Abstract
We previously isolated Rhodococcus sp. 065240, which catalyzes the defluorination of benzotrifluoride (BTF). In order to investigate the mechanism of this degradation of BTF, we performed proteomic analysis of cells grown with or without BTF. Three proteins, which resemble dioxygenase pathway enzymes responsible for isopropylbenzene degradation from Rhodococcus erythropolis BD2, were induced by BTF. Genomic PCR and DNA sequence analysis revealed that the Rhodococcus sp. 065240 carries the gene cluster, btf, which is highly homologous to the ipb gene cluster from R. erythropolis BD2. A mutant strain, which could not catalyze BTF defluorination, was isolated from 065240 strain by UV mutagenesis. The mutant strain had one mutation in the btfT gene, which encodes a response regulator of the two component system. The defluorinating ability of the mutant strain was recovered by complementation of btfT. These results suggest that the btf gene cluster is responsible for degradation of BTF.
Rhodococcus sp. 065240, which catalyzes the defluorination of benzotrifluoride (BTF), carries the gene cluster, btf. The dioxygenase pathway enzymes encoded by btf genes are responsible for degradation of BTF.
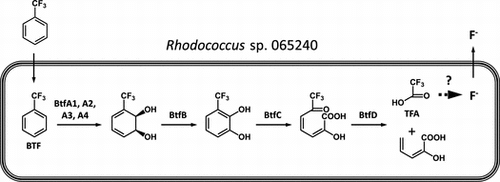
Fluorinated compounds are widely used in the fields of pharmacology, agrochemistry, and materials chemistry.Citation1,2) The unique properties of fluorine, such as strong electronegativity, and strong carbon–fluorine bond, may confer high stability in fluorinated compounds. Because of their unusual stability, residual fluorinated compounds in the environment have been an increasing problem. Recent reports have shown the presence of fluorinated organic compounds (FOCs), such as perfluorooctane sulfonate (PFOS), perfluorooctanoate (PFOA), and perfluorooctane sulfonylamide in soils and river water.Citation3–5) Notable concentrations of PFOS were also found in surface water and fish from Tokyo Bay.Citation5) Additionally, the use of hydrochlorofluorocarbons and hydrofluorocarbons as alternatives of chlorofluorocarbons may lead to accumulation of trifluoroacetic acid (TFA) in soils.Citation6) Consequently, these FOCs have been accumulating in wildlife and humans. It is reported that PFOA is currently detected in the blood of virtually all Americans at levels of about 4 ng/mL.Citation7,8) In Japan, blood PFOS concentrations ranged from 2.4 to 14 ng/mL.Citation5,9) The residues from fluoro-agrochemicals used abundantly in recent years are of greater concern. It is, therefore, important to understand their environmental fate as well as their biodegradation mechanism.
In order to find a clue to the solution of these problems, microbial defluorination technologies are being developed. While the biological degradation of chlorinated materials has been studied extensively,Citation10–12) little is known about the biodegradation of fluorinated compounds.Citation13,14) There are several reports concerning biological degradation of fluorinated materials: degradation of p-fluoroaniline with horseradish peroxidase,Citation15) fluoroacetate with monofluoroacetate dehalogenase (Pseudomonas indoloxidans, P. cepacia, Moraxella sp., Burkholderia sp.),Citation16–19) and TFA with an unknown enzyme(s).Citation6,20) Furthermore, studies on the metabolism of fluoroaromatic compounds, which are not natural compounds, have reported that the cleavage of the C–F bond occurs incidentally during oxygenase attack on the aromatic ring.Citation21−24) While microbial degradation of monofluoro compounds has been investigated, the mechanism of defluorination of the more stable trifluoromethyl group has not been reported.
In a previous study, we screened bacterial strains, which catalyze the defluorination of the fluorinated compounds, fluorobenzene (FB), and benzotrifluoride (BTF), and succeeded in isolating Rhodococcus sp. 065240, which catalyzes defluorination of the trifluoromethyl group of BTF.Citation25) Rhodococcus belongs to the mycolic acid-containing actinomycetes group, which includes Nocardia, Mycobacterium, and Corynebacterium.Citation26) It is known that the Rhodococcus group includes biodegraders of benzene (Rhodococcus opacus B4), isopropylbenzene (IPB) (Rhodococcus erythropolis BD2), and biphenyl (Rhodococcus sp. strain RHA1).Citation27–29) To the best of our knowledge, Rhodococcus sp. 065240 is the first bacterial strain reported to catalyze the defluorination of BTF. Analysis of BTF defluorination by the 065240 strain will contribute to understanding the mechanism of bacterial defluorination of the trifluoromethyl group. In this study, we performed proteomic analysis of 065240 cells grown with or without BTF. We found that BTF was degraded by a pathway closely related to the IPB degradation pathway in the 065240 strain.
Materials and methods
Bacterial strains and media
Rhodococcus sp. 065240 was used in this study.Citation25) Escherichia coli JM109 [recA1 endA1 gyrA96 thi hsdR17 e14− (mcrA−) supE44 relA1 Δ(lac-proAB)/F′ (traD36 proAB+ lacIq lacZΔM15)] and JM110 [dam dcm supE44 hsdR17 thi leu rpsL1 lacY galK galT ara tonA thr tsx Δ(lac-proAB)/F′ (traD36 proAB+ lac Iq lacZΔM15)] were used for plasmid constructions. Cells were grown at 30 °C in Lennox (L) broth containing 1% tryptone, 0.5% yeast extract, 0.5% NaCl, and 0.1% glucose (pH 7.0). Kanamycin (25 μg/mL in liquid medium and 50 μg/mL in solid medium for Rhodococcus sp., and 20 μg/mL for E. coli) was added when culturing cells carrying the plasmid.
For the BTF defluorination experiment, S medium containing 0.5% starch, 0.5% sucrose, 0.25% NZ amine, 0.25% polypeptone, 0.2% yeast extract, 0.1% extract ehlrich, 0.1% KH2PO4, and 0.05% MgSO4·7H2O was used.
Defluorination assay
The preculture of Rhodococcus sp. cells grown at 28 °C for 48 h was inoculated directly into the main culture medium at a volume of 1%. After 24–72 h of cultivation, 20 mM BTF was added to the culture, and cultivation was further continued at 28 °C for 24–192 h, aerobically. Since BTF is volatile, 20 mM BTF was supplemented one or two times to recover loss of BTF due to evaporation. The cultures were centrifuged to separate the cells from the supernatant. The concentration of the fluoride ion in the supernatant was determined by S500-Fluoride-pH/ION Meter with perfectION® combined fluoride sensor (Mettler Toledo, Greifensee, Switzerland).
Protein sample preparation and 2D-gel electrophoresis
Cells were harvested, suspended in 100 mM sodium phosphate buffer (pH 7.0), and disrupted by sonication. After unbroken cells was removed, cell lysate was centrifuged at 14,000 rpm for 15 min and the resultant supernatant was used for 2D-gel electrophoresis. Three μL of the cell extracts were dissolved in 150 μL of IPG buffer (urea 8 M, CHAPS 2% (w/v), 0.5% Zoom carrier ampholytes, 0.002% bromophenol blue, DTT 20 mM). The protein sample solutions were separated by isoelectric focusing using the Zoom Strips pH 4–7 and Zoom IPG runner system (Invitrogen, Carlsbad, CA). Focusing was performed in 3 steps (175 V for 15 min, 175–2000 V gradient for 1 h, and 2000 V for 30 min). NuPAGE 4–12% bis–tris Zoom gels in NuPAGE MOPS SDS running buffer (Invitrogen) were used for the second dimension. The gels were stained with Silver Stain II Kit (Wako, Osaka, Japan) or with coomassie brilliant blue. The protein spots were cut out and analyzed by mass spectrometry (MALDI-TOF/TOF ultrafleXtreme, Bruker Daltonics, Billerica, MA) after trypsin digestion.
Isolation of BTF degradation mutants
Dilution culture of Rhodococcus sp. 065240 was spread on a S agar plate at about 2000 cells/plate. The plate was irradiated for 20 s with UV light (64 W, 312 nm) at a distance of 1 cm. The survival rate was about 10%. Surviving colonies were assayed for BTF degradation.
DNA sequence analysis
The BTF degradation gene cluster was amplified by PCR with primer sets (Supplemental Table 1). The nucleotide sequences of the PCR products were determined by 3730xl DNA analyzer (Applied Biosystems, Foster City, CA). Homology searches were performed using the NCBI database along with the BLAST program (http://blast.ncbi.nlm.nih.gov).
Construction of plasmid
To construct a plasmid expressing the btfT gene, the DNA fragment carrying the ribosome-binding site (RBS) and the coding region of btfT was PCR-amplified using Rhodococcus sp. 065240 (wild type) chromosomal DNA as the template and a primer set, ipbT-sd2 and ipbTr2 (Supplemental Table 1). The amplified DNA fragment was digested with EcoRI and KpnI and ligated with the E. coli–Corynebacterium glutamicum shuttle vector pECtCitation30) digested with the same enzymes. The resulting plasmid was named pECt-btfT. For inducing the expression of btfT under the trc promoter on pECt vector, 1.0 mM IPTG was added to the culture medium.
To construct a plasmid expressing the btfA1–4 genes, the DNA fragment carrying RBS and the coding region of btfA1–4 was PCR-amplified using Rhodococcus sp. 065240 (wild type) chromosomal DNA as the template and a primer set, btfA1-eco-f and btfA4-t22-r (Supplemental Table 1). The amplified DNA fragment was digested with EcoRI and EcoT22I and ligated with the pECt plasmid digested with EcoRI and PstI. The resulting plasmid was named pECt-btfA1–4. For inducing the expression of btfA1–4 under the trc promoter on pECt vector, 0.1 mM IPTG was added to the culture medium.
Construction of a deletion mutant of btfA1–4 genes
Using Rhodococcus sp. 065240, a deletion mutant of btfA1–4 genes was constructed by 2-step homologous recombination, following the method used for C. glutamicum as described previously.Citation31) A DNA fragment (approximately 1.1 kb) carrying a thiostrepton-resistant (tsr) gene on the pIJ702Citation32) was PCR-amplified using pIJ702 as the template and a primer set, tsr-NheIf and tsr-NheIr (Supplemental Table 1). The amplified DNA fragment was digested with NheI and ligated with the vector plasmid pK18mobsacBCitation33) digested with the same enzyme. The newly constructed vector plasmid was named pK18mobsacB-tsr.
A 1.5-kb DNA fragment carrying the upstream flanking region and the ATG start codon of the btfA1 gene was PCR-amplified using a primer set, btfA1_-1451 and btfA1_13r (Supplemental Table 1). A 1.5-kb DNA fragment carrying the TGA stop codon and the downstream flanking region of the btfA4 gene was PCR-amplified using a primer set, btfA4_1207 and btfA4_ + 1437r (Supplemental Table 1). The PCR products were digested with BamHI and PstI or HindIII and PstI, respectively. The digested DNA fragments were cloned into the pK18mobsacB-tsr plasmid digested with BamHI and HindIII. The resulting plasmid, named pK18tsrΔbtfA1–4, was introduced into the wild-type 065240 strain by electroporation, and thiostrepton-resistant clones were selected (10 μg/mL thiostrepton). Because pK18tsrΔbtfA1–4 plasmid does not have a functional origin of replication in Rhodococcus sp., only those cells in which homologous recombination occurred between the chromosomal and plasmid DNA can grow on plates containing thiostrepton. Hence, thiostrepton-resistant transformants should carry the intact btfA1–4 genes, the deleted btfA1–4 genes, and the plasmid sequence on their chromosomes. One transformant was inoculated into L liquid medium without thiostrepton and grown at 30 °C for 2 days. The cells were then spread on L agar plates supplemented with 5% sucrose. Cells carrying the sacB gene encoded by the plasmid that had been integrated into the chromosome were killed in the presence of sucrose. Therefore, only those cells in which the sacB gene was excised from the chromosome by a second homologous recombination (between the intact and deleted btfA1–4) could grow on plates containing 5% sucrose. The btfA1–4 knockout strain was identified among sucrose-tolerant recombinants by PCR amplification with a primer set, preipbA1 and ipbC-90r (Supplemental Table 1). The btfA1–4 knockout strain was named DbtfA1–4.
Electroporation
Rhodococcus sp. R0418 mutant cells were grown in 0.1 L of L broth at 30 °C and harvested at log phase (OD660 = 0.6–0.8). Cells were washed once with ice-cold demineralized water and once with ice-cold 15% glycerol and suspended in ice-cold 15% glycerol. Cell suspension was either used directly for electroporation or stored in small aliquots at −80 °C. One hundred and twenty microliters of cell suspension was mixed with 400 ng of DNA in a 1-mm-gapped electrocuvette and subjected to a 2.5-kV electric pulse by an ECM 399 electroporator (BTX Harvard Apparatus, Holliston, MA). Pulsed cells were diluted immediately with 1 mL of L broth and incubated at 30 °C for 5 h, after which they were spread on L plates containing 50 μg/mL of kanamycin.
Analysis of fluoro-metabolites by 19F NMR
The culture supernatants of Rhodococcus sp. 065240 cells just after and 144 h after the first BTF addition were collected by centrifugation. The supernatants were freeze-dried and dissolved in DMSO-d6. After removing insoluble materials by centrifugation, the supernatants were analyzed by 19F nuclear magnetic resonance spectroscopy (19F NMR) using a Varian VXR-500 spectrometer (Varian, Palo Alto, CA). 19F NMR chemical shift values of the fluorinated metabolites were referenced to hexafluorobenzene (0.00 ppm).
DDBJ/EMBL/GenBank accession number
The nucleotide sequence data reported in this study will appear in the DDBJ/EMBL/GenBank nucleotide sequence databases with accession number AB970510.
Results
Proteomic analysis
Degradation enzymes are induced in the presence of substrate compounds, such as aromatic benzene, IPB, and biphenyl.Citation27,28,34) It is also possible that BTF induces degradation enzymes in the Rhodococcus sp. 065240. To identify the BTF degradation-related proteins, we first performed proteomic analysis. Protein samples were prepared from the 065240 cells grown in the presence or absence of BTF. As shown in Fig. (a), proteins of about 57, 49, 46, 42, 35, 33, 30, and 22 kDa were induced in the presence of BTF. Among them, the 57, 35, 33, 30, and 22 kDa proteins were also detected by the 2D-gel electrophoresis (Fig. (b)). These protein spots were used in peptide mass fingerprinting (PMF) analysis by MALDI-TOF mass-spectrometry. The peptide fingerprints of those proteins were compared with those from proteins of actinomycetes in the NCBI database using the MASCOT software. Protein at spot 1 was best matched with a protein chaperonin GroEL from R. opacus B4. Proteins at spots 2, 4, and, 5 were best matched with 3-isopropylcatechol (IPC) dioxygenase (ipbC gene product), 2-hydroxy-6-oxo-7-methylocta-2,4-dienoate (HOMODA) hydrolase (ipbD), and IPB dioxygenase (ipbA2), respectively, all from R. erythropolis BD2 (Table ). These three enzymes are known as IPB-degrading enzymes.Citation35) Identification of spot 3 was not successful.
Fig. 1. Proteomic analysis of Rhodococcus sp. 065240 grown in the presence or absence of BTF.
Notes: Twenty millimole BTF was added to the culture at 72 h, and cultivation was further continued for 24 h. Cell lysates of Rhodococcus sp. 065240 grown in the presence or absence of BTF were analyzed by 10% SDS-PAGE. A coomassie brilliant blue-stained gel is shown (a). 2D-gel electrophoresis of proteins of the 065240 strain grown in the presence or absence of BTF. 2D-gel electrophoresis was done as described in the Materials and methods Section. A silver-stained gel is shown. Arrows indicate the BTF-induced protein spots, which were analyzed by MALDI-TOF mass-spectrometry (b). Gels around spot 1 are highlighted (c).
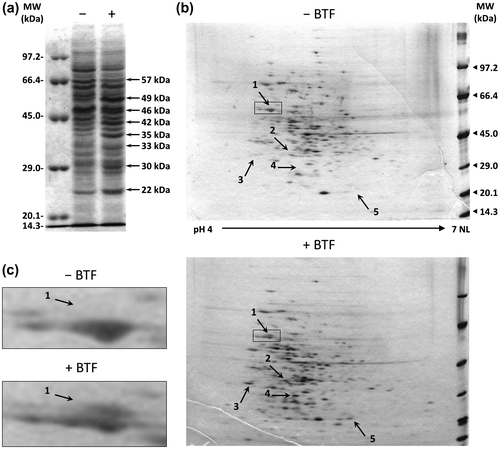
Table 1. Summary of protein identification by 2D-PAGE and PMF analysis.
Sequencing analysis of BTF degradation-related genes
The PMF analysis suggested that Rhodococcus sp. 065240 catalyzed defluorination of BTF through the IPB degradation-related pathway. We therefore designed primer sets corresponding to the R. erythropolis BD2 ipb genesCitation35) (Supplemental Table 1). PCR products were successfully amplified with primer sets for ipbA1 (IPB dioxygenase, iron-sulfur protein (ISP) large subunit, 1383 bp), ipbA2 (IPB dioxygenase, ISP small subunit, 564 bp), ipbA3 (IPB dioxygenase, ferredoxin, 324 bp), ipbA4 (IPB dioxygenase, ferredoxin reductase, 1239 bp), ipbB (IPB dihydrodiol dehydrogenase, 813 bp), ipbC (IPC dioxygenase, 954 bp), ipbD (HOMODA hydrolase, 825 bp), ipbS (putative sensor kinase, 4776 bp), and ipbT (putative response regulator, 630 bp) using chromosomal DNA of the 065240 strain as a template. The PCR products showed close similarity to the corresponding ipb genes of R. erythropolis BD2Citation35) (Fig. , Supplemental Table 2). Here, these BTF degradation-related genes were named btfA1, btfA2, btfA3, btfA4, btfB, btfC, btfD, btfS, and btfT, respectively. Each btf gene is highly homologous to the corresponding ipb gene (99–100%). Base substitutions were only found in the btfA1 gene (4 bases), btfA3 (1 base), btfA4 (1 base), btfB (3 bases), btfS (2 bases and a 3-base insertion), and btfT (1 base). These caused amino acid substitutions in BtfA1 (Ala284 → Thr, Leu287 → Trp, and Val311 → Ala), BtfA3 (Glu56 → Lys), BtfA4 (Ala62 → Ser), BtfS (Leu408 → Pro and Ala insertion at 423th), and BtfT (Ala31 → Thr). On the other hand, a spacer region of btfT and btfD showed low homology to that of ipbT and ipbD.
Fig. 2. Comparison of the DNA sequences of the btf gene cluster in the 065240 strain and the ipb gene cluster in R. erythropolis BD2.
Notes: Physical map of the 23-kb DNA region containing the ipb gene cluster in R. erythropolis BD2 (GenBank accession number AY223810) (a). Physical map of the 21-kb DNA region containing the btf gene cluster in the 065240 strain (b). Black arrows represent ipb or btf gene clusters. Gray arrows represent genes such as hypothetical ORFs and transposases. The IpbST- and BtfST-inducible promoters (PipbA1, PipbD, PbtfA1, PbtfD) are indicated by black triangles in white rectangles.
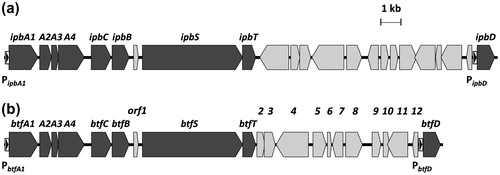
Isolation of a mutant deficient in BTF degradation
To isolate mutant strains of Rhodococcus sp. 065240, which could not catalyze BTF defluorination, UV mutagenesis was performed as described in the Materials and methods Section. Five hundred clones were screened in the BTF defluorination assay. About 80% of the clones defluorinated BTF at the same level as the wild type. About 20% of the clones showed relatively lower defluorination ability to the wild type. Among them, one strain, named R0418, showed the lowest defluorination ability (Fig. ). R0418 mutant still retained the ability to defluorinate FB.
Fig. 3. Degradation of FB and BTF by the R0418 mutant strain.
Notes: Twenty millimole BTF or FB was added to the cultures at 24 h, and cultivation was further continued for 192 h. Twenty millimole BTF or FB was supplemented again 72 h after the first BTF addition. Fluoride ion concentrations of culture supernatants grown with fluoro-compounds were measured. Control, no substrate; FB, fluorobenzene; BTF, benzotrifluoride; WT, Rhodococcus sp. 065240; R0418, R0418 mutant.
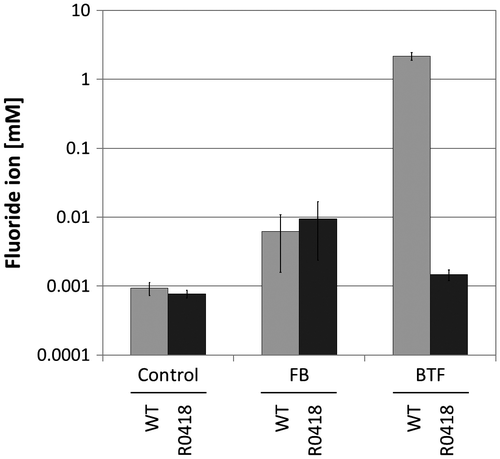
The mutant R0418 btf gene cluster was sequenced, and one point mutation (493th A to G) was found in the btfT gene encoding the transcriptional regulator protein of the two-component system BtfST, resulting in one amino acid substitution, Asn165 → Asp.
Complementation of R0418 mutant
To confirm that the btf genes are involved in the degradation of BTF, we did a complementation test of the R0418 mutant strain using the vector plasmid pECt, which is known as a shuttle vector between E. coli and C. glutamicum.Citation30) It was found that pECt was stably maintained in Rhodococcus sp. 065240 cells (data not shown). The btfT gene, including the RBS sequence, was amplified by PCR from the wild-type chromosomal DNA, and the amplified fragment was inserted into pECt. The constructed plasmid pECt-btfT was introduced into the R0418 mutant strain by electroporation. The transformant was grown in the presence of 20 mM BTF for 3 days, and then, the fluoride ion concentration in the culture medium was measured. As a result, the transformant recovered the ability to defluorinate BTF at the same level as the wild type (Fig. ), indicating that the Asn165 → Asp substitution in BtfT resulted in a loss of function.
Fig. 4. Complementation of R0418 mutant by the wild-type btfT gene.
Notes: Twenty millimole BTF was added to the cultures at 57 h, and cultivation was further continued for 83 h. Twenty millimole BTF was supplemented again 50 h after the first BTF addition. Fluoride ion concentrations of culture supernatants grown with BTF were measured. WT, Rhodococcus sp. 065240; R0418, R0418 mutant; pECt, vector plasmid; pECt-btfT, pECt carrying the wild-type btfT gene.
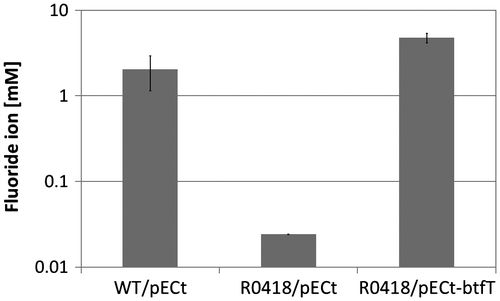
Effect of ΔbtfA1–4 mutation on the degradation of BTF
The complementation test of the R0418 mutant strain suggested that the btf gene cluster is involved in the BTF degradation in 065240 strain. However, it is reported that in the R. jostii RHA1, a transcriptional regulator protein, BphT1, which is homologous to BtfT, activated not only expression of genes in its gene cluster but also genes in another gene cluster.Citation36) Therefore, it is also possible that BtfT activates expression of other genes than the btf cluster. To confirm involvement of the btf gene cluster in BTF degradation, we constructed a deletion mutant of btfA1–4 genes, named DbtfA1–4. It was found that the DbtfA1–4 mutant lost the defluorination ability, like the R0418 mutant (Fig. ). Furthermore, the defluorination ability of DbtfA1–4 was recovered by introduction of pECt-btfA1–4. This directly indicates that the btf gene cluster is responsible for degradation of BTF in the 065240 strain. At least, a dioxygenase encoded by btfA1–4 is required for degradation of BTF.
Fig. 5. Degradation of BTF by the DbtfA1–4 mutant strain.
Notes: Twenty millimole BTF was added to the cultures at 50 h, and cultivation was further continued for 77 h. Twenty millimole BTF was supplemented again 48 h after the first BTF addition. Fluoride ion concentrations of culture supernatants grown with BTF were measured. WT, Rhodococcus sp. 065240; DbtfA1–4, btfA1–4 knockout strain; pECt, vector plasmid; pECt-btfA1–4, pECt carrying the intact btfA1–4 genes.
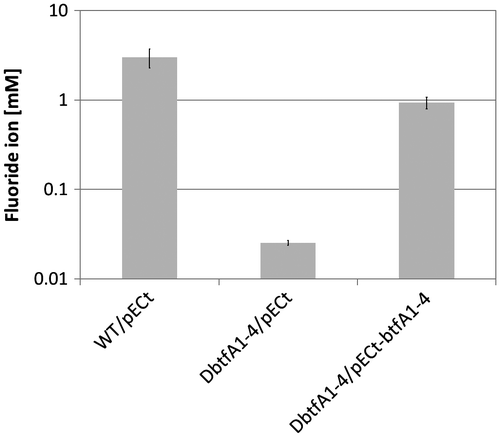
Analysis of fluoro-metabolites in the culture of the 065240 strain grown in the presence of BTF
Our proteomic and genetic analyses strongly suggest that BTF was degraded via the dioxygenase pathway, which resembles the IPB pathway (see Fig. ). To confirm this speculation, fluoro-metabolites in the culture supernatants of the 065240 strain grown in the presence of BTF were analyzed by 19F NMR. As shown in Fig. , at the time of BTF addition, no fluoro-metabolites were detected in the culture other than BTF. After 144 h cultivation, the signal of BTF was decreased and many signals of fluoro-metabolites were detected instead. Among them, a signal at 89.0 ppm was assigned to that of TFA. This supports our speculation that BTF was degraded through the dioxygenase pathway similarly to the IPB pathway (Fig. ).
Fig. 6. Comparison of IPB and BTF degradation pathways.
Notes: Proposed degradation pathway of IPBCitation27,35) (a). Hypothetical degradation pathway of BTF by the 065240 strain (b). Compounds: I, IPB; II, cis-2,3-dihydro-2,3-dihydroxyIPB; III, IPC; IV, HOMODA; V, isobutyric acid; VI, 2-hydroxypenta-2,4-dienoic acid; VII, BTF; VIII, cis-2,3-dihydro-2,3-dihydroxyBTF; IX, 3-trifluoromethylcatechol; X, 2-hydroxy-6-oxo-7,7,7-trifluorohepta-2,4-dienoic acid; XI, TFA. Fluoride ions are released from TFA or other fluorinated intermediate metabolites. For details, see the text.
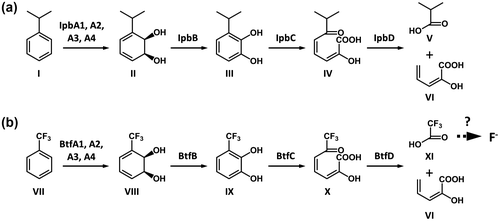
Fig. 7. 19F NMR spectra of the culture supernatants of Rhodococcus sp. 065240 grown in the presence of BTF.
Notes: Twenty millimole BTF was added to the cultures at 53 h, and cultivation was further continued for 144 h. Twenty millimole BTF was supplemented 48 and 120 h after the first BTF addition. The culture supernatants just after (0 h) and 144 h after the first BTF addition were analyzed by 19F NMR as described in the Materials and methods. A part of the spectrum (70–110 ppm) is shown. Spectra of authentic BTF and TFA were shown below. The chemical shift values were referenced to hexafluorobenzene (0.00 ppm).
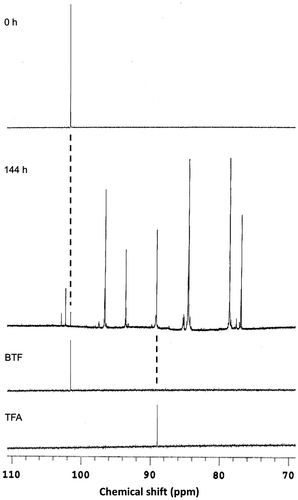
Effects of BTF analogs on the induction of the btf gene cluster
We then checked the substrate spectrum of the BTF degradation pathway. Protein expression was examined by 2D-gel electrophoresis in the presence of BTF analogs (Table ). It was found that IPB and biphenyl induced BTF-degrading proteins (spots 2, 4, and 5 in Table ) at almost similar levels to that of BTF. Benzene, t-butylbenzene, and toluene also induced the same BTF-degradation proteins, although at lower levels than that seen with BTF. Benzoic acid did not induce the BTF-degradation proteins. Interestingly, the GroEL protein (spot 1 in Table ) was induced only in the presence of BTF. The effects of BTF on protein expression in the R0418 mutant strain were also studied. The BTF-degrading proteins were not induced in the mutant strain (Supplemental Fig. 1). This confirmed that the R0418 btfT mutation is a loss-of-function type mutation. On the other hand, the GroEL protein (spot 1) was expressed at higher levels in the R0418 mutant than in the wild type.
Table 2. Substrate spectrum of protein induction in the Rhodococcus sp. 065240.
Discussion
In this study, we analyzed the mechanism of degradation of BTF by Rhodococcus sp. 065240. We first carried out a proteomic analysis of the 065240 strain grown in the presence or absence of BTF. As a result, it was found that three proteins, which resemble the IPB-degradation enzymes, IpbA2, IpbC, and IpbD from R. erythropolis BD2, were induced in the 065240 cells grown in the presence of BTF (Table ). Genomic PCR revealed that the 065240 strain indeed carries a gene cluster, named the btf gene cluster, which is highly homologous to the ipb gene cluster of R. erythropolis BD2. The btf gene cluster contains all genes corresponding to the ipbA1, ipbA2, ipbA3, ipbA4, ipbB, ipbC, and ipbD, in addition to the ipbS and ipbT, encoding the two-component system IpbST, which control the expression of the ipb genesCitation35) (Fig. ). Since BTF structurally resembles IPB (the trifluoromethyl group is roughly as bulky as the isopropyl group),Citation37) this btf gene cluster is very likely to be involved in Rhodococcus sp. 065240’s degradation of BTF.
To confirm this supposition, we isolated mutants from Rhodococcus sp. 065240. One mutant strain, named R0418, which almost completely lost the ability to degrade BTF, was isolated after UV mutagenesis (Fig. ). DNA sequencing analysis of the mutant btf gene cluster revealed that the mutant R0418 strain carried one point mutation in its btfT gene, which caused the Asn165 → Asp substitution. In the R0418 mutant cells, induction of IpbA2, IpbC, and IpbD was not observed in the presence of BTF (Table ). The btfT gene is highly homologous to the genes of the two-component response regulator of the bnz gene cluster in R. opacus B4 and the bph gene cluster in Rhodococcus jostii RHA1.Citation36) The Asn165 of BtfT is conserved in these homologs and is considered to be involved in DNA binding. It is likely that the Asn165 → Asp substitution in the BtfT affected DNA-binding activity, resulting in a loss of function. The R0418 mutant was complemented by the wild-type btfT gene on the plasmid (Fig. ). These results suggested that the btf gene cluster is responsible for the degradation of BTF in the 065240 strain. Interestingly, R0418 still retained the ability to defluorinate FB. Moreover, a protein induction profile by FB was different from that by BTF (data not shown). These suggest that defluorination of FB was catalyzed by an independent pathway of the BTF degradation pathway.
To confirm the involvement of the btf gene cluster in BTF degradation, we constructed a deletion mutant of btfA1–4 genes, named DbtfA1–4, and found that the DbtfA1–4 mutant lost the defluorination ability, like the R0418 mutant (Fig. ). We also showed that the defluorination ability of DbtfA1–4 was recovered by introduction of pECt-btfA1–4. These results clearly indicate that the btf gene cluster is responsible for degradation of BTF in the 065240 strain. At least, a dioxygenase encoded by btfA1–4 is essential for degradation of BTF. This indicates that the first step of BTF degradation should be catalyzed enzymatically.
In our typical culture conditions (Figs. ), a total of 40 mM BTF was added to the culture, and at most, 3 mM fluoride ions were released in the culture supernatants. Overall efficiency of defluorination was 2.5%. However, as BTF was evaporated during the cultivation, the net efficiency of defluorination should be higher.
A possible BTF degradation pathway was predicted based on the Ipb pathway (Fig. ). BTF degradation is initiated by a BTF 2,3-dioxygenase (dioxygenase large subunit (BtfA1), dioxygenase small subunit (BtfA2), dioxygenase ferredoxin subunit (BtfA3), and dioxygenase ferredoxin reductase subunit (BtfA4)) and a 2,3-dihydro-2,3-dihydroxyBTF dehydrogenase (BtfB), which gives 3-trifluoromethylcatechol through cis-2,3-dihydro-2,3-dihydroxyBTF. 3-Trifluoromethylcatechol subsequently undergoes a meta-cleavage reaction by a 3-trifluoromethylcatechol 2,3-dioxygenase (BtfC), and the resulting meta-cleavage product, 2-hydroxy-6-oxo-7,7,7-trifluorohepta-2,4-dienoic acid, is hydrolyzed to TFA and 2-hydroxypenta-2,4-dienoic acid by hydrolase (BtfD). To confirm this speculation, fluoro-metabolites in the culture supernatants were analyzed using 19F NMR, and the signal of TFA was detected (Fig. ). These suggest that defluorination occurred after degradation by the Btf pathway.
Homologous dioxygenase pathways are also reported for benzene (bnz gene cluster in R. opacus B4) and biphenyl (bph gene cluster in Rhodococcus sp. strain RHA1).Citation28,34) BTF degradation ability of these two strains, as well as R. erythropolis BD2, needs to be examined. In this study, we showed that the dioxygenase encoded by btfA1–4 genes was essential for BTF degradation. This enzyme is highly homologous to the counterparts of the above Rhodococcus strains. Moreover, the downstream enzymes of the dioxygenase pathways are also highly homologous. Therefore, it is possible that these Rhodococcus strains also catalyze degradation of BTF, at least, from BTF to TFA. To identify an enzyme(s) to catalyze defluorination, further metabolomics analysis of fluoro-metabolites as well as further genetic analysis needs to be done. In the spacer region of btfT and btfD, putative transposase genes (orf2 and orf3) were found (Supplemental Table 2). It is likely that these gene clusters have been distributed among Rhodococcus species via interspecies gene transfer.
We also examined the substrate spectrum of the BTF degradation pathway (Table ). IPB and biphenyl induced the BTF degradation enzymes at almost similar levels to that of BTF. Benzene, t-butylbenzene, and toluene also induced the enzymes at lower levels. These suggest that the same pathway that degrades BTF could also degrade these aromatic compounds. At the very least under our experimental conditions, the BtfST two component system recognizes these compounds and induces the BTF-degrading enzymes. Since we used the S medium, which contains relatively rich nutrients, there is a possibility that the medium composition affected gene expression profiles.
Interestingly, GroEL protein, as well as the protein at spot 3 obtained from 2D-gel electrophoresis in Fig. , was induced only by BTF. These two proteins were induced also in the btfT mutant R0418. These results suggest that BTF induced the stress response in the Rhodococcus sp. 065240 cells. It is well known that the GroEL protein is induced under conditions that cause protein denaturation, such as high temperature.Citation38) It is likely that BTF also causes protein denaturation. Identification of the spot 3 protein was not successful. It is possible that there is no homologous protein to the spot 3 protein in the database. It is also possible that trypsin digestion was insufficient for PMF analysis.
In this study, we used an E. coli–C. glutamicum shuttle vector pECt for complementation test. Corynebacterium and Rhodococcus both belong to the my colic acid-containing actinomycetes group.Citation26) This is the first example that shows that the C. glutamicum plasmid can proliferate in the Rhodococcus cells. C. glutamicum is an industrially important microorganism and therefore has been investigated extensively.Citation39) It is worth examining if genetic tools and techniques used for C. glutamicum, such as plasmid vectors and transposon mutagenesis,Citation40,41) can be applied to investigations of the Rhodococcus species.
Supplemental material
The supplemental material for this paper is available at http://dx.doi.org/10.1080/09168451.2014.982502.
Supplemental Materials
Download Zip (1.4 MB)Acknowledgment
We thank the Technical Department, Tokyo Institute of Technology for technical assistance in mass spectrometry.
Additional information
Funding
References
- Key DJ, Howell RD, Criddle CS. Fluorinated organics in the biosphere. Environ. Sci. Technol. 1997;31:2445–2454.10.1021/es961007c
- Lewandowski G, Meissner E, Milchert E. Special applications of fluorinated organic compounds. J. Hazard. Mat. 2006;136:385–391.10.1016/j.jhazmat.2006.04.017
- Giesy JP, Kannan K. Global distribution of perfluorooctane sulfonate in wildlife. Environ. Sci. Technol. 2001;35:1339–1342.10.1021/es001834k
- Saito N, Harada K, Inoue K, Sasaki K, Yoshinaga T, Koizumi A. Perfluorooctanoate and perfluorooctane sulfonate concentrations in surface water in Japan. J. Occup. Health. 2004;46:49–59.10.1539/joh.46.49
- Taniyasu S, Kannan K, Horii Y, Hanari N, Yamashita N. A survey of perfluorooctane sulfonate and related perfluorinated organic compounds in water, fish, birds, and humans from Japan. Environ. Sci. Technol. 2003;37:2634–2639.10.1021/es0303440
- Visscher PT, Culbertson CW, Oremland RS. Degradation of trifluoroacetate in oxic and anoxic sediments. Nature. 1994;369:729–731.10.1038/369729a0
- Calafat AM, Wong LY, Kuklenyik Z, Reidy JA, Needham LL. Polyfluoroalkyl chemicals in the U.S. population: data from the national health and nutrition examination survey (NHANES) 2003–2004 and comparisons with NHANES 1999–2000. Environ. Health Perspect. 2007;115:1596–1602.10.1289/ehp.10598
- Steenland K, Woskie S. Cohort mortality study of workers exposed to perfluorooctanoic acid. Am. J. Epidemiol. 2012;176:909–917.10.1093/aje/kws171
- Inoue K, Okada F, Ito R, Kato S, Sasaki S, Nakajima S, Uno A, Saijo Y, Sata F, Yoshimura Y, Kishi R, Nakazawa H. Perfluorooctane sulfonate (PFOS) and related perfluorinated compounds in human maternal and cord blood samples: assessment of PFOS exposure in a susceptible population during pregnancy. Environ. Health Perspect. 2004;112:1204–1207.10.1289/ehp.6864
- Janssen DB, Pries F, Van der Ploeg JR. Genetics and biochemistry of dehalogenating enzymes. Annu. Rev. Microbiol. 1994;48:163–191.10.1146/annurev.mi.48.100194.001115
- Phillips TM, Seech AG, Lee H, Trevors JT. Biodegradation of hexachlorocyclohexane (HCH) by microorganisms. Biodegradation. 2005;16:363–392.10.1007/s10532-004-2413-6
- Smidt H, de Vos WM. Anaerobic microbial dehalogenation. Annu. Rev. Microbiol. 2004;58:43–73.10.1146/annurev.micro.58.030603.123600
- Morii Y, Hasumi F, Kitazume T. Accumulation of 2,2-difluoroethanol by bacteria. J. Fluorine Chem. 2004;125:731–733.10.1016/j.jfluchem.2003.12.009
- Nataranjan R, Azerad R, Badet B, Copin E. Microbial cleavage of the C–F bond. J. Fluorine Chem. 2005;126:425–436.
- Goldman P, Milne GWA. Carbon–fluorine bond cleavage. II. Studies on the mechanism of the defluorination of fluoroacetate. J. Biol. Chem. 1966;241:5557–5559.
- Horiuchi N. The C–F bond rupture in mono-fluoroacetate by soil microbes. J. Agric. Chem. Soc. Jpn. 1961;35:870–873.
- Kawasaki H, Tsuda K, Matsushita I, Tonomura K. Lack of homology between two haloacetate dehalogenase genes encoded on a plasmid from Moraxella sp. strain B. J. Gen. Microbiol. 1992;138:1317–1323.10.1099/00221287-138-7-1317
- Kurihara T, Yamauchi T, Ichiyama S, Takahata H, Esaki N. Purification, characterization, and gene cloning of a novel fluoroacetate dehalogenase from Burkholderia sp. FA1. J. Mol. Catal. B: Enzym. 2003;23:347–355.10.1016/S1381-1177(03)00098-5
- Tsang JSH, Sallis PJ, Bull AT, Hardman DJ. A monobromoacetate dehalogenase from Pseudomonas cepacia MBA4. Arch. Microbiol. 1988;150:441–446.10.1007/BF00422284
- Kim BR, Suidan MT, Wallington TJ, Du X. Biodegradability of trifluoroacetic acid. Environ. Eng. Sci. 2000;17:337–342.10.1089/ees.2000.17.337
- Carvalho MF, Ferreira MI, Moreira IS, Castro PM, Janssen DB. Degradation of fluorobenzene by rhizobiales strain F11 via ortho cleavage of 4-fluorocatechol and catechol. Appl. Environ. Microbiol. 2006;72:7413–7417.10.1128/AEM.01162-06
- Carvalho MF, Jorge RF, Pacheco CC, Marco PD, Castro PML. Isolation and properties of a pure bacterial strain capable of fluorobenzene degradation as sole carbon and energy source. Environ. Microbiol. 2005;7:294–298.10.1111/emi.2005.7.issue-2
- Song B, Palleroni NJ, Haggblom MM. Isolation and characterization of diverse halobenzoate-degrading denitrifying bacteria from soils and sediments. Appl. Environ. Microbiol. 2000;66:3446–3453.10.1128/AEM.66.8.3446-3453.2000
- Vargas C, Song B, Camps M, Häggblom MM. Anaerobic degradation of fluorinated aromatic compounds. Appl. Microbiol. Biotechnol. 2000;53:342–347.10.1007/s002530050032
- Iwai N, Sakai R, Tsuchida S, Kitazume M, Kitazume T. Screening of fluorinated materials degrading microbes. J. Fluorine Chem. 2009;130:434–437.10.1016/j.jfluchem.2009.02.004
- Chun J, Kang SO, Hah YC, Goodfellow M. Phylogeny of mycolic acid-containing actinomycetes. J. Ind. Microbiol. 1996;17:205–213.10.1007/BF01574694
- Dabrock B, Kesseler M, Averhoff B, Gottschalk G. Identification and characterization of a transmissible linear plasmid from Rhodococcus erythropolis BD2 that encodes isopropylbenzene and trichloroethene catabolism. Appl. Environ. Microbiol. 1994;60:853–860.
- Na KS, Kuroda A, Takiguchi N, Ikeda T, Ohtake H, Kato J. Isolation and characterization of benzene-tolerant Rhodococcus opacus strains. J. Biosci. Bioeng. 2005;99:378–382.10.1263/jbb.99.378
- Seto M, Kimbara K, Shimura M, Hatta T, Fukuda M, Yano K. A novel transformation of polychlorinated biphenyls by Rhodococcus sp. strain RHA1. Appl. Environ. Microbiol. 1995;61:3353–3358.
- Sato H, Orishimo K, Shirai T, Hirasawa T, Nagahisa K, Shimizu H, Wachi M. Distinct roles of two anaplerotic pathways in glutamate production induced by biotin limitation in Corynebacterium glutamicum. J. Biosci. Bioeng. 2008;106:51–58.10.1263/jbb.106.51
- Gao YG, Suzuki H, Itou H, Zhou Y, Tanaka Y, Wachi M, Watanabe N, Tanaka I, Yao M. Structural and functional characterization of the LldR from Corynebacterium glutamicum: a transcriptional repressor involved in L-lactate and sugar utilization. Nucleic Acids Res. 2008;36:7110–7123.10.1093/nar/gkn827
- Katz E, Thompson CJ, Hopwood DA. Cloning and expression of the tyrosinase gene from Streptomyces antibioticus in Streptomyces lividans. J. Gen. Microbiol. 1983;129:2703–2714.
- Schäfer A, Tauch A, Jäger W, Kalinowski J, Thierbach G, Pühler A. Small mobilizable multi-purpose cloning vectors derived from the Escherichia coli plasmids pK18 and pK19: selection of defined deletions in the chromosome of Corynebacterium glutamicum. Gene. 1994;145:69–73.10.1016/0378-1119(94)90324-7
- Seto M, Masai E, Ida M, Hatta T, Kimbara K, Fukuda M, Yano K. Multiple polychlorinated biphenyl transformation systems in the gram-positive bacterium Rhodococcus sp. strain RHA1. Appl. Environ. Microbiol. 1995;61:4510–4513.
- Stecker C, Johann A, Herzberg C, Averhoff B, Gottschalk G. Complete nucleotide sequence and genetic organization of the 210-kilobase linear plasmid of Rhodococcus erythropolis BD2. J. Bacteriol. 2003;185:5269–5274.10.1128/JB.185.17.5269-5274.2003
- Takeda H, Shimodaira J, Yukawa K, Hara N, Kasai D, Miyauchi K, Masai E, Fukuda M. Dual two-component regulatory systems are involved in aromatic compound degradation in a polychlorinated-biphenyl degrader, Rhodococcus jostii RHA1. J. Bacteriol. 2010;192:4741–4751.10.1128/JB.00429-10
- MacPhee JA, Panaye A, Dubois JE. A critical examination of the Taft steric parameter – ES. Definition of a revised, broader and homogeneous scale. Extension to highly congested alkyl groups. Tetrahedron. 1978;34:3553–3562.10.1016/0040-4020(78)88431-2
- Martin J, Horwich AL, Hartl FU. Prevention of protein denaturation under heat stress by the chaperonin Hsp60. Science. 1992;258:995–998.10.1126/science.1359644
- Yamashita C, Hashimoto K, Kumagai K, Maeda T, Takada A, Yabe I, Kawasaki H, Wachi M. L -glutamate secretion by the N-terminal domain of the Corynebacterium glutamicum NCgl1221 mechanosensitive channel. Biosci. Biotechnol. Biochem. 2013;77:1008–1013.10.1271/bbb.120988
- Tsuchida Y, Kimura S, Suzuki N, Inui M, Yukawa H. Characterization of a new 2.4-kb plasmid of Corynebacterium casei and development of stable corynebacterial cloning vector. Appl. Microbiol. Biotechnol. 2009;81:1107–1115.10.1007/s00253-008-1746-1
- Suzuki N, Okai N, Nonaka H, Tsuge Y, Inui M, Yukawa H. High-throughput transposon mutagenesis of Corynebacterium glutamicum and construction of a single-gene disruptant mutant library. Appl. Environ. Microbiol. 2006;72:3750–3755.10.1128/AEM.72.5.3750-3755.2006