Abstract
Plants produce a host of secondary metabolites with a wide range of biological activities, including potential toxicity to eukaryotic cells. Plants generally manage these compounds by transport to the apoplast or specific organelles such as the vacuole, or other self-tolerance mechanisms. For efficient production of such bioactive compounds in plants or microbes, transport and self-tolerance mechanisms should function cooperatively with the corresponding biosynthetic enzymes. Intensive studies have identified and characterized the proteins responsible for transport and self-tolerance. In particular, many transporters have been isolated and their physiological functions have been proposed. This review describes recent progress in studies of transport and self-tolerance and provides an updated inventory of transporters according to their substrates. Application of such knowledge to synthetic biology might enable efficient production of valuable secondary metabolites in the future.
Graphical abstract
Uses of tolerance and transport proteins for efficient production of valuable compounds in microorganisms.
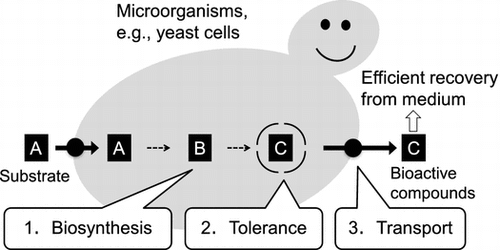
Plants have developed sophisticated processes to enhance their survival, including the production of various secondary metabolites.Citation1) These compounds serve diverse purposes, such as in defense responses against herbivores and pathogens, and in protection against ultraviolet light. Many secondary metabolites have biological activities useful for humans, including uses as medicinal compounds. To produce such bioactive compounds stably and at high concentrations in plant cells, cultured cells that produce high levels of secondary metabolites have been established.Citation1,2) In addition, many studies have investigated the biosynthetic enzymes and genes for secondary metabolites. Some studies have reported production of valuable metabolites using cultured cells or transgenic plants over-expressing biosynthetic enzyme genes,Citation2,3) but these successes remain limited.
Some secondary metabolites, especially alkaloids, are toxic to prokaryotic and eukaryotic cells. For example, berberine, a benzylisoquinoline alkaloid produced in some plant species inhibits the biosynthesis of DNA and protein in bacteria and functions as a chemical protectant against bacteria. These compounds also are toxic to plant cells that do not produce them; interestingly, however, plant cells producing such metabolites are often insensitive to them, suggesting the existence of self-tolerance mechanisms. One important mechanism plants use to prevent cytotoxicity of metabolites is excretion of those metabolites from the cytosol to the apoplast or to organelles such as the vacuole.Citation4) This transport reduces the cytosolic concentration of active compounds, resulting in a decrease in cytotoxicity. In addition, biosynthetic intermediates move at the intra- and inter-cellular levels. Therefore, transport systems likely participate in the efficient biosynthesis of these metabolites. In addition, other mechanisms such as modification of metabolites to less-toxic forms, also likely act in self-tolerance.Citation4)
In contrast to the many studies of biosynthetic enzymes and genes,Citation2) for many years, little attention was paid to transport and other self-tolerance mechanisms. However, those mechanisms are now being intensively studied and recent work has identified many of the responsible proteins. Understanding the mechanisms of transport and tolerance, in addition to the mechanisms of biosynthesis, would contribute to the efficient production of valuable compounds in plant cells or via synthetic biology using microbes. This review provides an updated inventory of the known transporters of secondary metabolites and briefly summarizes current knowledge of self-tolerance mechanisms in plants.
I. Membrane transport of secondary metabolites
Most secondary metabolites are stored in the vacuole or excreted to the apoplast in the cell. In addition, some alkaloids, such as berberine and nicotine, are translocated from the source organ (biosynthesis) to a sink organ (accumulation). Various studies have proposed different mechanisms for this movement, such as simple diffusion, vesicle-mediated transport, and transporter-mediated membrane transport. The identified transporter proteins for secondary metabolites can be roughly classified into 4 families: the ABC (ATP-binding cassette) transporter,Citation5–7) NRT (nitrate-peptide transporter),Citation8,9) MATE (multidrug and toxic compound extrusion)Citation7,10) and PUP (purine permease)Citation11) families.
These transporter families have a large numbers of members and also play important roles in the transport of primary metabolites and plant hormones. For instance, the Arabidopsis thaliana genome has approximately 120 genes for ABC proteins. Most ABC proteins consist of transmembrane domains (TMDs) and nucleotide binding domains (NBDs). From the topology of those sub-domains, plant ABC proteins are classified into 8 subfamilies (ABCA-ABCG and ABCI; ABCH is absent in plants). In animals, ABC proteins have multiple functions, e.g. as transporters, channels, and channel regulators. ABC transporters export or import their substrates using energy obtained from ATP hydrolysis. In plants, the repertory of substrates includes primary metabolites, hormones, and heavy metals, and these transport activities are relevant for various physiological functions throughout plant development.Citation5–7) As for the NRT family, the Arabidopsis thaliana genome has 53 NRT genes. NRTs were originally identified as transporters for nitrate or peptide, and probably function as proton symporters. Recent progress expanded the repertory of NRT substrates to glucosinolates and plant hormones.Citation8,9) Most plant MATE transporters have 12 TMDs and are presumed to function as proton antiporters. The Arabidopsis thaliana genome has 56 MATE genes and some plant MATE transporters act in xenobiotic efflux, Fe translocation, Al detoxification, and hormone signaling.Citation7,10) The Arabidopsis thaliana genome has 21 PUP genes. Most PUPs have 10 TMDs and influx their substrates probably as proton symporters. PUP substrates include purine ring-containing compounds like adenine, cytosine, and cytokinins, and pyridine ring-containing compounds like pyridoxine and pyridoxal.Citation11) Previous reviews have provided some information on the transport mechanisms for some group of secondary metabolites, identifying specific transporters and defining some specific substrates, mainly for alkaloids, flavonoids, glucosinolates.Citation9,12–18) This review summarizes the current understanding of these transporters, based on their substrates (Table ).
Table 1 Transporters involved in the movement of secondary metabolites in plants.
I.i. Alkaloids
Alkaloids are low-molecular weight compounds that contain nitrogen. Most alkaloids have strong biological activities and presumably function as defense compounds in planta. Some have medicinal uses, e.g. vincristine and vinblastine from Catharanthus roseus for anti-cancer medicine, and morphine from opium poppy (Papaver somniferum) for analgesics.Citation1) The biosynthesis of some alkaloids occurs in multiple organelles within a cell, as well as multiple tissues within an organ. Further, some alkaloids are translocated from source organ to sink organ; therefore, intra-cellular, inter-cellular, and inter-organ transport have been suggested to be important in alkaloid biology.
In C. roseus, 2 major valuable vinca alkaloids, vinblastine and vincristine, are biosynthesized via the dimerization of the monomeric monoterpenoid indole alkaloids catharanthine and vindoline. The biosynthetic intermediates move dynamically among organelles (intra-cellular transport) and cells (inter-cellular transport) during vinca alkaloid biosynthesis.Citation19,20) CrTPT2 is a plasma-membrane localized G-type ABC transporter responsible for the efflux of catharanthine to the leaf surface, where it plays a role in protection of plants against herbivores.Citation21) By contrast, vindoline accumulates in internal leaf cells and occasionally in mesophyll vacuoles via a proton/alkaloid antiporter.Citation22) This spatial separation of catharanthine and vindoline limits the rate of production of dimeric monoterpenoid indole alkaloids. Down regulation of CrTPT2 by virus-induced gene silencing decreases the catharanthine level at the leaf surface and increases the catharanthine available for synthesis of dimers in internal leaf cells, leading to an increase in the dimers of catharanthine and vindoline. This result shows the possibility for high-level production of valuable compounds by transport engineering.Citation21)
Coptis japonica, a medicinal plant used in Asian countries, produces the alkaloid berberine in the root and translocates it to the rhizome, which is therefore used as the medicinal part of this plant.Citation23) Biochemical analysis suggested that B-type ABC transporters act in berberine transportCitation24,25) and 3 ABCB transporters, CjABCB1 (CjMDR1), CjABCB2, and CjABCB3 have been isolated and characterized.Citation26–28) CjABCB1 and CjABCB2 are involved in berberine translocation from the root to the rhizome by transporting berberine from xylem vessels to the cytoplasm at the plasma membrane of cells around vascular bundles in rhizome.Citation27–29) Berberine accumulates in the vacuole in cultured cells and probably also cells of the rhizome, and a proton/berberine antiport system was suggested to be responsible for this vacuolar transport.Citation30)
In Nicotiana tabacum, nicotine is biosynthesized in roots and translocated to aerial parts via the xylem.Citation31,32) Nicotine accumulates in leaf vacuoles at high concentrations, and plays an important role in defense against herbivores,Citation33) indicating the importance of nicotine translocation for defense. Five nicotine transporters have been identified. The MATE transporters jasmonate-inducible alkaloid transporter 1 (JAT1), JAT2, and MATE1 show nicotine transport activities, probably acting as nicotine/proton antiporters and localize to the vacuolar membrane. JAT1 and JAT2 likely transport nicotine into leaf vacuolesCitation34–37); MATE1 and MATE2 likely transport nicotine into root vacuoles.Citation38) Nicotine uptake permease1 (NUP1), a member of the PUP family, mediates nicotine influx at the plasma membrane of cells of root tips, the main site of nicotine biosynthesis.Citation39,40) Interestingly, NUP1 also regulates nicotine metabolism and translocation.Citation39,41,42) At the flowering stage, benzyl acetone attracts pollinators and nicotine repels pollinators; this balance affects the rates of outcrossing and seed production.Citation43,44) High levels of JAT1 in flower tissues suggest the involvement of this protein in these important reproductive processes by transporting nicotine in flowers.Citation37)
For further detailed information about alkaloid transport, please refer to previous reviews.Citation45,46)
I.ii. Phenolic compounds
Phenolic compounds have at least one aromatic ring with one or more hydroxyl substituents, and consist of several subgroups, e.g. phenylpropanoids, lignans, flavonoids, and condensed tannins (polymers of flavonoids). Flavonoids have several important roles in plants, serving as signaling molecules for plant-microbe symbiosis, attracting pollinators by influencing flower color, and protecting cells against ultraviolet light stress.Citation47) To carry out these functions, phenolics must be secreted to the apoplast or accumulate in vacuoles and some transport mechanisms, i.e. vesicle-dependent movement, ABC transporter-dependent transport, MATE transporter-dependent transport, and glutathione S-transferase (GST)-involved transport, have been described previously.Citation17,18,48)
The involvement of C-type ABC transporters in vacuolar anthocyanin accumulation was proposed based on the finding that a mutant defective in GST was unable to accumulate anthocyanin in vacuoles,Citation49) as glutathione conjugates are the preferred substrates of the human C-type ABC (MRP; multidrug resistance-associated protein) transporter. Genetic analysis in maize supported this hypothesis; suppression of ZmMRP3, a tonoplast-localized C-type ABC transporter, significantly decreased the anthocyanin level in leaves and husks.Citation50) Biochemical analysis of VvABCC1 of grapevine (Vitis vinifera) proved that this C-type ABC transporter mediates co-transport of glucosylated anthocyanidin, malvidin 3-O-glucoside with glutathione (GSH) in an ATP-dependent manner.Citation51) Since this transporter localizes to the tonoplast and is expressed in the exocarp, where most anthocyanins accumulate at high concentrations during berry development and ripening, VvABCC1 likely acts in vacuolar accumulation of anthocyanins in grape berry.
MATE transporters are also involved in anthocyanin transport. Citation10) MTP77 was isolated from Solanum lycopersicum as a candidate for flavonoid transport.Citation52) Two grapevine MATEs, VvAM1 and VvAM3, also called anthoMATEs, function in anthocyanin accumulation in small vesicles that actively move alongside the tonoplast.Citation53,54) In Medicago truncatula, MATE2 transports malonylated flavonoid glucosides into vacuoles, and is involved in flower coloration and anthocyanin pigmentation in leaves.Citation55) In addition, Arabidopsis thaliana FLOWER FLAVONOID TRANSPORTER (FFT), a MATE transporter, might participate in vacuolar anthocyanin accumulation in the seed coat.Citation56–58)
Transport of epicatechin glucoside or related compounds into the vacuole is implicated in the formation of condensed tannin, and deficiency in this transport process decreases seed coloration. AtTT12 from Arabidopsis and MtMATE1 from M. truncatula are tonoplast-localized transporters of epicatechin 3′-O-glucoside (E3′G), the precursor of condensed tannin, and are involved in proanthocyanidin biosynthesis in the seed coat.Citation59–61) Similar transport functions were proposed for VvMATE1 and VvMATE2 from V. viniferaCitation62) and MdMATE1 and MdMATE2 from apple (Malus × domestica Borkh.),Citation63) although VvMATE2 localizes to the Golgi.
With regard to other processes underlying vacuolar flavonoid accumulation, bilitranslocase transporters were also proposed to carry out vacuolar flavonoid transport.Citation64,65) Furthermore, a vesicle-mediated mechanism was suggested,Citation66) and a golgi-localized GFS9 required for membrane trafficking was identified as responsible for fusion of vesicles containing flavonoids to the vacuole.Citation67) Autophagy is also involved in anthocyanin pigment movement.Citation68) In addition to the involvement of GST in C-type ABC transporter-dependent flavonoid transport, GST is also proposed to function as a flavonoid carrier by forming a flavonoid-GST complex, which is delivered to the vacuole or vesicles.Citation18,69)
Secretion of flavonoids to the soil is a prominent process in plant-microbe interactions.Citation70,71) As a first signal for formation of a symbiosis with rhizobia, soybean roots secrete several isoflavonesCitation72) and ABC proteins were suggested to be involved in this secretion.Citation73) Flavonoid secretion also plays an important role in defense against root-infecting pathogens and MtABCG10, G-type ABC transporter from M. truncatula, might be involved in this process by modulating the isoflavone levels in roots.Citation74) Other phenolic compounds are similarly secreted to the soil in response to environmental factors, i.e. scopoletin and coumarin secretion possibly mediated by AtABCG37.Citation75)
Lignification has important roles in plant development, particularly in the vasculature. Monolignol efflux to the apoplast is necessary for lignification and is mediated by the G-type ABC transporter AtABCG29 in Arabidopsis.Citation76) By contrast, proton antiport of monolignol glucoside into endosomes was proposed to function in differentiating xylem in woody plants.Citation77)
I.iii. Glucosinolates
Glucosinolates are amino acid-derived secondary metabolites that contain sulfate and thioglucose. Glucosinolates and thioglucosidases, also called myrosinases, normally occur in separate compartments within the cell. When plants are injured, the glucosinolates come into contact with and are hydrolyzed by the thioglucosidases, resulting in the production of isothiocyanates, nitriles, and thiocyanates. These degradation products have various biological activities including deterrents and attractants, and play important roles in defense against herbivory.Citation78) Therefore, production and accumulation of glucosinolates in the appropriate tissues and cells at each developmental stage are essential for plant adaptation to the environment.
During reproductive development, glucosinolates are translocated from leaves to seeds, where glucosinolates are not produced, via phloem transport; this is accompanied by a decrease in leaf glucosinolate levels.Citation79) Functional screening of an Arabidopsis transporter cDNA library identified GLUCOSINOLATE TRANSPORTER 1 (GTR1) and GTR2.Citation8) These GTR transporters belong to the nitrate/peptide (NRT/PTR) transporter family, and both mediate influx of glucosinolate as their preferred substrate. These 2 proteins play an important role in aliphatic and indole glucosinolate translocation to seeds by loading glucosinolates into the phloem in leaves.Citation8) In the vegetative phase, some glucosinolates are distributed bidirectionally between roots and rosette leaves. Furthermore, glucosinolates are allocated within the mature leaf. GTR1 and GTR2 are also implicated in these transport processes.Citation80–82) Recent progress in understanding glucosinolate transport mechanisms was summarized previously.Citation9,16) For efficient inter-organ and inter-tissue movement of glucosinolates, plasma membrane-localized efflux transporters and tonoplast-localized transporters might exist, and further identification of such transporters is awaited. GTR1 also can transport jasmonoyl-isoleucine and gibberellin, and is involved in stamen development. Therefore, it appears to have multiple functions in plant development.Citation83)
I.iv. Terpenoids
Terpenoids, also called isoprenoids, are biosynthesized from 2 precursors, isopentenyl diphosphate (IPP) and its isomer dimethylallyldiphosphate (DMAPP). Isoprenoids are classified as monoterpenes (C10), sesquiterpene (C15), diterpenes (C20), sesterterpenes (C25), triterpenes (C30), tetraterpenes (C40, carotenoids), and steroids (C18~30). Some isoprenoids have beneficial uses including flavorings and medicines.Citation84)
As transporters for terpenoids, only G-type ABC transporters have been reported so far. Nicotiana plumbaginifolia pleiotropic drug resistance1 (NpPDR1/NpABC1) was first isolated as a plasma membrane-localized protein induced by treatment with sclareolide, a close analog of the endogenous antifungal diterpene compound sclareol.Citation85) NpPDR1 is preferentially expressed throughout the root, in the leaf glandular trichomes, and in the flower petals and is proposed to transport sclareol. Down-regulation of NpPDR1 causes susceptibility to some fungal and oomycete pathogens.Citation86,87) Therefore, NpPDR1 could be involved in pathogen resistance by transporting sclareol to the plant surface. Similar functions were proposed for SpTUR2, a Spirodela polyrrhiza protein, and AtPDR12/AtABCG40, both of which have high similarity to NpPDR1,Citation88,89) although AtPDR12/AtABCG40 also plays important roles in lead tolerance and ABA transport.Citation90,91) A diterpene transporter from N. tabacum, NtPDR1, belonging to the G-type ABC transporters and having high amino acid sequence identity with NpPDR1 (84%), was identified as an elicitor-responsive gene,Citation92) with several putative cis-elements including the GCC box and JA-responsive elements in its promoter region.Citation93) NtPDR1 also could play a key role in plant defense by transporting anti-fungal diterpenes such as sclareol, manool, cembrene, and eucalyptol.Citation94)
I.v. Cuticlar waxes and suberin
Land plants cover their aerial surfaces with a cuticle, which protects plants against water loss and thus helped plants to expand their habitat from aqueous to terrestrial environments. Cuticle is composed of cuticular waxes and cutin, which are formed from very long-chain fatty acid derivatives and cutin monomers, respectively. Those compounds are preferentially produced in the epidermal cells and then exported to the plant surface. Transport processes for these compounds have been summarized in previous reviews of cuticular wax biosynthesis, export, and regulation.Citation95–97)
Forward and reverse genetic approaches have identified many transporters involved in cuticle formation, all of which are G-type ABC transporters. A mutant in AtABCG12/CER5 shows decreased cuticular wax loads accompanied by increased cytosolic lipid inclusions.Citation98) The AtABCG12/CER5 transporter is a half-size G-type ABC transporter localized to the plasma membrane and probably functions in wax export to the cuticle by forming heterodimers with AtABCG11/COF1/DSO/WBC11.Citation99) AtABCG11 is required for secretion of cutin precursors as homodimers.Citation99–103) Similar functions are also suggested for related proteins in other plants, namely Pp-ABCG7 from the moss Physcomitrella patens,Citation104) and WBC11 from Sorghum bicolor.Citation105) Some full-size G-type ABC transporters are reported to be essential for cuticle formation and leaf water retention. HvABCG31/EIBI1 from Hordeum vulgare,Citation106–109) OsABCG31 from rice,Citation106) GL13 from Zea mays,Citation110) and AtABCG32/PEC1 from ArabidopsisCitation111,112) are involved in the formation of functional cuticle, and mutants of the respective genes in barley and maize showed high sensitivity to drought.
In flower organs, the half-size G-type ABC transporter AtABCG13 is preferentially expressed in petals and carpels, and suggested to export cuticular lipid from cells of these tissues to the outer cell matrix.Citation113) Formation of exine, a lipid barrier on the surface of pollen, is essential for normal pollen development and male fertility.Citation114) G-type ABC transporters contribute to this process. Sporopollenin lipid precursors are produced in the tapetum cells and subsequently transported into the locule, probably by plasma-membrane localized AtABCG26 in ArabidopsisCitation115–120) or OsABCG15 in rice,Citation121–125) for polymerization on developing microspores. OsABCG26 also functions in efflux of lipid molecules at the plasma membrane of tapetal cells collaboratively with OsABCG15.Citation125) AtABCG1 and AtABCG16 appear to be necessary for pollen wall integrity,Citation126) whereas AtABCG9 and AtABCG31 are essential for deposition of steryl glycosides on the pollen coat.Citation127) AtABCG9 is also involved in vascular patterning in cotyledons and the floral stem, functioning with AtABCG11 and AtABCG14.Citation128)
Suberin plays an important role in protection against desiccation and consists of complex polymers of metabolites such as glycerol, ferulic acid, and C16 and C18 fatty acids.Citation129) G-type ABC transporters participate in suberin formation. In rice, OsABCG5/RCN1 has an essential role in hypodermal suberization of roots.Citation130) Potato ABCG1 is necessary for suberin formation and normal development of the tuber, possibly by exporting suberin components.Citation131) AtABCG11, involved in cutin formation as described above, is also involved in suberin metabolism in roots.Citation132) The triple mutant of AtABCG2, AtABCG6, and AtABCG20 displays pleiotropic phenotypes including decreased suberin content in seed coats and roots with increased water and salt permeability and few lateral roots. Hence, these 3 ABCGs appear to be involved in the formation of an effective suberin barrier in seed coats and roots.Citation126)
II. Other tolerance mechanisms for secondary metabolites
In addition to efflux of secondary metabolites to the apoplast or vacuolar lumen, several species-specific tolerance mechanisms exist. These mechanisms involve biosynthesis in the extracellular space or vacuolar lumen, conversion to non-toxic forms via glycosylation, and mutation of the target proteins of the metabolites, as summarized previously.Citation4,133) For example, some metabolites are biosynthesized at the apoplast or vacuolar lumen to avoid production in the cytosol, as reported for cannabinoids and anthocyanins.Citation134,135) Toxic compounds are often converted to less-toxic forms via glycosylation, and most glycosylated compounds are stored in the vacuole.Citation4) Mutation of the target protein of the toxic compound has been reported for camptothecin, an anticancer alkaloid that induces cell death by inhibiting DNA topoisomerase activity. Topoisomerase I enzymes of camptothecin-producing plants, namely, Camptotheca acuminata, Ophiorrhiza pumila, and Ophiorrhiza liukiuensis, have point mutations that render them resistant to camptothecin, enabling these plants to produce the toxic compound stably within their cells.Citation136) Interestingly, one such mutation is identical to that found in human cancer cells that show resistance to camptothecin.Citation136) Detailed descriptions of self-tolerance mechanisms are available in recent reviews.Citation4,133)
Other proteins involved in self-tolerance were identified from functional screening using yeast cells. For example, C. japonica produces the anti-bacterial alkaloid berberine to defend against pathogens. Yeast cells transformed with a C. japonica cDNA library were screened on medium containing berberine. One cDNA, encoding galactinol synthase (CjGolS), clearly conferred berberine tolerance to yeast cells.Citation137) Similar screening with a Sophora flavescens cDNA library was performed for a prenylated flavonoid of this plant, sophoraflavanone G (SFG), which has anti-bacterial and anti-tumor activities. S. flavescens regulatory particle triple-A ATPase 2 (SfRPT2), a member of the 26S proteasome subunit, conferred SFG tolerance when expressed in yeast cells.Citation138) Although the detailed tolerance mechanisms remain unknown, these proteins could be useful for synthetic biology to enable production of secondary metabolites that are valuable but toxic, since these proteins confer tolerance to yeast cells.
III. Conclusions and future perspectives
In recent years, intensive studies have identified many molecules related to transport and self-tolerance of secondary metabolites. These molecules play important roles in both secondary metabolite biosynthesis and the exertion of their biological activity. For example, transport of condensed tannin precursor into the vacuole by AtTT12 and MtMATE1 is involved in seed coloration, and alkaloid catharanthine efflux to the leaf epidermis by CrTPT2 is involved in defense mechanisms. Notably, some plants with altered expression of transporters show significant changes in metabolite accumulation; i.e. the Arabidopsis double knockout of GTR1 and GTR2 glucosinolate transporters shows clearly diminished glucosinolate contents in seed, and down regulation of CrTPT2 increases production of dimers of catharanthine and vindoline, which are precursors of the anti-cancer compound vinblastine.Citation8,21) These findings strongly suggest the possibility of metabolic engineering of secondary metabolites, especially useful bioactive compounds, by altering the expression of transporters, as proposed for transport engineering of primary metabolites and ions.Citation139)
In addition, these tolerance and transport proteins would be useful in synthetic biology for secondary metabolite production. Biosynthesis of such bioactive compounds in micro-organisms by expressing biosynthetic enzyme genes often leads to growth retardation and low productivity, probably due to the toxicity of the products and negative feedback on the biosynthetic enzymes.Citation140) Micro-organisms harboring not only genes for biosynthesis but also genes for tolerance and transport should show tolerance to the bioactivity of the products. These microbes would also transport the product to the medium, potentially leading to high production by the micro-organism and efficient recovery from the medium (Fig. ). Further advances in this field could lead to efficient production of valuable secondary metabolites in plants or micro-organisms.
Fig. 1. Uses of tolerance and transport proteins for efficient production of valuable compounds in micro-organisms.
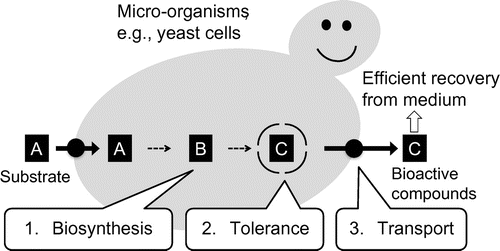
Funding
This work was supported by Japan Society for the Promotion of Science (JSPS KAKENHI) [grant number 25712012] Grant-in-Aid for Young Scientists (A) to N.S.
Acknowledgments
I am very grateful to professor Kazufumi Yazaki (Kyoto University) for his continuous support and invaluable discussion. I sincerely thank professor Fumihiko Sato (Kyoto University) for his kind guidance and encouragement, and all my past and present co-workers and laboratory members for their help and discussion. I also acknowledge Dr Akifumi Sugiyama for critical reading and comments on the manuscript. I am grateful to the Japan Society for Bioscience, Biotechnology, and Agrochemistry for its presentation to me of the Award for the Encouragement of Young Scientists.
Disclosure statement
No potential conflict of interest was reported by the author.
Notes
This review was written in response to the author’s receipt of the JSBBA Award for Young Scientists in 2015.
Abbreviations: ABC, ATP-binding cassette; GST, glutathione S-transferase; MATE, multidrug and toxic compound extrusion; MDR, multidrug resistance; MRP, multidrug-resistance associated protein; NBD, nucleotide binding domain; NRT, nitrate transporter; PDR, pleiotropic drug-resistance; PUP; purine permease; TMD, transmembrane domain.
References
- Croteau R, Kutchan TM, Lewis NG. Natural products (secondary metabolites). In: Buchanan B, Gruissem W, Jones, R, Editors. Biochemistry & molecular biology of plants. Rockville, MD: American Society of Plant Physiologists; 2000. p. 1250–1318.
- Yazaki K. Natural products and metabolites. In: Christou, P, Klee H, Editors. Handbook of plant biotechnology, Vol. 2, Chichester: Wiley; 2004. p. 811–857.
- Rischer H, Hakkinen ST, Ritala A, et al. Plant cells as pharmaceutical factories. Curr. Pharm. Des. 2013;19:5640–5660.10.2174/1381612811319310017
- Sirikantaramas S, Yamazaki M, Saito K. Mechanisms of resistance to self-produced toxic secondary metabolites in plants. Phytochem. Rev. 2007;7:467–477.
- Verrier PJ, Bird D, Burla B, et al. Plant ABC proteins – a unified nomenclature and updated inventory. Trends Plant Sci. 2008;13:151–159.10.1016/j.tplants.2008.02.001
- Yazaki K, Shitan N, Sugiyama A, et al. Cell and molecular biology of ATP-binding cassette proteins in plants. Int. Rev. Cell Mol. Biol. 2009;276:263–299.10.1016/S1937-6448(09)76006-X
- Shoji T. ATP-binding cassette and multidrug and toxic compound extrusion transporters in plants: a common theme among diverse detoxification mechanisms. Int. Rev. Cell Mol. Biol. 2014;309:303–346.10.1016/B978-0-12-800255-1.00006-5
- Nour-Eldin HH, Andersen TG, Burow M, et al. NRT/PTR transporters are essential for translocation of glucosinolate defence compounds to seeds. Nature. 2012;488:531–534.10.1038/nature11285
- Nour-Eldin HH, Halkier BA. The emerging field of transport engineering of plant specialized metabolites. Curr. Opin. Biotechnol. 2013;24:263–270.10.1016/j.copbio.2012.09.006
- Takanashi K, Shitan N, Yazaki K. The multidrug and toxic compound extrusion (MATE) family in plants. Plant Biotech. 2014;31:417–430.10.5511/plantbiotechnology.14.0904a
- Jelesko JG. An expanding role for purine uptake permease-like transporters in plant secondary metabolism. Front Plant Sci. 2012;3:78.
- Yazaki K, Sugiyama A, Morita M, et al. Secondary transport as an efficient membrane transport mechanism for plant secondary metabolites. Phytochem. Reviews. 2008;7:513–524.10.1007/s11101-007-9079-8
- Yazaki K. Transporters of secondary metabolites. Curr. Opin. Plant Biol. 2005;8:301–307.10.1016/j.pbi.2005.03.011
- Yazaki K. ABC transporters involved in the transport of plant secondary metabolites. FEBS Lett. 2006;580:1183–1191.10.1016/j.febslet.2005.12.009
- Shitan N, Yazaki K. New insights into the transport mechanisms in plant vacuoles. Int. Rev. Cell Mol. Biol. 2013;305:383–433.10.1016/B978-0-12-407695-2.00009-3
- Jørgensen ME, Nour-Eldin HH, Halkier BA. Transport of defense compounds from source to sink: lessons learned from glucosinolates. Trends Plant Sci. 2015;20:508–514.10.1016/j.tplants.2015.04.006
- Zhao J, Dixon RA. The ‘ins’ and ‘outs’ of flavonoid transport. Trends Plant Sci. 2010;15:72–80.10.1016/j.tplants.2009.11.006
- Zhao J. Flavonoid transport mechanisms: how to go, and with whom. Trends Plant Sci. 2015;20:576–585.10.1016/j.tplants.2015.06.007
- De Luca V, Salim V, Thamm A, et al. Making iridoids/secoiridoids and monoterpenoid indole alkaloids: progress on pathway elucidation. Curr. Opin. Plant Biol. 2014;19:35–42.10.1016/j.pbi.2014.03.006
- Verma P, Mathur AK, Srivastava A, et al. Emerging trends in research on spatial and temporal organization of terpenoid indole alkaloid pathway in Catharanthus roseus: a literature update. Protoplasma. 2012;249:255–268.10.1007/s00709-011-0291-4
- Yu F, De Luca V. ATP-binding cassette transporter controls leaf surface secretion of anticancer drug components in Catharanthus roseus. Proc. Natl. Acad. Sci. U S A. 2013;110:15830–15835.10.1073/pnas.1307504110
- Carqueijeiro I, Noronha H, Duarte P, et al. Vacuolar transport of the medicinal alkaloids from Catharanthus roseus is mediated by a proton-driven antiport. Plant Physiol. 2013;162:1486–1496.10.1104/pp.113.220558
- Ikezawa N, Tanaka M, Nagayoshi M, et al. Molecular cloning and characterization of CYP719, a methylenedioxy bridge-forming enzyme that belongs to a novel P450 family, from cultured Coptis japonica cells. J. Biol. Chem. 2003;278:38557–38565.10.1074/jbc.M302470200
- Sakai K, Shitan N, Sato F, et al. Characterization of berberine transport into Coptis japonica cells and the involvement of ABC protein. J. Exp. Bot. 2002;53:1879–1886.10.1093/jxb/erf052
- Shitan N, Tanaka M, Terai K, et al. Human MDR1 and MRP1 recognize berberine as their transport substrate. Biosci. Biotechnol. Biochem. 2007;71:242–245.10.1271/bbb.60441
- Yazaki K, Shitan N, Takamatsu H, et al. A novel Coptis japonica multidrug-resistant protein preferentially expressed in the alkaloid-accumulating rhizome. J. Exp. Bot. 2001;52:877–879.
- Shitan N, Bazin I, Dan K, et al. Involvement of CjMDR1, a plant multidrug-resistance-type ATP-binding cassette protein, in alkaloid transport in Coptis japonica. Proc. Natl. Acad. Sci. U S A. 2003;100:751–756.10.1073/pnas.0134257100
- Shitan N, Dalmas F, Dan K, et al. Characterization of Coptis japonica CjABCB2, an ATP-binding cassette protein involved in alkaloid transport. Phytochemistry. 2013;91:109–116.10.1016/j.phytochem.2012.02.012
- Shitan N, Kiuchi F, Sato F, et al. Establishment of Rhizobium-mediated transformation of Coptis japonica and molecular analyses of transgenic plants. Plant Biotechnol. 2005;22:113–118.10.5511/plantbiotechnology.22.113
- Otani M, Shitan N, Sakai K, et al. Characterization of Vacuolar transport of the endogenous alkaloid berberine in Coptis japonica. Plant Physiol. 2005;138:1939–1946.10.1104/pp.105.064352
- Shoji T, Hashimoto T. Smoking out the masters: transcriptional regulators for nicotine biosynthesis in tobacco. Plant Biotech. 2013;30:217–224.10.5511/plantbiotechnology.13.0221a
- Dewey RE, Xie J. Molecular genetics of alkaloid biosynthesis in Nicotiana tabacum. Phytochemistry. 2013;94:10–27.10.1016/j.phytochem.2013.06.002
- Steppuhn A, Gase K, Krock B, et al. Nicotine’s defensive function in nature. PLoS Biol. 2004;2:E217.10.1371/journal.pbio.0020217
- Morita M, Shitan N, Sawada K, et al. Vacuolar transport of nicotine is mediated by a multidrug and toxic compound extrusion (MATE) transporter in Nicotiana tabacum. Proc. Natl. Acad. Sci. U S A. 2009;106:2447–2452.10.1073/pnas.0812512106
- Shitan N, Morita M, Yazaki K. Identification of a nicotine transporter in leaf vacuoles of Nicotiana tabacum. Plant Signal Behav. 2009;4:530–532.10.4161/psb.4.6.8588
- Shitan N, Minami S, Morita M, et al. Involvement of the leaf-specific multidrug and toxic compound extrusion (MATE) transporter Nt-JAT2 in vacuolar sequestration of nicotine in Nicotiana tabacum. PLoS One. 2014;9:e108789.10.1371/journal.pone.0108789
- Shitan N, Hayashida M, Yazaki K. Translocation and accumulation of nicotine via distinct spatio-temporal regulation of nicotine transporters in Nicotiana tabacum. Plant Signal Behav. 2015;10:e1035852.
- Shoji T, Inai K, Yazaki Y, et al. Multidrug and toxic compound extrusion-type transporters implicated in vacuolar sequestration of nicotine in tobacco roots. Plant Physiol. 2009;149:708–718.
- Hildreth SB, Gehman EA, Yang H, et al. Tobacco nicotine uptake permease (NUP1) affects alkaloid metabolism. Proc. Natl. Acad. Sci. U S A. 2011;108:18179–18184.10.1073/pnas.1108620108
- Kato K, Shitan N, Shoji T, et al. Tobacco NUP1 transports both tobacco alkaloids and vitamin B6. Phytochemistry. 2015;113:33–40.10.1016/j.phytochem.2014.05.011
- Kato K, Shoji T, Hashimoto T. Tobacco nicotine uptake permease regulates the expression of a key transcription factor gene in the nicotine biosynthesis pathway. Plant Physiol. 2014;166:2195–2204.10.1104/pp.114.251645
- Zhao B, Agblevor F, Ritesh. KC, et al. Enhanced production of the alkaloid nicotine in hairy root cultures of Nicotiana tabacum L. Plant Cell, Tissue Organ Culture (PCTOC). 2013;113:121–12910.1007/s11240-012-0256-0
- Kessler D, Gase K, Baldwin IT. Field experiments with transformed plants reveal the sense of floral scents. Science. 2008;321:1200–1202.10.1126/science.1160072
- Kessler D, Bhattacharya S, Diezel C, et al. Unpredictability of nectar nicotine promotes outcrossing by hummingbirds in Nicotiana attenuata. Plant J. 2012;71:529–538.10.1111/tpj.2012.71.issue-4
- Shitan N, Yazaki K. Accumulation and membrane transport of plant alkaloids. Curr. Pharm. Biotechnol. 2007;8:244–252.10.2174/138920107781387429
- Shitan N, Kato K, Shoji T. Alkaloid transporters in plants. Plant Biotech. 2014;31:453–463.
- Falcone Ferreyra ML, Rius SP, Casati P. Flavonoids: biosynthesis, biological functions, and biotechnological applications. Front Plant Sci. 2012;3:222. http://journal.frontiersin.org/article/10.3389/fpls.2012.00222/abstract
- Petrussa E, Braidot E, Zancani M, et al. Plant flavonoids – biosynthesis, transport and involvement in stress responses. Int. J. Mol. Sci. 2013;14:14950–14973.10.3390/ijms140714950
- Marrs KA, Alfenito MR, Lloyd AM, et al. A glutathione S-transferase involved in vacuolar transfer encoded by the maize gene Bronze-2. Nature. 1995;375:397–400.10.1038/375397a0
- Goodman CD, Casati P, Walbot V. A multidrug resistance-associated protein involved in anthocyanin transport in Zea mays. Plant Cell. 2004;16:1812–1826.10.1105/tpc.022574
- Francisco RM, Regalado A, Ageorges A, et al. ABCC1, an ATP binding cassette protein from grape berry, transports anthocyanidin 3-O-glucosides. Plant Cell. 2013;25:1840–1854.10.1105/tpc.112.102152
- Mathews H, Clendennen SK, Caldwell CG, et al. Activation tagging in tomato identifies a transcriptional regulator of anthocyanin biosynthesis, modification, and transport. Plant Cell. 2003;15:1689–1703.10.1105/tpc.012963
- Gomez C, Terrier N, Torregrosa L, et al. Grapevine MATE-type proteins act as vacuolar H+-dependent acylated anthocyanin transporters. Plant Physiol. 2009;150:402–415.10.1104/pp.109.135624
- Gomez C, Conejero G, Torregrosa L, et al. In vivo grapevine anthocyanin transport involves vesicle-mediated trafficking and the contribution of anthoMATE transporters and GST. Plant J. 2011;67:960–970.10.1111/tpj.2011.67.issue-6
- Zhao J, Huhman D, Shadle G, et al. MATE2 mediates vacuolar sequestration of flavonoid glycosides and glycoside malonates in Medicago truncatula. Plant Cell. 2011;23:1536–1555.10.1105/tpc.110.080804
- Thompson EP, Davies JM, Glover BJ. Identifying the transporters of different flavonoids in plants. Plant Signal Behav. 2010;5:860–863.10.4161/psb.5.7.11894
- Thompson EP, Wilkins C, Demidchik V, et al. An Arabidopsis flavonoid transporter is required for anther dehiscence and pollen development. J. Exp. Bot. 2009;61:439–451.
- Kitamura S, Oono Y, Narumi I. Arabidopsis pab1, a mutant with reduced anthocyanins in immature seeds from banyuls, harbors a mutation in the MATE transporter FFT. Plant Mol Biol. 2016;90:7–18.
- Zhao J, Dixon RA. MATE transporters facilitate vacuolar uptake of epicatechin 3'-O-glucoside for proanthocyanidin biosynthesis in Medicago truncatula and arabidopsis. Plant Cell. 2009;21:2323–2340.10.1105/tpc.109.067819
- Debeaujon I, Peeters AJ, Leon-Kloosterziel KM, et al. The TRANSPARENT TESTA12 gene of arabidopsis encodes a multidrug secondary transporter-like protein required for flavonoid sequestration in vacuoles of the seed coat endothelium. Plant Cell. 2001;13:853–871.10.1105/tpc.13.4.853
- Marinova K, Pourcel L, Weder B, et al. The arabidopsis MATE transporter TT12 acts as a vacuolar flavonoid/H+ -antiporter active in proanthocyanidin-accumulating cells of the seed coat. Plant Cell. 2007;19:2023–2038.10.1105/tpc.106.046029
- Pérez-Díaz R, Ryngajllo M, Pérez-Díaz J, et al. VvMATE1 and VvMATE2 encode putative proanthocyanidin transporters expressed during berry development in Vitis vinifera L. Plant Cell Rep. 2014;33:1147–1159.10.1007/s00299-014-1604-9
- Frank S, Keck M, Sagasser M, et al. Two differentially expressed MATE factor genes from apple complement the Arabidopsis transparent testa12 mutant. Plant Biol. (Stuttg). 2011;13:42–50.10.1111/plb.2010.13.issue-1
- Braidot E, Petrussa E, Bertolini A, et al. Evidence for a putative flavonoid translocator similar to mammalian bilitranslocase in grape berries (Vitis vinifera L.) during ripening. Planta. 2008;228:203–213.10.1007/s00425-008-0730-4
- Bertolini A, Peresson C, Petrussa E, et al. Identification and localization of the bilitranslocase homologue in white grape berries (Vitis vinifera L.) during ripening. J. Exp. Bot. 2009;60:3861–3871.10.1093/jxb/erp225
- Poustka F, Irani NG, Feller A, et al. A trafficking pathway for anthocyanins overlaps with the endoplasmic reticulum-to-vacuole protein-sorting route in arabidopsis and contributes to the formation of vacuolar inclusions. Plant Physiol. 2007;145:1323–1335.10.1104/pp.107.105064
- Ichino T, Fuji K, Ueda H, et al. GFS9/TT9 contributes to intracellular membrane trafficking and flavonoid accumulation in Arabidopsis thaliana. Plant J. 2014;80:410–423.10.1111/tpj.2014.80.issue-3
- Chanoca A, Kovinich N, Burkel B, et al. Anthocyanin vacuolar inclusions form by a microautophagy mechanism. Plant Cell. 2015;27:2545–2559.10.1105/tpc.15.00589
- Sun Y, Li H, Huang JR. Arabidopsis TT19 functions as a carrier to transport anthocyanin from the cytosol to tonoplasts. Mol. Plant. 2012;5:387–400.10.1093/mp/ssr110
- Hassan S, Mathesius U. The role of flavonoids in root-rhizosphere signalling: opportunities and challenges for improving plant-microbe interactions. J. Exp. Bot. 2012;63:3429–3444.10.1093/jxb/err430
- Sugiyama A, Yazaki K. Flavonoids in plant rhizospheres: secretion, fate and their effects on biological communication. Plant Biotech. 2014;31:431–443.10.5511/plantbiotechnology.14.0917a
- Sugiyama A, Yamazaki Y, Yamashita K, et al. Developmental and nutritional regulation of isoflavone secretion from soybean roots. Biosci. Biotechnol. Biochem. 2015;80:89–94.
- Sugiyama A, Shitan N, Yazaki K. Involvement of a soybean ATP-binding cassette-type transporter in the secretion of genistein, a signal flavonoid in legume-rhizobium symbiosis. Plant Physiol. 2007;144:2000–2008.10.1104/pp.107.096727
- Banasiak J, Biala W, Staszkow A, et al. A Medicago truncatula ABC transporter belonging to subfamily G modulates the level of isoflavonoids. J. Exp. Bot. 2013;64:1005–1015.10.1093/jxb/ers380
- Fourcroy P, Sisó-Terraza P, Sudre D, et al. Involvement of the ABCG37 transporter in secretion of scopoletin and derivatives by Arabidopsis roots in response to iron deficiency. New Phytol. 2014;201:155–167.10.1111/nph.12471
- Alejandro S, Lee Y, Tohge T, et al. AtABCG29 Is a monolignol transporter involved in lignin biosynthesis. Curr. Biol. 2012;22:1207–1212.10.1016/j.cub.2012.04.064
- Tsuyama T, Kawai R, Shitan N, et al. Proton-dependent coniferin transport, a common major transport event in differentiating xylem tissue of woody plants. Plant Physiol. 2013;162:918–926.10.1104/pp.113.214957
- Halkier BA, Gershenzon J. Biology and biochemistry of glucosinolates. Annu. Rev. Plant, Biol. 2006;57:303–333.10.1146/annurev.arplant.57.032905.105228
- Ellerbrock B, Kim JH, Jander G. Contribution of glucosinolate transport to arabidopsis defense responses. Plant Signal Behav. 2007;2:282–283.10.4161/psb.2.4.4014
- Andersen TG, Nour-Eldin HH, Fuller VL, et al. Integration of biosynthesis and long-distance transport establish organ-specific glucosinolate profiles in vegetative arabidopsis. Plant Cell. 2013;25:3133–3145.10.1105/tpc.113.110890
- Andersen TG, Halkier BA. Upon bolting the GTR1 and GTR2 transporters mediate transport of glucosinolates to the inflorescence rather than roots. Plant Signal Behav. 2014;9:e27740.10.4161/psb.27740
- Madsen SR, Olsen CE, Nour-Eldin HH, et al. Elucidating the role of transport processes in leaf glucosinolate distribution. Plant Physiol. 2014;166:1450–1462.10.1104/pp.114.246249
- Saito H, Oikawa T, Hamamoto S, et al. The jasmonate-responsive GTR1 transporter is required for gibberellin-mediated stamen development in Arabidopsis. Nat. Commun. 2015;6:6095. http://www.nature.com/ncomms/2015/150204/ncomms7095/full/ncomms7095.html 10.1038/ncomms7095
- Ncube B, Van Staden J. Tilting plant metabolism for improved metabolite biosynthesis and enhanced human benefit. Molecules. 2015;20:12698–12731.10.3390/molecules200712698
- Jasinski M, Stukkens Y, Degand H, et al. A plant plasma membrane ATP binding cassette-type transporter is involved in antifungal terpenoid secretion. Plant Cell. 2001;13:1095–1107.10.1105/tpc.13.5.1095
- Stukkens Y, Bultreys A, Grec S, et al. NpPDR1, a pleiotropic drug resistance-type ATP-binding cassette transporter from Nicotiana plumbaginifolia, plays a major role in plant pathogen defense. Plant Physiol. 2005;139:341–352.10.1104/pp.105.062372
- Bultreys A, Trombik T, Drozak A, et al. Nicotiana plumbaginifolia plants silenced for the ATP-binding cassette transporter gene NpPDR1 show increased susceptibility to a group of fungal and oomycete pathogens. Mol. Plant Pathol. 2009;10:651–663.10.1111/mpp.2009.10.issue-5
- van den Brule S, Muller A, Fleming AJ, et al. The ABC transporter SpTUR2 confers resistance to the antifungal diterpene sclareol. Plant J. 2002;30:649–662.10.1046/j.1365-313X.2002.01321.x
- Campbell EJ, Schenk PM, Kazan K, et al. Pathogen-responsive expression of a putative ATP-binding cassette transporter gene conferring resistance to the diterpenoid sclareol is regulated by multiple defense signaling pathways in arabidopsis. Plant Physiol. 2003;133:1272–1284.10.1104/pp.103.024182
- Kang J, Hwang JU, Lee M, et al. PDR-type ABC transporter mediates cellular uptake of the phytohormone abscisic acid. Proc. Natl. Acad. Sci. U S A. 2010;107:2355–2360.10.1073/pnas.0909222107
- Lee M, Lee K, Lee J, et al. AtPDR12 contributes to lead resistance in arabidopsis. Plant Physiol. 2005;138:827–836.10.1104/pp.104.058107
- Sasabe M, Toyoda K, Shiraishi T, et al. cDNA cloning and characterization of tobacco ABC transporter: NtPDR1 is a novel elicitor-responsive gene. FEBS Lett. 2002;518:164–168.10.1016/S0014-5793(02)02697-2
- Schenke D, Sasabe M, Toyoda K, et al. Genomic structure of the NtPDR1 gene, harboring the two miniature inverted-repeat transposable elements, NtToya1 and NtStowaway101. Genes Genet. Syst. 2003;78:409–418.10.1266/ggs.78.409
- Crouzet J, Roland J, Peeters E, et al. NtPDR1, a plasma membrane ABC transporter from Nicotiana tabacum, is involved in diterpene transport. Plant Mol. Biol. 2013;82:181–192.10.1007/s11103-013-0053-0
- Hurlock AK, Roston RL, Wang K, et al. Lipid trafficking in plant cells. Traffic. 2014;15:915–932.10.1111/tra.2014.15.issue-9
- Bernard A, Joubès J. Arabidopsis cuticular waxes: advances in synthesis, export and regulation. Prog. Lipid Res. 2013;52:110–129.10.1016/j.plipres.2012.10.002
- Li N, Xu C, Li-Beisson Y, et al. Fatty acid and lipid transport in plant cells. Trends Plant Sci. 2016;21:145–158.
- Pighin JA, Zheng H, Balakshin LJ, et al. Plant cuticular lipid export requires an ABC transporter. Science. 2004;306:702–704.10.1126/science.1102331
- McFarlane HE, Shin JJ, Bird DA, et al. Arabidopsis ABCG transporters, which are required for export of diverse cuticular lipids, dimerize in different combinations. Plant Cell. 2010;22:3066–3075.10.1105/tpc.110.077974
- Bird D, Beisson F, Brigham A, et al. Characterization of Arabidopsis ABCG11/WBC11, an ATP binding cassette (ABC) transporter that is required for cuticular lipid secretion. Plant J. 2007;52:485–498.10.1111/j.1365-313X.2007.03252.x
- Luo B, Xue XY, Hu WL, et al. An ABC transporter gene of Arabidopsis thaliana, AtWBC11, is involved in cuticle development and prevention of organ fusion. Plant Cell Physiol. 2007;48:1790–1802.10.1093/pcp/pcm152
- Panikashvili D, Savaldi-Goldstein S, Mandel T, et al. The Arabidopsis DESPERADO/AtWBC11 transporter is required for cutin and wax secretion. Plant Physiol. 2007;145:1345–1360.10.1104/pp.107.105676
- Ukitsu H, Kuromori T, Toyooka K, et al. Cytological and biochemical analysis of COF1, an Arabidopsis mutant of an ABC transporter gene. Plant Cell Physiol. 2007;48:1524–1533.10.1093/pcp/pcm139
- Buda GJ, Barnes WJ, Fich EA, et al. An ATP binding cassette transporter is required for cuticular wax deposition and desiccation tolerance in the moss Physcomitrella patens. Plant Cell. 2013;25:4000–4013.10.1105/tpc.113.117648
- Mizuno H, Kawahigashi H, Ogata J, et al. Genomic inversion caused by gamma irradiation contributes to downregulation of a WBC11 homolog in bloomless sorghum. Theor. Appl. Genet. 2013;126:1513–1520.10.1007/s00122-013-2069-x
- Chen G, Komatsuda T, Ma JF, et al. An ATP-binding cassette subfamily G full transporter is essential for the retention of leaf water in both wild barley and rice. Proc. Natl. Acad. Sci. U S A. 2011;108:12354–12359.10.1073/pnas.1108444108
- Chen G, Komatsuda T, Ma JF, et al. A functional cutin matrix is required for plant protection against water loss. Plant Signal Behav. 2011;6:1297–1299.10.4161/psb.6.9.17507
- Ma X, Sela H, Jiao G, et al. Population-genetic analysis of HvABCG31 promoter sequence in wild barley (Hordeum vulgare ssp. spontaneum). BMC Evol. Biol. 2012;12:188. http://bmcevolbiol.biomedcentral.com/articles/10.1186/1471-2148-12-18810.1186/1471-2148-12-188
- Yang Z, Zhang T, Lang T, et al. Transcriptome comparative profiling of barley eibi1 mutant reveals pleiotropic effects of HvABCG31 gene on cuticle biogenesis and stress responsive pathways. Int. J. Mol. Sci. 2013;14:20478–20491.10.3390/ijms141020478
- Li L, Li D, Liu S, et al. The maize glossy13 gene, cloned via BSR-Seq and Seq-walking encodes a putative ABC transporter required for the normal accumulation of epicuticular waxes. PLoS One. 2013;8:e82333.10.1371/journal.pone.0082333
- Fabre G, Garroum I, Mazurek S, et al. The ABCG transporter PEC1/ABCG32 is required for the formation of the developing leaf cuticle in Arabidopsis. New Phytol. 2016;209:192–201.10.1111/nph.13608
- Bessire M, Borel S, Fabre G, et al. A member of the PLEIOTROPIC DRUG RESISTANCE family of ATP binding cassette transporters is required for the formation of a functional cuticle in Arabidopsis. Plant Cell. 2011;23:1958–1970.10.1105/tpc.111.083121
- Panikashvili D, Shi JX, Schreiber L, et al. The Arabidopsis ABCG13 transporter is required for flower cuticle secretion and patterning of the petal epidermis. New Phytol. 2011;190:113–124.10.1111/nph.2011.190.issue-1
- Quilichini TD, Grienenberger E, Douglas CJ. The biosynthesis, composition and assembly of the outer pollen wall: A tough case to crack. Phytochemistry. 2015;113:170–182.10.1016/j.phytochem.2014.05.002
- Quilichini TD, Friedmann MC, Samuels AL, et al. ATP-binding cassette transporter G26 is required for male fertility and pollen exine formation in Arabidopsis. Plant Physiol. 2010;154:678–690.10.1104/pp.110.161968
- Xu J, Yang C, Yuan Z, et al. The ABORTED MICROSPORES regulatory network is required for postmeiotic male reproductive development in Arabidopsis thaliana. Plant Cell. 2010;22:91–107.10.1105/tpc.109.071803
- Choi H, Jin J-Y, Choi S, et al. An ABCG/WBC-type ABC transporter is essential for transport of sporopollenin precursors for exine formation in developing pollen. Plant J. 2011;65:181–193.10.1111/tpj.2011.65.issue-2
- Dou XY, Yang KZ, Zhang Y, et al. WBC27, an adenosine tri-phosphate-binding cassette protein, controls pollen wall formation and patterning in arabidopsis. J. Integr. Plant Biol. 2011;53:74–88.10.1111/jipb.2011.53.issue-1
- Kuromori T, Ito T, Sugimoto E, et al. Arabidopsis mutant of AtABCG26, an ABC transporter gene, is defective in pollen maturation. J. Plant Physiol. 2011;168:2001–2005.10.1016/j.jplph.2011.05.014
- Quilichini TD, Samuels AL, Douglas CJ. ABCG26-mediated polyketide trafficking and hydroxycinnamoyl spermidines contribute to pollen wall exine formation in Arabidopsis. Plant Cell. 2014;26:4483–4498.10.1105/tpc.114.130484
- Zhu L, Shi J, Zhao G, et al. Post-meiotic deficient anther1 (PDA1) encodes an ABC transporter required for the development of anther cuticle and pollen exine in rice. J. Plant Biol. 2013;56:59–68.10.1007/s12374-013-0902-z
- Niu BX, He FR, He M, et al. The ATP-binding Cassette Transporter OsABCG15 is required for anther development and pollen fertility in rice. J. Integr. Plant Biol. 2013;55:710–720.10.1111/jipb.v55.8
- Qin P, Tu B, Wang Y, et al. ABCG15 encodes an ABC transporter protein, and is essential for post-meiotic anther and pollen exine development in rice. Plant Cell Physiol. 2013;54:138–154.10.1093/pcp/pcs162
- Wu L, Guan Y, Wu Z, et al. OsABCG15 encodes a membrane protein that plays an important role in anther cuticle and pollen exine formation in rice. Plant Cell Rep. 2014;33:1881–1899.10.1007/s00299-014-1666-8
- Zhao G, Shi J, Liang W, et al. Two ATP Binding Cassette G (ABCG) transporters, OsABCG26 and OsABCG15, collaboratively regulate rice male reproduction. Plant Physiol. 2015;169:2064–2079.
- Yadav V, Molina I, Ranathunge K, et al. ABCG transporters are required for suberin and pollen wall extracellular barriers in Arabidopsis. Plant Cell. 2014;26:3569–3588.10.1105/tpc.114.129049
- Choi H, Ohyama K, Kim YY, et al. The role of Arabidopsis ABCG9 and ABCG31 ATP binding cassette transporters in pollen fitness and the deposition of steryl glycosides on the pollen coat. Plant Cell. 2014;26:310–324.10.1105/tpc.113.118935
- Le Hir R, Sorin C, Chakraborti D, et al. ABCG9, ABCG11 and ABCG14 ABC transporters are required for vascular development in Arabidopsis. Plant J. 2013;76:811–824.10.1111/tpj.12334
- Franke R, Schreiber L. Suberin–a biopolyester forming apoplastic plant interfaces. Curr. Opin. Plant Biol. 2007;10:252–259.10.1016/j.pbi.2007.04.004
- Shiono K, Ando M, Nishiuchi S, et al. RCN1/OsABCG5, an ATP-binding cassette (ABC) transporter, is required for hypodermal suberization of roots in rice (Oryza sativa). Plant J. 2014;80:40–51.10.1111/tpj.2014.80.issue-1
- Landgraf R, Smolka U, Altmann S, et al. The ABC transporter ABCG1 is required for suberin formation in potato tuber periderm. Plant Cell. 2014;26:3403–3415.10.1105/tpc.114.124776
- Panikashvili D, Shi JX, Bocobza S, et al. The Arabidopsis DSO/ABCG11 transporter affects cutin metabolism in reproductive organs and suberin in roots. Mol. Plant. 2010;3:563–575.10.1093/mp/ssp103
- Sirikantaramas S, Yamazaki M, Saito K. How plants avoid the toxicity of self-produced defense bioactive compounds. Nat. Prod. Discourse, Diversity, Des. 2014;69–82.
- Sirikantaramas S, Taura F, Morimoto S, et al. Recent advances in Cannabis sativa research: biosynthetic studies and its potential in biotechnology. Curr. Pharm. Biotechnol. 2007;8:237–243.10.2174/138920107781387456
- Matsuba Y, Sasaki N, Tera M, et al. A novel glucosylation reaction on anthocyanins catalyzed by acyl-glucose-dependent glucosyltransferase in the petals of carnation and delphinium. Plant Cell. 2010;22:3374–3389.10.1105/tpc.110.077487
- Sirikantaramas S, Yamazaki M, Saito K. Mutations in topoisomerase I as a self-resistance mechanism coevolved with the production of the anticancer alkaloid camptothecin in plants. Proc. Natl. Acad. Sci. U S A. 2008;105:6782–6786.10.1073/pnas.0801038105
- Takanashi K, Shitan N, Sugiyama A, et al. Galactinol synthase gene of Coptis japonica is involved in berberine tolerance. Biosci. Biotechnol. Biochem. 2008;72:398–405.10.1271/bbb.70495
- Shitan N, Kamimoto Y, Minami S, et al. A tolerance gene for prenylated flavonoid encodes a 26S proteasome regulatory subunit in Sophora flavescens. Biosci. Biotechnol. Biochem. 2011;75:982–984.10.1271/bbb.100665
- Schroeder JI, Delhaize E, Frommer WB, et al. Using membrane transporters to improve crops for sustainable food production. Nature. 2013;497:60–66.10.1038/nature11909
- Ro DK, Ouellet M, Paradise EM, et al. Induction of multiple pleiotropic drug resistance genes in yeast engineered to produce an increased level of anti-malarial drug precursor, artemisinic acid. BMC Biotechnol. 2008;8:83. http://bmcbiotechnol.biomedcentral.com/articles/10.1186/1472-6750-8-8310.1186/1472-6750-8-83