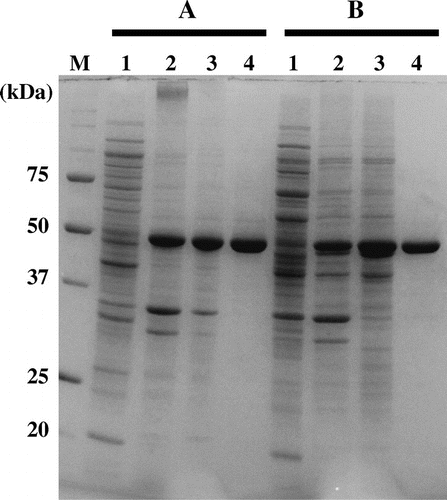
Wild-type Af-Tth has been characterized as a homodimer with a molecular mass of 93 kDa.Citation23) The potential role of Cys301 in the dimerization of the protein was investigated by subjecting the Af-Tth C301A mutant protein to gel-filtration column chromatography at pH 4.0. A single protein peak was observed on the chromatograms of the C301A mutant and wild-type enzyme (Fig. ). The molecular mass of each protein peak was estimated to be approximately 100 kDa (Fig. ). This suggested that the dimerization between protein subunits does not occur via a disulfide bond involving Cys301. Various interactions besides disulfide bonding by cysteine residues may facilitate dimerization. 4THase from Ad. ambivalens has been reported to exist in both dimer and monomer forms, and enzyme activity has been detected in both forms,Citation25) indicating that subunit association is not essential for enzyme activity. Although 12 cysteine residues have been identified in the 4THase from Ad. ambivalens, these residues may not be involved in dimerization but rather in disulfide bond formation within monomer subunits for thermostability.
Fig. 3. Gel-filtration column chromatography of wild-type and mutant Af-Tth.
Notes: The refolded active Af-Tth wild-type (A) and C301A mutant (B) proteins were loaded onto TSKgel G3000SW chromatography columns. Elution volumes of peaks are indicated for each protein.
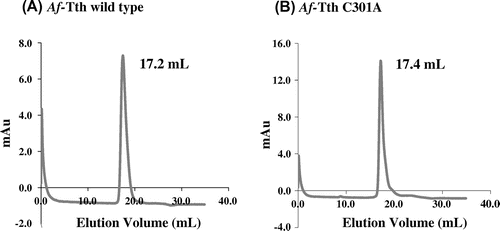
Fig. 4. Molecular mass determination of wild-type and mutant Af-Tth.
Notes: TSKgel G3000SW gel-filtration column was calibrated by using standard molecular mass markers (closed circle): 1, aldolase (158 kDa); 2, albumin (67 kDa); 3, ovalbumin (43 kDa); and 4, chymotrypsinogen (25 kDa). The molecular masses of Af-Tth wild-type (closed square) and C301A mutant (open diamond) proteins are determined to be 100 kDa. Ve and Vc represent elution volume of each protein and column volume, respectively.
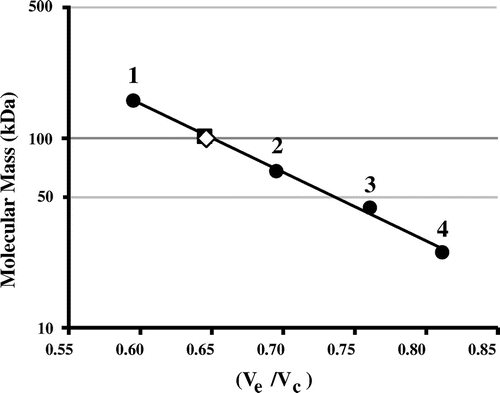
Furthermore, far-UV CD spectra of the both proteins were virtually identical, suggesting that the C301A mutation did not induce significant structural alterations (data not shown).
Conclusions
The C301A mutant Af-Tth was, like the wild-type protein, readily solubilized by acidic refolding treatment. In spite of cysteine deficiency, the 4THase activity of the C301A mutant was measured at levels comparable to those of the wild-type Af-Tth. A single peak corresponding to 100 kDa was observed for both the C301A mutant protein and wild-type Af-Tth by gel-filtration chromatography (Fig. ). These findings suggest that the sole cysteine residue (Cys301) in Af-Tth is not involved in either the catalytic reaction or dimerization. Because cysteine residues are essential in the reactions of all the enzymes involved in dissimilatory sulfur oxidation metabolism with the exception of SoxB, the cysteine-independent reaction of Af-Tth is unusual and may be a novel reaction mechanism. It is thus interesting to further investigate the reaction mechanism of a tetrathionate hydrolysis in detail. We have successfully produced crystals of recombinant Af-Tth,Citation28) which, in future studies, will allow X-ray crystallographic analysis to be carried out to reveal the 3D structure, active site, and reaction mechanism of this unique enzyme.
Acknowledgments
This work was financially supported by the Japan Society for the Promotion of Science (JSPS) [grant number 26450482] and the Japan Oil, Gas and Metals National Corporation (JOGMEC).
References
- Frigaard NU, Dahl C. Sulfur metabolism in phototrophic sulfur bacteria. Adv. Microb. Physiol. 2009;54:103–200.
- Friedrich CG, Bardischewsky F, Rother D, Quentmeier A, Fischer J. Prokaryotic sulfur oxidation. Curr. Opin. Microbiol. 2005;8:253–259.10.1016/j.mib.2005.04.005
- Ghosh W, Dam B. Biochemistry and molecular biology of lithotrophic sulfur oxidation by taxonomically and ecologically diverse bacteria and archaea. FEMS Microbiol. Rev. 2009;33:999–1043.10.1111/fmr.2009.33.issue-6
- Rawlings DE. Characteristics and adaptability of iron- and sulfur-oxidizing microorganisms used for the recovery of metals from minerals and their concentrates. Microb. Cell Fact. 2005;4:13.10.1186/1475-2859-4-13
- Rawlings DE, Johnson DB. The microbiology of biomining: development and optimization of mineral-oxidizing microbial consortia. Microbiology. 2007;153:315–324.10.1099/mic.0.2006/001206-0
- Dopson M, Johnson DB. Biodiversity, metabolism and applications of acidophilic sulfur-metabolizing microorganisms. Environ. Microbiol. 2012;14:2620–2631.10.1111/emi.2012.14.issue-10
- Kelly DP, Shergill JK, Lu WP, Wood AP. Oxidative metabolism of inorganic sulfur compounds by bacteria. Antonie Van Leeuwenhoek. 1997;71:95–107.10.1023/A:1000135707181
- Müller FH, Bandeiras TM, Urich T, Teixeira M, Gomes CM, Kletzin A. Coupling of the pathway of sulphur oxidation to dioxygen reduction: characterization of a novel membrane-bound thiosulphate:quinone oxidoreductase. Mol. Microbiol. 2004;53:1147–1160.10.1111/j.1365-2958.2004.04193.x
- Urich T, Coelho R, Kletzin A, Frazao C. The sulfur oxygenase reductase from Acidianus ambivalens is an icosatetramer as shown by crystallization and Patterson analysis. Biochim. Biophys. Acta. 2005;1747:267–270.10.1016/j.bbapap.2004.11.015
- Cherney MM, Zhang Y, Solomonson M, Weiner JH, James MN. Crystal structure of sulfide:quinone oxidoreductase from Acidithiobacillus ferrooxidans: insights into sulfidotrophic respiration and detoxification. J. Mol. Biol. 2010;398:292–305.10.1016/j.jmb.2010.03.018
- Friedrich CG, Rother D, Bardischewsky F, Quentmeier A, Fischer J. Oxidation of reduced inorganic sulfur compounds by bacteria: emergence of a common mechanism? Appl. Environ. Microbiol. 2001;67:2873–2882.10.1128/AEM.67.7.2873-2882.2001
- Welte C, Hafner S, Kratzer C, Quentmeier A, Friedrich CG, Dahl C. Interaction between Sox proteins of two physiologically distinct bacteria and a new protein involved in thiosulfate oxidation. FEBS Lett. 2009;583:1281–1286.10.1016/j.febslet.2009.03.020
- Friedrich CG, Quentmeier A, Bardischewsky F, Rother D, Kraft R, Kostka S, Prinz H. Novel genes coding for lithotrophic sulfur oxidation of Paracoccus pantotrophus GB17. J. Bacteriol. 2000;182:4677–4687.10.1128/JB.182.17.4677-4687.2000
- Rother D, Henrich HJ, Quentmeier A, Bardischewsky F, Friedrich CG. Novel genes of the sox gene cluster, mutagenesis of the flavoprotein SoxF, and evidence for a general sulfur-oxidizing system in Paracoccus pantotrophus GB17. J. Bacteriol. 2001;183:4499–4508.10.1128/JB.183.15.4499-4508.2001
- Sauve V, Roversi P, Leath KJ, Garman EF, Antrobus R, Lea SM, Berks BC. Mechanism for the hydrolysis of a sulfur-sulfur bond based on the crystal structure of the thiosulfohydrolase SoxB. J. Biol. Chem. 2009;284:21707–21718.10.1074/jbc.M109.002709
- Denkmann K, Grein F, Zigann R, Siemen A, Bergmann J, van Helmont S, Nicolai A, Pereira IA, Dahl C. Thiosulfate dehydrogenase: a widespread unusual acidophilic c-type cytochrome. Environ. Microbiol. 2012;14:2673–2688.10.1111/emi.2012.14.issue-10
- Kikumoto M, Nogami S, Kanao T, Takada J, Kamimura K. Tetrathionate-forming thiosulfate dehydrogenase from the acidophilic, chemolithoautotrophic bacterium Acidithiobacillus ferrooxidans. Appl. Environ. Microbiol. 2013;79:113–120.10.1128/AEM.02251-12
- Urich T, Gomes CM, Kletzin A, Frazao C. X-ray Structure of a self- compartmentalizing sulfur cycle metalloenzyme. Science. 2006;311:996–1000.10.1126/science.1120306
- Veith A, Urich T, Seyfarth K, Protze J, Frazao C, Kletzin A. Substrate pathways and mechanisms of inhibition in the sulfur oxygenase reductase of Acidianus ambivalens. Front. Microbiol. 2011;2:37.
- Tano T, Kitaguchi H, Harda M, Nagasawa T, Sugio T. Purification and some properties of a tetrathionate decomposing enzyme from Thiobacillus thiooxidans. Biosci. Biotech. Biochem. 1996;60:224–227.10.1271/bbb.60.224
- de Jong GAH, Hazeu W, Bos P, Kuenen JG. Polythionate degradation by tetrathionate hydrolase of Thiobacillus ferrooxidans. Microbiology. 1997;143:499–504.10.1099/00221287-143-2-499
- Bugaytsova Z, Lindström EB. Localization, purification and properties of a tetrathionate hydrolase from Acidithiobacillus caldus. Eur. J. Biochem. 2004;271:272–280.10.1046/j.1432-1033.2003.03926.x
- Kanao T, Kamimura K, Sugio T. Identification of a gene encoding a tetrathionate hydrolase in Acidithiobacillus ferrooxidans. J. Biotechnol. 2007;132:16–22.10.1016/j.jbiotec.2007.08.030
- de Jong GAH, Hazeu W, Bos P, Kuenen JG. Isolation of the tetrathionate hydrolase from Thiobacillus acidophilus. Eur. J. Biochem. 1997;243:678–683.10.1111/ejb.1997.243.issue-3
- Protze J, Müller FH, Lauber K, Nass B, Mentele R, Lottspeich F, Kletzin A. An extracellular tetrathionate hydrolase from the thermoacidophilic archaeon Acidianus ambivalens with an activity optimum at pH 1. Front. Microbiol. 2011;2:68.
- Kanao T, Matsumoto C, Shiraga K, Yoshida K, Takada J, Kamimura K. Recombinant tetrathionate hydrolase from Acidithiobacillus ferrooxidans requires exposure to acidic conditions for proper folding. FEMS Microbiol. Lett. 2010;309:43–47.
- Rzhepishevska OI, Valdés J, Marcinkeviciene L, Gallardo CA, Meskys R, Bonnefoy V, Holmes DS, Dopson M. Regulation of a novel Acidithiobacillus caldus gene cluster involved in metabolism of reduced inorganic sulfur compounds. Appl. Environ. Microbiol. 2007;73:7367–7372.10.1128/AEM.01497-07
- Kanao T, Kosaka M, Yoshida K, Nakayama H, Tamada T, Kuroki R, Yamada H, Takada J, Kamimura K. Crystallization and preliminary X-ray diffraction analysis of tetrathionate hydrolase from Acidithiobacillus ferrooxidans. Acta Crystallogr. Sect. F Struct. Biol. Cryst. Commun. 2013;69:692–694.10.1107/S1744309113013419