Abstract
Fluoroacetamide (Mw = 77.06) is a lethal rodenticide to humans and animals which is still frequently abused in food storage somewhere in China. The production of antibodies for fluoroacetamide is difficult due to its high toxicity to animals, which limits the application of immunoassay method in poison detection. In this work, aptamers targeting N-fluoroacetyl glycine as an analog of fluoroacetamide were selected by a specific systematic evolution of ligands by exponential enrichment (SELEX) strategy. The binding ability of the selected aptamers to fluoroacetamide was identified using surface plasmon resonance (SPR)-based assay. The estimated KD values in the low micromolar range showed a good affinity of these aptamers to the target. Our work verified that the SELEX strategy has the potential for developing aptamers targeted to small molecular toxicants and aptamers can be employed as new recognition elements instead of antibodies for poison detection.
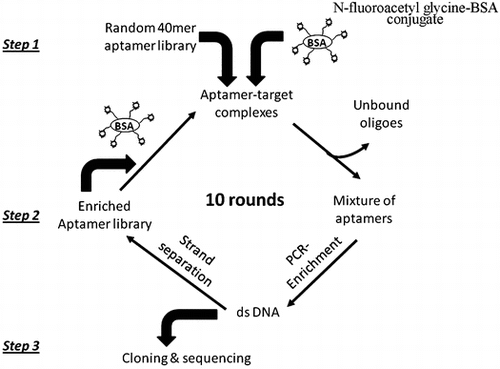
Fluoroacetamide (C2H4FNO, CAS No. 640-19-7, Fig. (A)), a low molecular weight toxicant, was used as agricultural insecticide and rodenticide. The oral median lethal dose (LD50) of rats is 15 mg/kg. A dose of 0.1–0.5 g was considered lethal for human beings.Citation1) Considering its acute and secondary toxicity, it has been prohibited to produce, sell, and use by Chinese government since the 1970s. However, it is still illegally applied for deratization due to its ease of synthesis, cheap price, good palatability, and strong temptation to mice. For that it has no odor or flavor and high solubility in water, it is often accidentally eaten due to food/drink contamination, or intentionally used to poison human or other animals.Citation2) Therefore, fluoroacetamide becomes one of the main objects for toxicology analysis.
Fig. 1. Structure formula of fluoroacetamide and its analog, N-fluoroacetyl glycine (A) and synthetic steps of N-fluoroacetyl glycine (B).
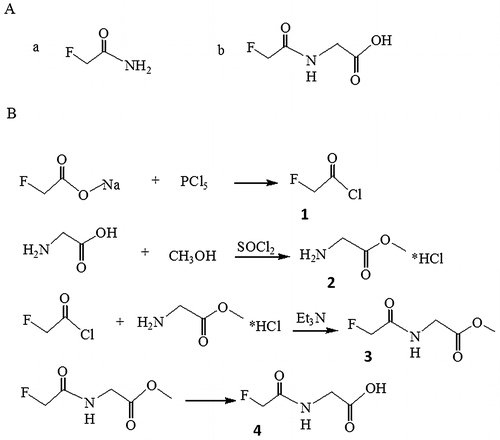
So far, a number of classical analytical methods, such as thioindigo reaction, GC/MS, GC/FID, and GC/NPD have been described to detect and quantify fluoroacetamide.Citation3–5) However, the chemical method has low sensitivity, poor stability, and is easy to produce false positive. The instrumental methods rely on expensive equipment and qualified handling skills. Moreover, they are time-consuming with regard to the necessity to derivatize fluoroacetamide. Thus, these methods are not suitable for onsite rapid detection. The conventional rapid detection method is based on immunoassay with antibodies as molecular recognition elements.Citation6) Small molecules like fluoroacetamide generally cannot elicit an immune response alone for antibody generation. More importantly, high toxicity of fluoroacetamide to animals makes it impossible to produce antibodies, and even the antibodies can be obtained, they will become unstable under unfavorable conditions. All these reasons greatly limit the application of immunoassay method in rapid detection of fluoroacetamide.
Aptamers are ssDNA or RNA that can bind to a target with high affinity and specificity.Citation7) They are commonly selected in vitro from an initial random library containing 1013–1015 oligonucleotides by SELEX strategy.Citation8) The typical SELEX process consists of some repeated steps that is selection (binding, partition, and elution), amplification, and relevant ssDNA separation. In general, 6-20 SELEX rounds are needed for aptamer selection. After the last round, the enriched aptamer pool is cloned, and several candidate sequences have to be characterized.Citation9,10) Aptamers have several advantages competed with antibodies. DNA aptamers can resist high temperature and severe pH condition. The affinity and specificity of aptamers bound to target are equal or even superior to antibodies. Once selected, aptamers can be chemically synthesized with high purity, avoiding batch-to-batch variation. They can be chemically modified with functional groups to improve the performance and facilitate the immobilization. What’s more, the selection process of aptamers doesn’t depend on animals or cell lines which makes it possible to develop the aptamers for toxic targets.Citation11) Therefore, aptamers show the potential to replace antibodies as molecular recognition elements for developing novel analysis assays and systems.Citation12) Since its introduction in 1990, aptamer technology has been applied in clinical diagnostics,Citation13,14) therapeutics, Citation15,16) bioanalysis (in the form of test kits, biosensor et al.)Citation17–19), and separation and purification.Citation20)
The aim of this study was to obtain a fluoroacetamide-specific aptamer with good affinity. To immobilize the small molecular target on the solid matrix, we successfully introduced an active group onto the fluoroacetamide and develop a target-protein conjugate. The SELEX strategy based on microplates and SPR assay method were applied to select and identify the aptamer. As mentioned above, the aptamer-based method will provide a promising option for rapid monitoring and screening fluoroacetamide.
Materials and methods
Chemicals and reagents
Sodium fluoroacetate, phosphorus pentachloride, glycine, thionyl chloride, and NaOH were purchased from Sinopharm Chemical Reagent Co. Ltd. (Shanghai, China). Methanol, ethanamine-triethylamine, ethyl acetate, chloroform, HCl, and ethanol were purchased from Shanghai Lingfeng Chemical Reagent Co. Ltd. (Shanghai, China). N-hydroxysuccinimide (NHS), 1-ethyl-3-(3-dimethylaminopropyl)-carbodiimide (EDC), Casein-Na, and ethanolamine were obtained from Sigma. Bovine serum albumin (BSA) was purchased from Merck. The random ssDNA library and primers were synthesized by Life Technologies. Their sequences were listed as follows: ssDNA library: 5′-GCG GAT GAA GAC TGG TCT-N40-GCC CTA AAT ACG AGC AAC-3′ (76-mers), forward primer: 5′-GCG GAT GAA GAC TGG TCT-3′ and biotinylated reverse primer: 5′-Bio-GTT GCT CGT ATT TAG GGC-3′.
Synthesis and identification of N-fluoroacetyl glycine
The detailed synthetic pathway of N-fluoroacetyl glycine, an analog of fluoroacetamide is shown in Fig. (B). (a). Synthesis of fluoroacetyl chloride (Molecule 1 in Fig. (B)): Sodium fluoroacetate (4.5 g, 45 mM) was added to a three-neck round-bottom flask. Then phosphorus pentachloride (11 g, 53 mM) was slowly added under magnetic stirring and the mixture was heated in oil bath till the effluent comes out at about 78 °C. The distillate at 89 °C was collected to give a yield of 3.2 g of fluoroacetyl chloride (73.7%). (b). Synthesis of glycine methyl ester hydrochloride (molecule 2 in Fig. (B)): Glycine (3.0 g, 40 mM) dissolving in methanol (15 mL) and thionyl chloride (5.2 g, 44 mM) were reacted with stirring at room temperature for about 1 h. The product was filtered, washed with methanol to yield white solid (4.5 g, 80.0%). The crude was used for the next step directly. (c). Synthesis of methyl 1-fluoracetamide acetate (molecule 3 in Fig. (B)): Molecule 1 (0.97 g, 10 mM) was added slowly under stirring to a solution of molecule 2 (1.26 g, 10 mM) and triethylamine (2.02 g, 28 mM) in chloroform (10 mL) in a 100-mL three-neck round-bottom flask. The mixture was allowed to react at room temperature for 6 h. The product was concentrated, diluted with water, and extracted twice with ethyl acetate. The organic layers were combined, washed with brine, dried with Na2SO4, and concentrated to afford colorless oil (0.5 g, 3.3%). (d). Synthesis of N-fluoroacetyl glycine (molecule 4 in Fig. (B)): Molecule 3 (0.9 g, 6 mM) and sodium hydroxide (0.48 g, 12 mM) were dissolved in ethanol (15 mL) in a 100-mL three-neck round-bottom flask. The solution was refluxed for about 5 h. The product was concentrated, diluted with water, and acidified to pH 5. The white solid was precipitated out by evaporation. 1 mL of water was added and the solution was filtered to remove sodium chloride. The filter residue was dried under infrared lamp to obtain white solid (0.17 g, 21.0%). The final product was characterized by 1H NMR spectroscopy performed with a Bruker AVANCE 300 instrument (1H 300 MHz) in DMSO-d6 with tetramethylsilane ((CH3)4Si, TMS) as the internal reference. The chemical shifts were determined relative to TMS, which was set at δ 0.0.
Generation of N-fluoroacetyl glycine-BSA conjugate
N-fluoroacetyl glycine was conjugated with BSA by N-hydroxysuccinimide (NHS)-active ester method. Briefly, a solution of 1-ethyl-3-[3-dimethylaminopropyl] carbodiimide (EDC, 2 mg/mL) and N-hydroxysuccinimide (NHS, 5 mg/mL) in 0.01 M phosphate buffer (PBS) was added to N-fluoroacetyl glycine (1 mg/mL) and mixed for 6 h at room temperature. The activated N-fluoroacetyl glycine was added to BSA solution (10 mg/mL), and allowed to react for 12 h at room temperature. At the end of reaction, dialysis was performed for three days with nine changes of 0.01 M PBS buffer.
SELEX procedure
N-fluoroacetyl glycine-BSA conjugate was coated on a microplate with PBS buffer overnight. The coated wells were washed three times with washing solution (binding buffer + 0.05% Tween 20) and blocked with 3% BSA for 2 h at 37 °C. Control plate was coated with 3% BSA for 2 h. Prior to selection, the ssDNA library was heated to 95 °C in binding buffer (20 mM Tris-HCl (pH 7.4), 137 mM NaCl, 5 mM KCl, 1 mM MgCl2, 2 mM CaCl2) for 10 min, followed by immediate chilling on ice for 5 min. The denatured DNA library was firstly incubated for 1 h in the control plate to remove ssDNA bound to BSA. Subsequently, the unbound ssDNA was transferred to a N-fluoroacetyl glycine-BSA conjugate-coated plate and incubated for 1 h at 37 °C. Then the microplate was washed three times with washing solution. The DNA bound to the toxicant-protein conjugate was eluted with 50 μL of deionized water each well at 95 °C for 15 min.
PCR and Generation of ssDNA
The selected ssDNA was amplified by PCR with the forward and biotinylated reverse primers. The PCR was performed in a final volume of 50 μL that contained 25 μL of Host TaqMaster Mix (TianGen), 1 μL of each oligonucleotide primer (1 μM), and an appropriate amount of template ssDNA. The PCR conditions were initial heat denaturation at 95 °C for 3 min, 25 cycles of 95 °C for 30 s, 55 °C for 30 s, 72 °C for 30 s, and an extension step at 72 °C for 3 min. After amplification, the PCR product was electrophoresed on a 2.0% agarose gel (Sigma) with a 50 bp DNA ladder (TaKaRa). The agarose gel containing the target DNA fragment was excised and purified using GenElute™ Gel Extraction Kit (Sigma) according to manufacturer instructions. Then, the purified product was separated using streptavidin-coated magnetic beads (Sigma) to remove the biotin-labeled strands. In brief, 50 μL of the PCR product was incubated with prewashed beads (2–10 mg) for 1 h using gentle rotation. The coupled beads were washed three times with binding & washing buffer (5mM Tris-HCl(pH 7.5), 0.5mM EDTA, 1M NaCl). Then alkaline denaturation was performed with 100 μL of freshly prepared 0.15 M NaOH for 15 min. The supernatant was neutralized by HCl. The obtained ssDNA was applied as the enriched pool for the subsequent round of selection.
Affinity analysis with an SPR sensor
The increase in affinity of the selected library from each round (round 1 to 10) was assessed by SPR analysis, performed on a BI-3000 SPR system (Biosensing Instrument). The system was operated at 25 °C in running buffer (PBS) at a flow rate of 40 μL/min. All the buffer for experiments was filtered through a 0.22 μm filter and degassed before use.
The sensor chip with a thin film of gold (Biosensing Instrument) was functionalized by coating a self-assembled monolayers (SAMs). 2 mM of cystamine dihydrochloride solution (Sigma) was cast onto the Au film overnight at room temperature away from light. The surface was rinsed with water and dried with N2. Then carboxylate dextran (Sigma) in a solution (200 μL) made of 15 mM NHS and 75 mM EDC mixed by 1:1 ratio was injected and allowed to react for 3 h to activate the carboxylic acid groups. In this state, the modified sensor surface is accessible for the amino groups of carrier proteins (BSA) to bind.
For the sample channel, N-fluoroacetyl glycine-BSA was immobilized on the chip, followed by injection of 1 mM ethanolamine to block unreacted NHS esters. Immobilization was confirmed by an increase in resonance units (RU). The reference channel was treated with EDC/NHS activation and ethanolamine blocking. The selected aptamers (round 1 to 10) were injected, respectively, over the surface for 270 s at a flow rate of 40 μL/min. Continuous analysis of samples was achieved by regenerating the sensing surface with 40 mM NaOH after each cycle. The initial ssDNA library served as negative control.
Cloning and Sequencing of ssDNA Aptamers
After the last round of selection, ssDNA was amplified with the unmodified reverse primer (5′- GCG GAT GAA GAC TGG TCT-3′), and cloned into pMD® 19-T (TaKaRa), a T-A cloning-based vector, according to manufacturer instructions. The ligated plasmids were transformed into competent Escherichia coli (DH5α) (Qiagen). 54 recombinant clones were picked for analysis and sequenced by Life Technologies.
The multiple sequences of these aptamers were analyzed by DNA alignment with DNAMAN software. The secondary structures of ssDNA were predicted by web-based Mfold program, which is based on free energy minimization algorithm.Citation21)
Assays for aptamers binding to surface-bound N-fluoroacetyl-glycine-BSA
The aptamers binding to fluoroacetamide was evaluated by SPR assay. Aptamer 1-1 and 6-1 were synthesized by Life Technologies. (See Table for sequences). They were diluted in PBS to give a series of concentrations (4, 6, 8, 10, and 12 μM) and injected over the gold film surface for 270 s at a flow rate of 40 μL/min. After each cycle, the sensing surface was regenerated with 40 mM NaOH. Difference in resonance units (RU) where reference channel (flow cell two) was subtracted from sample channel (flow cell one) was measured with an increase in concentration of aptamer 1-1 and 6-1. The relationship between the concentration of the aptamer and the response was plotted. The equilibrium dissociation constant (KD) was calculated by BI-KA program (Biosensing Instrument).
Table 1. Sequences of the 40nt core regions of the cloned aptamers.
Assays for aptamers binding to free fluoroacetamide
The binding ability of the aptamer to free fluoroacetamide was determined by competitive inhibition assay. In this case, free fluoroacetamide behaves as a kind of inhibitor to the reaction between the aptamer and N-fluoroacetyl glycine-BSA conjugate. The fluoroacetamide solution prepared with PBS buffer was mixed with an equal volume of aptamer 1-1 or 6-1 (5 μM) and incubated for 30 min. The final concentrations of fluoroacetamide in solution were 0, 1.06, 3.12, 6.25, 12.5, 25, 50, 100, 200 nM. The mixture solution was injected over the sensor surface immobilized N-fluoroacetyl-glycine-BSA. The changes of binding response were measured as a result of inhibition by free fluoroacetamide. The regeneration of the conjugate surface was performed with 40 mM NaOH after each cycle to dissociate the bound aptamer. The relationship between the concentration of free fluoroacetamide and the response was plotted.
Determination of the specificity of the aptamer by a dipstick assay
The specificity of the aptamer was examined using a test paper based on lateral-flow technology. The aptamer-based fluorescent latex particles were prepared as detection probe. Briefly, streptavidin-coated fluorescent latex particles (ThermoFisher, 300 nm) dispersed in 0.05 M MES buffer (pH 6.0) was mixed with 50 μM of the biotinylated aptamer1-1 (without reverse primer) under ultrasound. After incubation at 37 °C for 2 h, the conjugated particles were blocked with ethanolamine (0.1 M) and Casein Block (0.5% Casein-Na, 0.1 M Bis-Tris, pH 8.5) in turn. The test paper consisted of four overlapping pads including absorbent pad, nitrocellulose membrane (CN140, Sartorius), glass fiber conjugate pad, and sample pad placed on the PVC backing. 2% aptamer conjugated fluorescent particles diluted with buffer A (200 mM Tris-HCl, 137 mM NaCl, 100 mM KCl, 100 mM (NH4)2SO4, 20 mM MgSO2, 4 mM CaCl2, 2 mM MgCl2, 2% Tween 20, 1% SDS, 0.5% PVP) were spotted on the conjugate pad, and 50 μM of the complementary sequence was immobilized on the membrane to form test line. The loaded test paper was dried overnight at 37 °C and cut into strips with the width of 4 mm before use.
The sample pad was immersed in buffer A containing 30 mg/mL fluoroacetamide to allow liquid to migrate upward along the membrane. After reaction, the fluorescence intensity of test line was measured by a time-resolved fluorometer. Chloroacetamide, iodoacetamide, and trifluoroacetamide, the structurally similar molecules to fluoroacetamide, were treated under the same process. Buffer A without any target was used as negative control. Each target molecules should be tested three times.
Results and discussion
Synthesis of N-fluoroacetyl glycine as a fluoroacetamide analog
Fluroacetamide (Mw = 77.06) is a very small molecule so that it can’t be directly immobilized on a solid phase support. In this study, we resolved the difficulties in screening the aptamer to low molecular weight target by synthesizing the toxicant-protein conjugate. N-fluoroacetyl glycine as a fluoroacetamide analog was initially prepared and covalently coupled to the carrier protein (BSA). As such, fluoroacetamide-BSA complex could be immobilized on the microplate surface. The synthesis route of N-fluoroacetyl glycine is shown in Fig. (B). After each reaction step, the intermediate product was purified and confirmed by NMR spectroscopy. Fig. clearly confirms the identity and structure of N-fluoroacetyl glycine. 1H NMR (300 MHz, DMSO-d6) δ ppm: 8.46 (m, 1H, NH); 4.97–4.73 (s + s, 2H, FCH2−); 3.80 (d, 2H, –CH2−). The data demonstrated that N-fluoroacetyl glycine was successfully synthesized.
Selection of aptamers
To remove ssDNA bound either to BSA or to the solid support, counter-selection was necessary to be conducted before each round of selection. The SELEX procedure outlined in Fig. was used to develop the aptamers targeted to fluoroacetamide. Overall, total procedure included three steps. In step one, the aptamer library was incubated with immobilized N-fluoroacetylglycine-BSA to form the complexes. In step two, the unbound and weakly bound oligoes were washed off and the oligoes specifically binding the target were eluted from the N-fluoroacetylglycine-BSA immobilized surface by heating. Following PCR amplification and strand separation, the resulting enriched ssDNA library was used for the next round of selection. In step three, PCR product from the final round was cloned to plasmid vector and DNA sequences were characterized.
The quality and size of the PCR products from each round were monitored by a gel electrophoresis using a 2.0% agarose gel. As shown in Fig. , the expected DNA bands at 76 bp were observed in lane 1-10 of the gel. With increase in rounds, the band became increasingly clear, indicating that the selected aptamer specifically binding the target molecule was constantly enriched.
Fig. 4. Analysis of PCR products from each round of SELEX enrichment. PCR products were sampled after each round of enrichment and run on a 2% agarose gel.
Notes: M: molecular ladder (50–500 bp), Lanes 1-10: PCR products from enrichment rounds 1-10, and lane 11: no template control.
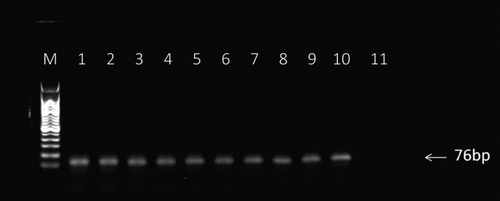
The selected aptamer from each round was injected over the N-fluoroacetylglycine-BSA immobilized sensor chip to evaluate the affinity. The response signal (in RU) gradually increased from about 20.54 RU in the first round to 68.35 RU in the 9th round and the value didn’t continue to increase in the 10th round(Fig. ). The results suggested that the affinity of ssDNA to target was improved via repeated selection steps and the ssDNA had been maximally enriched after the 9th round. After the 10th round, the eluted ssDNA was amplified by PCR with regular, nonbiotinylated primers to facilitate subsequent molecular cloning.
Sequence and structure analysis
The core region sequences of 54 clones are shown in Table . They were classified into three different groups on the basis of sequence similarity. Within Group I, 43 sequences were identical (79.6%), and the other five sequences only had a few bases variations against it. The sequences within Group II contained 30 bases that were shorter than the length of normal selected aptamer, which may be caused by PCR mutations. This phenomenon which also appeared in some other studies couldn’t be fully explained.Citation22,23) The sequences that showed homologous neither to Group I and II nor to each other were incorporated into Group III. The multiple sequence alignment was performed using DNAMAN that allowed for gaps to find potential motifs among the sequences (Fig. ).
Fig. 6. Multiple sequence alignments of the selected aptamers (without primers).
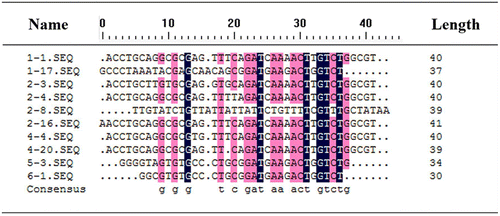
The secondary structures of two representative sequences (1-1 in Group I and 6-1 in Group II) were predicted using Mfold software. The structures with minimum free energy of each sequence were chosen for further study (Fig. ). It was observed that the stem-loop structure was the major motif, which was reported in many literatures as one of the most widespread structural element for target recognition.Citation11,22) The predicted structures for both 1-1 and 6-1 involved a similar section containing a two-loop hairpin structure located on a stem, where the consensus sequence was included. The consensus sequence could play an important role in binding with the target. In addition, a G-rich region was found in the two-loop hairpin of aptamer 6-1, which was possibly folded into a G-quadruplex structure. This structure was considered to increase the stability of the aptamer-target complex.Citation24) The secondary structures of the other aptamers in Group III were also predicted (Fig. ). They were greatly different from the structures of aptamers in Group I and II. In view of the frequency and the structural similarity, the aptamer 1-1 and 6-1 were used for the following investigation.
Validating the interaction between aptamers and N-fluoroacetyl glycine-BSA
To simplify the sequence, we synthesized the core regions of aptamer 1-1 and 6-1 without the primers that could be useless for target recognition. Their binding ability to N-fluoroacetyl glycine-BSA was evaluated by SPR assay. Fig. shows the correlation between the change in RU and the concentration of aptamer 1-1 and 6-1. The response signal raised with an increase in concentration, which resulted from the binding interaction between these aptamers and the N-fluoroacetyl glycine-BSA surface. The BSA immobilized sensor surface that was prepared as described in above method was used to be a negative control. When aptamer 1-1 and 6-1 were exposed to the BSA immobilized surface, no change in response was detected until the concentration reached up to 12 μM (Fig. ). The result indicated that the aptamers didn’t exhibit specific binding to BSA after the counter-selection step. The KD values of aptamer 1-1 and 6-1 were measured at 1 and 1.2 μM, respectively, which were comparable to those of some published aptamers.Citation22,25–27) Taken together, these data suggested that aptamer 1-1 and 6-1 specifically bind to N-fluoroacetyl glycine-BSA.
Validating the interaction between aptamers and free fluoroacetamide
To demonstrate the aptamers have the ability to bind to free fluoroacetamide, we developed a competitive inhibition assay. After pre-incubation with free fluoroacetamide in solution, aptamer 1-1 or 6-1 was flowed over N-fluoroacetyl glycine-BSA surface. The response signal was sensitive to the variation of free fluoroacetamide concentration (Fig. ). With increasing concentration, less and less binding of the aptamer to the sensor surface was observed due to the inhibition effect of free fluoroacetamide. In another word, the aptamers were bound to free fluoroacetamide in solution instead of binding to the fluoroacetamide-BSA surface. The results showed that the selected aptamers have the abilities to bind not only the surface-immobilized fluoroacetamide but also free fluoroacetamide, and the binding of the aptamers to fluoroacetamide exhibits concentration dependence.
Specificity of the aptamer
To verify the specificity of the selected aptamer, a lateral-flow test strip was developed. In the absence of fluoroacetamide, the aptamer-fluorescent latex complex was captured by the complementary sequence on the test line, where a brilliant pink band was observed under 365-nm ultraviolet light. In the presence of fluoroacetamide, the band gradually dimmed until disappearance with increasing fluoroacetamide concentration due to the competitive inhibition effect between fluoroacetamide and the complementary sequence (data not shown). As shown in Fig. , the fluorescence intensity of fluorescent latex particles captured at the test zone significantly lowered when dipping the test strip into the solution containing fluoroacetamide. In contrast, relatively small change of intensity was measured for the other three molecules. The results indicated that the aptamer 1-1 has good affinity to fluoroacetamide, but not to other similar molecules.
Fig. 10. Characterization of specificity of aptamer 1-1 for fluoroacetamide. The binding affinity of aptamer 1-1 to fluoroacetamide and other structurally similar molecules was tested by a dipstick assay based on lateral-flow technology. The fluorescence intensity at test line for each target was measured. The buffer solution without any target was used as blank.
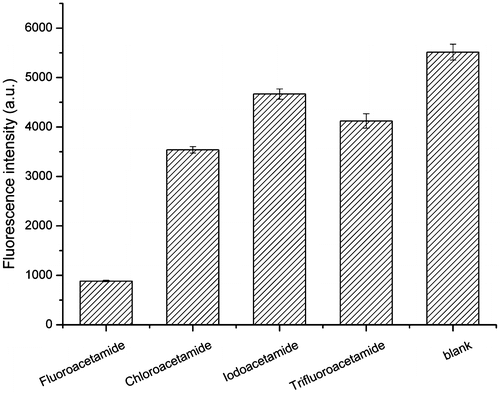
Conclusions
By using the SELEX strategy, we have succeeded in developing two DNA aptamers that specially recognize the small molecule toxicant fluoroacetamide. The equilibrium dissociation constant (KD) values were obtained by SPR assay at 1 and 1.2 μM, respectively, which demonstrates a good affinity of the selected aptamers to the target. In this study, microplates were used as solid matrixes to separate the target-bound and unbound oligonucleotides from a random ssDNA library, and the N-fluoroacetyl glycine-BSA conjugate was developed for SELEX to overcome the difficulty in immobilization of small molecules to the solid matrix. Our results suggest that this SELEX process is convenient, rapid and efficient for aptamer selection and can be extended to discover aptamers targeted to other low molecular weight toxicants. In addition, we have applied a SPR-based inhibition assay to investigate the binding ability of the selected aptamers to the free fluoroacetamide in this work. The result showed that the response value was correlated to the concentration of fluoroacetamide. Thus, this assay will possibly provide a viable alternative to conventional analytical methods such as GC/MS and LC/MS for semi-quantitative detection of small molecule toxicants.
Since aptamers can be produced without in vivo animal immunization, they are considered as promising recognition elements for poison detection instead of antibodies. Combined with some other analytical technologies such as fluorometry and electrochemical method, aptamer technology can be applied for poison rapid screening on the scene, which has high potential application in the fields of food safety, environmental monitoring, and forensic science. In this work, a simple lateral-flow test strip was preliminarily developed to determine the specificity of the aptamer, and on this basis it is hoped that an aptamer biosensor for rapid detection of fluoroacetamide will be further developed through optimization.
Disclosure statement
No potential conflict of interest was reported by the authors.
Acknowledgments
The authors would like to thank Dr. N. Sang (Drexel University, Philadelphia, USA) for reviewing the manuscript prior to submission, and Dr. Xian Ye (Chinese Academy of Science & Shanghai Institute of Materia Medica, Shanghai, China) for NMR analysis of synthesized N-Fluoroacetyl glycine.
References
- Shen M, Xiang P. Forensic toxicology handbook. Beijing: Science Press; 2012. p. 256.
- Xu X, Song G, Zhu Y, et al. Simultaneous determination of two acute poisoning rodenticides tetramine and fluoroacetamide with a coupled column in poisoning cases. J. Chromatogr. B. 2008;876:103–108.10.1016/j.jchromb.2008.10.027
- Logan BK, Stafford DT, Tebbett IR, et al. Rapid screening for 100 basic drugs and metabolites in urine using cation exchange solid-phase extraction and high-performance liquid chromatography with diode array detection. J. Anal. Toxicol. 1990;14:154–159.10.1093/jat/14.3.154
- Cai X, Zhang D, Ju H, et al. Fast detection of fluoroacetamide in body fluid using gas chromatography-mass spectrometry after solid-phase microextraction. J. Chromatogr. B. 2004;802:239–245.10.1016/S1570-0232(03)00556-7
- Feng SZ, Yu ZS. Analysis of fluoroacetamide in biological samples with GC/NPD. GC/FID. Forensic Med. 1999;15:91–92.
- Godal A, Olsnes S, Pihl A. Radioimmunoassays of abrin and ricin in blood. J. Toxicol. Environ. Health. 1981;8:409–417.10.1080/15287398109530079
- Ellington AD, Szostak JW. In vitro selection of RNA molecules that bind specific ligands. Nature. 1990;346:818–822.10.1038/346818a0
- Tuerk C, Gold L. Systematic evolution of ligands by exponential enrichment: RNA ligands to bacteriophage T4 DNA polymerase. Science. 1990;249:505–510.10.1126/science.2200121
- Stoltenburg R, Reinemann C, Strehlitz B. SELEX—A (r)evolutionary method to generate high-affinity nucleic acid ligands. Biomol. Eng. 2007;24:381–403.10.1016/j.bioeng.2007.06.001
- Darmostuk M, Rimpelova S, Gbelcova H, et al. Current approaches in SELEX: An update to aptamer selection technology. Biotechnol. Adv. 2015;33:1141–1161.10.1016/j.biotechadv.2015.02.008
- Handy SM, Yakes BJ, DeGrasse JA, et al. First report of the use of a saxitoxin-protein conjugate to develop a DNA aptamer to a small molecule toxin. Toxicon. 2013;61:30–37.10.1016/j.toxicon.2012.10.015
- Szeitner Z, András J, Gyurcsányi RE, et al. Is less more? Lessons from aptamer selection strategies. J. Pharmaceut. Biomed. 2014;101:58–65.10.1016/j.jpba.2014.04.018
- Tombelli S, Minunni M, Mascini M. Aptamers-based assays for diagnostics, environmental and food analysis. Biomol. Eng. 2007;24:191–200.10.1016/j.bioeng.2007.03.003
- Wandtke T, Woźniak J, Kopiński P. Aptamers in diagnostics and treatment of viral infections. Viruses. 2015;7:751–780.10.3390/v7020751
- McConnell EM, Holahan MR, DeRosa MC. Aptamers as promising molecular recognition elements for diagnostics and therapeutics in the central nervous system. Nucleic Acid Ther. 2014;24:388–404.10.1089/nat.2014.0492
- Que-Gewirth NS, Sullenger BA. Gene therapy progress and prospects: RNA aptamers. Gene Ther. 2007;14:283–291.10.1038/sj.gt.3302900
- Liu JW, Mazumdar D, Lu Y. A simple and sensitive “dipstick” test in serum based on lateral flow separation of aptamer-linked nanostructures. Angew. Chem. Int. Ed. 2006;45:7955–7959.10.1002/(ISSN)1521-3773
- Maehashi K, Katsura T, Kerman K, et al. Label-free protein biosensor based on aptamer-modified carbon nanotube field-effect transistors. Anal. Chem. 2007;79:782–787.10.1021/ac060830g
- Hayat A, Mishra RK, Catanante G, et al. Development of an aptasensor based on a fluorescent particles-modified aptamer for ochratoxin A detection. Anal. Bioanal. Chem. 2015;407:7815–7822.10.1007/s00216-015-8952-3
- Ravelet C, Grosset C, Peyrin E. Liquid chromatography, electrochromatography and capillary electrophoresis applications of DNA and RNA aptamers. J. Chromatogr. A. 2006;1117:1–10.10.1016/j.chroma.2006.03.101
- Zuker M. Mfold web server for nucleic acid folding and hybridization prediction. Nucleic Acids Res. 2003;31:3406–3415.10.1093/nar/gkg595
- Kim YS, Hyun CJ, Kim IA, et al. Isolation and characterization of enantioselective DNA aptamers for ibuprofen. Bioorg. Med. Chem. 2010;18:3467–3473.10.1016/j.bmc.2010.03.074
- Masud MM, Kuwahara M, Ozaki H, et al. Sialyllactose-binding modified DNA aptamer bearing additional functionality by SELEX. Bioorg. Med. Chem. 2004;12:1111–1120.10.1016/j.bmc.2003.12.009
- Kulbachinskiy AV. Methods for selection of aptamers to protein targets. Biochemistry (Moscow). 2007;72:1505–1518.10.1134/S000629790713007X
- Majerfeld I, Puthenvedu D, Yarus M. RNA affinity for molecular L-histidine; genetic code origins. J. Mol. Evol. 2005;61:226–235.10.1007/s00239-004-0360-9
- Mannironi C, Di Nardo A, Fruscoloni P, et al. In vitro selection of dopamine RNA ligands. Biochemistry. 1997;36:9726–9734.10.1021/bi9700633
- He J, Liu Y, Fan M, et al. Isolation and identification of the DNA aptamer target to acetamiprid. J. Agric. Food Chem. 2011;59:1582–1586.10.1021/jf104189g