Abstract
The polyhistidine peptides (PHPs) have been previously reported as novel cell-penetrating peptides and are efficiently internalized into mammal cells; however, penetration of PHPs into other cell types is unknown. In this study, the cellular uptake of PHPs in plant and yeast cells was found to be dependent on the number of histidines, and short PHPs (H6–H10 peptides) showed effective internalization. The H8 peptide showed the highest cell-penetrating capacity and localized to vacuoles in plant and yeast cells. Low-temperature conditions inhibited significantly the cellular uptake of short PHPs by both cells. However, net charge neutralization of PHPs also completely inhibited cellular uptake by plant cells, but not by yeast cells. These results indicate that short PHPs penetrate effectively into plant and yeast cells by similar mechanism with the exception of net charge dependency. The findings show the short PHPs are promising candidates for new delivery tools into plant and yeast cells.
Graphical abstract
Short polyhistidine peptides (H6–H10 peptides) were effectively internalized by plant and yeast cells.
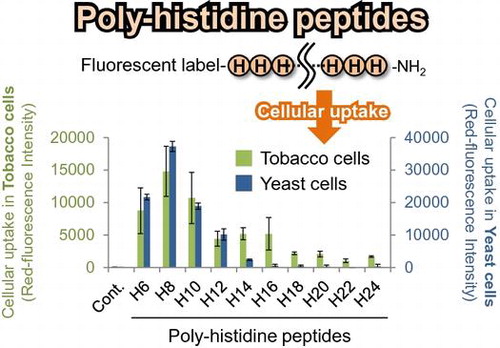
Molecular delivery of compounds to plant cells facilitates improvements in breeds and temporary transformation of plants. For example, it has been reported that functional molecules can regulate a wide range of traits such as form, flowering, smell, taste, dye, and environmental resistance.Citation1–5) In addition, yeasts have been also used for production of useful substances such as biofuels.Citation6) Although discussion to the benefits and drawbacks of using genetically modified crops and organisms continues, it is extremely important in industrial development to give useful traits that are not possible via traditional genetic hybridizations of plants and yeasts. To produce these useful crops and organisms, the development of techniques that introduce functional molecules and genes efficiently into plant and yeast cells are required.
Cell-penetrating peptides (CPPs) are one group of molecular delivery tools.Citation7–9) CPPs are able to introduce hydrophilic molecules with low permeability into cells. Currently, various CPPs have been reported. For example, HIV-Tat (48–60) (TAT-peptide: GRKKRRQRRRPPQ-NH2),Citation10) penetrating (RQIKIWFQNRRMKWKK-NH2),Citation11) and octa-arginine peptide (R8 peptide: RRRRRRRR-NH2)Citation12) are well-known CPPs. Because these CPPs contain cationic basic amino acid, they interact with anionic carbohydrate chains on the surface of membranes leading to internalization of the CPPs effectively into the cytoplasm.Citation13,14) In recent years, CPPs have been studied and applied in various fields.
In a previous study, we found polyhistidine peptides (PHPs) as novel CPPs, which are efficiently internalized in mammalian cells. We demonstrated that PHPs show higher penetrating capacity than already known CPPs.Citation15) However, the cell-penetrating capacity of these PHPs into plant and yeast cells remains unknown.
In this study, we investigated the cellular uptake of PHPs by plant and yeast cells. Several peptides with different numbers of histidine residues were synthesized, and their cellular uptake efficiency was determined using Tobacco tabacum suspension-cultured cells (BY-2) and Saccharomyces cerevisiae (NMY51). Furthermore, we examined the influence of cell wall, charge, and temperature to elucidate the cell-penetrating mechanism of PHPs by plant and yeast cells.
Materials and methods
Peptide synthesis and purification
The PHPs were synthesized by Biologica (Shanghai, China) with tetramethyl-rhodamine (TAMRA) labeling at the N-terminus and an amide group at the C-terminus.
All peptides were purified to homogeneity by Waters purification systems (Waters, Milford, USA) using a reversed-phase COSMOSIL 5C18-MS-II column (10 × 250 mm) (Nacalai Tesque, Kyoto, Japan). The column was run for 50 min at 1 mL/min with a linear gradient from 10 to 60% (v/v) acetonitrile in water containing 0.1% (v/v) trifluoroacetic acid. The molecular masses were confirmed by matrix-assisted laser desorption/ionization time-of-flight mass spectrometry (Auto FlexII Bruker Daltonics, Billerica, USA).
Cell culture
Nicotiana tabacum (tobacco) suspension-cultured cells (BY-2; resource number rpc00001) were obtained from the RIKEN BioResource Center (Ibaraki, Japan) and used as plant cells. The BY-2 cells were cultured in Linsmaier and Skoog (LS) medium (pH 5.8) supplemented with 3% sucrose at 27 °C on a rotary shaker (200 rpm) and subcultured every week.
S. cerevisiae (NMY51) were kindly gifted from Dr Yabuta and used as yeast cells. The yeast cells were cultured in YPD liquid medium (1% yeast extract, 2% peptone, 2% glucose) at 30 °C. Prior to liquid culturing, the frozen glycerol stock was streak cultured onto YPD plates and the plates incubated for 2–3 days at 30 °C, and then a single colony was suspended in YPD liquid medium.
Protoplast preparation
The tobacco BY-2 cells in LS medium were incubated with equal amounts of digestion medium (3% cellulase, 0.2% macerozym, 0.1% pectolyase, 8 mM CaCl2, 25 mM MES-KOH, pH 5.6, 450 mM sucrose) for 3 h at 37 °C under slow shaking. After incubation, the BY-2 cells were washed three times with W5 solution (154 mM NaCl, 125 mM CaCl2, 5 mM KCl, 5 mM MES-KOH, pH 5.7) and collected by centrifugation for 3 min at 100 × g. The collected BY-2 cells were suspended in the W5 solution until use.
The yeast MMY51 cells in YPD medium were washed with distilled water by centrifugation for 3 min at 100 × g. The collected cells were suspended in pre-incubation medium [50 mM ethylenediaminetetraacetic acid (EDTA) pH 9.0, 35 mM β-mercaptoethanol] and shaken slowly (40 rpm) for 20 min at 37 °C. After pre-incubation, the yeast cells were harvested and washed in the following buffer: washing solution I (1.2 M sorbitol, 50 mM EDTA, pH 7.5), washing solution II (1.2 M sorbitol, 50 mM Tris, pH 7.5), and enzyme buffer (1.2 M sorbitol, 50 mM Tris, 0.1 mM potassium acetate, 0.5 mM magnesium acetate) by centrifugation for 3 min at 100 × g. After washing, the yeast cells were suspended in enzyme solution (1.2 M sorbitol, 50 mM Tris, 0.1 mM potassium acetate, 0.5 mM magnesium acetate, 60 U/mL zymolyase) and shaken slowly (40 rpm) for 90 min at 37 °C. After enzymatic treatment, the yeast cells were collected by centrifugation for 3 min at 100 × g and suspended in the enzyme buffer until use.
Confocal laser scanning microscopy (CLSM)
The tobacco BY-2 cells (2.0 × 104 cells/mL) in a final volume of 500-μL LS medium were treated with 1 μM of TAMRA-labeled PHPs. Incubation was performed for 12 h at 27 °C on a rotary shaker (200 rpm). After incubation, the BY-2 cells were washed three times with LS medium by centrifugation for 5 min at 500 × g and were suspended in LS medium until observation.
The yeast cells (OD600 = 0.5) in a final volume of 50-μL YPD medium were treated with 10 μM of TAMRA-labeled PHPs. Incubation was performed for 1 h at 30 °C on a rotary shaker (300 rpm). After incubation, the yeast cells were washed three times with phosphate-buffered saline (PBS) by centrifugation for 5 min at 500 × g and suspended in PBS until observation.
Intracellular uptake of the TAMRA-labeled PHPs in the BY-2 and yeast cells was observed under CLSM FluoView (Olympus, Tokyo, Japan). Fluorescence images were obtained using the conditions for rhodamine detection (excitation wavelength, 555 nm; emission wavelength, 580 nm). Subsequently, the cell walls of the BY-2 and yeast cells were removed by the protoplast preparation method described above. The amounts of TAMRA-labeled PHPs retained in the cells were quantified by measuring the fluorescence intensity (pixels) in the cells.
Intracellular distribution of TAMRA-labeled PHPs in the BY-2 and yeast cells was determined by checking co-localization with organelle markers. Hoechst 33342 (Dojindo, Kumamoto, Japan), Fluorescent Brightener 28 (Sigma-Aldrich, At. Louis, MO), and BCECF-AM (Sigma-Aldrich, At. Louis, MO) were used as organelle markers for nucleus, cell wall, and vacuole, respectively. The BY-2 and yeast cells were treated with TAMRA-labeled PHPs and washed under the same conditions described above. After washing, the both cells treated with 10 μg/mL Hoechst 33342 (30 min), 1 μg/mL Fluorescent Brightener 28 (10 min), or 30 μg/mL BCECF-AM (30 min), and washed again. The fluorescence images were acquired using the FluoView, and intracellular distribution of the TAMRA-labeled PHPs was determined.
Results
Cellular uptake of PHPs by CLSM
We designed and synthesized the PHPs with TAMRA labeling to evaluate cellular uptake in BY-2 plant cells and yeast cells (Table ). While long PHPs (longer than 16 histidine residues) showed high cellular uptake by mammalian cells in our previous study,Citation15) short PHPs (H6–H10) seemed to be internalized in both plant cells (Fig. (A)) and yeast cells (Fig. (A)). On the other hand, long PHPs were trapped in the cell walls of plant cells. To confirm cellular uptake of short PHPs in both cells, cell walls were removed by enzymatic degradation and intracellular TAMRA-labeled PHPs in protoplasts were observed and quantified under CLSM. Fluorescent images showed short PHPs localized to not cell walls but subcellular regions in plant cells (Fig. (B)) and yeast cells (Fig. (B)). Fluorescent quantitative analysis in protoplasts also showed short PHPs were internalized in both plant cells (Fig. (C)) and yeast cells (Fig. (C)).
Table 1. Sequence and net charge of the PHPs used this study.
Fig. 1. Cellular uptake of PHPs into BY-2 plant cells.
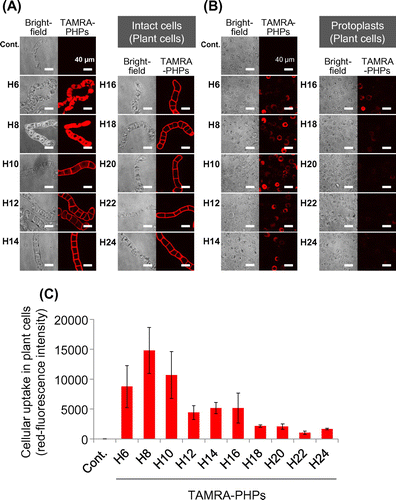
Intracellular distribution of PHPs
Intracellular distribution of the PHPs after cell penetration was determined using three organelle markers. Organelle markers used in this experiment were Hoechst 33342, Fluorescent Brightener 28, and BCECF-AM to stain nucleus, cell wall, and vacuole respectively. Co-localization between each organelle marker and PHPs in the plant and yeast cells was observed under CLSM. The TAMRA-H8 peptide and TAMRA-H24 peptide were used as a short and long PHP. As a result, red fluorescence derived from the TAMRA-H8 peptide was observed in the same area of green fluorescence from BCECF-AM in both cells. Quantitative analysis for distribution of fluorescence intensity also showed co-localization between the TAMRA-H8 peptide and vacuole (Figs. (A) and (A)). On the other hand, the TAMRA-H24 peptide showed no cellular uptake in both cells and co-localization with the cell walls in the plant cells.
The influence of temperature on cellular uptake of PHPs
Temperature is a critical factor for cellular uptake of CPPs via endocytosis pathways. Thus, the influence of temperature on the cellular uptake of PHPs was investigated. The cellular uptake of PHPs under physiological (27 °C for plant cells; 30 °C for yeast cells) and low-temperature conditions (4 °C) was compared. We found that cellular uptake of PHPs by both plant and yeast cells was inhibited significantly under the low-temperature condition (Figs. (B) and (B)). For plant cells, long PHPs were observed to be trapped in the cell walls, as observed for the results presented in Fig. (A).
The influence of positive charge on cellular uptake of PHPs
Next, we examined the influence of positive charge on PHPs’ cellular uptake by plant and yeast cells. The cell walls are negatively charged, thus, it seemed that the positively charged histidine residues may interact with the negatively charged cell walls and influence the cellular uptake of PHP. Therefore, we compared the cellular uptake of PHPs in plant and yeast cells under conditions where histidine residues are positively charged (pH 5.8) and neutral (pH 7.4). As a result, at pH 7.4, the cellular uptake of all PHPs in the plant cells was inhibited completely (Fig. (C)). Adsorption of long PHPs on the plant cell wall was also prevented. In contrast, cellular uptake of short PHPs by the yeast cells was not inhibited under pH 7.4 conditions (Fig. (C)).
Discussion
In this study, we investigated the cellular uptake of PHPs by BY-2 and yeast cells. Interestingly, short PHPs (H6–H10) showed high cell-penetrating capacity into tobacco BY-2 and yeast cells (Figs. and ). However, longer PHPs showed no cellular uptake by both cell types. The correlation between the length of PHPs and cell-penetrating capacity against plant/yeast cells differed to our previous report describing the uptake of PHPs by mammalian cells. Our previous study reported that long PHPs (>14 histidine residues) showed high cellular uptake by mammalian cells, whereas short PHPs (<12 histidine residues) showed no cell-penetrating capacity.Citation15) Although the reason for this difference is unclear, our results indicate the selectivity of short PHPs is common feature in the cellular uptake of PHPs by both plant and yeast cells.
Fig. 2. Cellular uptake of PHPs into NMY51 yeast cells.
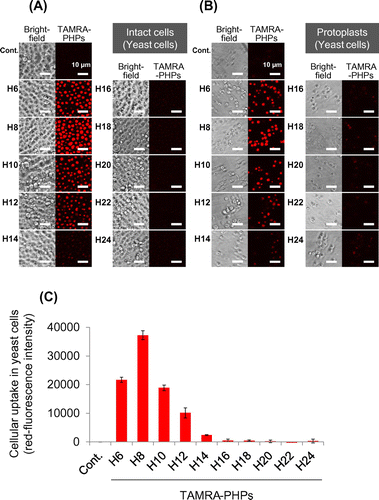
Fig. 3. Distribution and cellular uptake route of PHPs in the BY-2 plant cells.
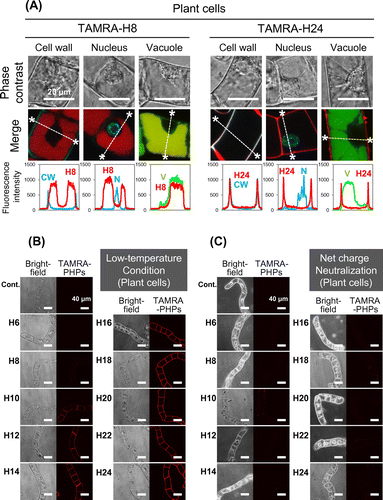
Fig. 4. Distribution and cellular uptake route of PHPs in the NMY51 yeast cells.
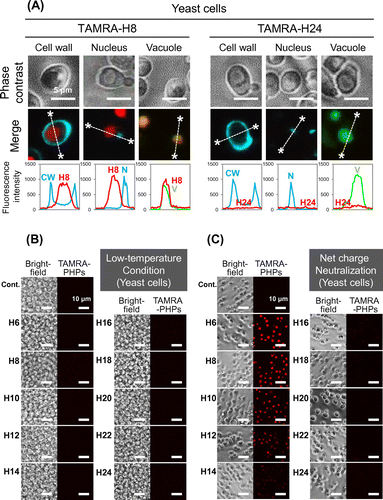
Intracellular distribution of short PHP also showed similar feature in plant and yeast cells. The H8 peptide, used as a typical short PHP, localized to vacuole after cellular uptake (Figs. (A) and (A)). This result is reasonable because extracellular molecules are known to be transported to the vacuole when they are imported via endocytosis in plant and yeast cells.Citation16,17) It is speculated that the short PHP was also transported into vacuole by similar pathway described above after cell penetration.
To elucidate the cellular uptake pathway of the PHPs, the influence of temperature against the PHPs cellular uptake in the plant and yeast cells were examined. As a result, the cellular uptake of short PHPs in both the plant and yeast cells was inhibited significantly under a low-temperature condition (4 °C) (Figs. (B) and (B)). This result indicates that the short PHPs are internalized into the plant/yeast cells via a temperature-dependent pathway. Currently, various CPPs are reported to be internalized into cells via endocytosis and their cellular uptakes are inhibited under low-temperature conditions.Citation18,19) From these reports, cellular uptake of the short PHPs is also considered to be via the endocytosis pathway. The PHPs have also been reported to penetrate mammalian cell membranes via endocytosis in a temperature-dependent manner.Citation15) Therefore, temperature-dependent endocytosis is a common uptake route for the cellular uptake of PHPs by various types of cells.
However, long PHPs (H12–H24) were trapped in only cell wall of BY-2 cells (Figs. (A), (A) and (B)). The adsorption in the cell walls of plant cells has also been reported for other cationic CPPs.Citation20,21) Because the cell wall of plant cells are negatively charged, the interactions between cationic CPPs and the cell wall of plants cells are attributed to electrostatic interactions.Citation16) Therefore, we hypothesize that the adsorption of long PHPs in cell walls was also owing to electrostatic interactions. To confirm this hypothesis, the cellular uptake of PHPs was determined at pH 7.4. At this pH, histidine (intrinsic pKa = 6.0) is neutral, whereas a lower pH value of this amino acid is protonated and thus positively charged (Table ). As expected, long PHPs showed no adsorption into the cell walls under conditions where the histidine was neutral (pH 7.4) (Fig. (C)). These results indicate that the adsorption of long PHPs in the cell walls was caused by electrostatic interactions as well as other cationic CPPs. In other words, these results show short PHPs are effective CPPs for plant cells without the adsorption in cell walls (Fig. (A)). However, cellular uptake of short PHPs by plant cells was also inhibited by net charge neutralization of histidine at pH 7.4 (Fig. (C)). This result suggests that positive charge of histidine is essential for the cellular uptake of short PHPs into plant cells. Short PHPs (H6–H10) have a lower overall net positive charge (3.68–6.13) when compared with those of longer PHPs under acidic pH conditions (pH 5.8) (Table ), and we speculate this lower net positive charge may facilitate weak electrostatic interactions with cell walls that lead to subsequent internalization.
On the other hand, long PHPs showed no adsorption in the cell walls in yeast cells (Figs. (A), (A) and (B)). Short PHPs also showed cellular uptake by yeast cells under pH 7.4 conditions (Fig. (C)), even though a large difference in the net charge between pH 5.8 and 7.4 conditions existed for the PHPs (Table ). These results indicate that the cellular uptake of PHPs in yeast cells occurs in a positive charge-independent manner, while for plant cells cellular uptake of PHPs occurs via a positive charge-dependent process. We postulate that this difference in positive charge dependency is attributed to different components present in the cell walls of plant and yeast cells. Plant cell walls are composed mainly of cellulose, hemicellulose, and pectin.Citation22,23) These cell walls contain free carboxylate groups of galacturonic acid moieties in the pectin layer. Thus, it is likely that the positively charged histidine residues strongly interact with the negatively charged plant cell walls under the experimental conditions used (i.e. pH 5.8). However, yeast cell walls are composed mainly of mannan, glucan, and chitin.Citation24,25) Mannan is present in the outermost layer of the yeast cell wall. While a small fraction of the neutral carbohydrate chains undergoes modification by the addition of acidic carbohydrate chains to the mannose phosphate, the main components of mannan are neutral carbohydrate chains.Citation26) Although yeast cell walls also have a negative charge, the negativity may be noticeably weaker than that observed for plant cell walls. This difference likely explains why the cellular uptake of short PHPs in yeast cells is positive charge independent in comparison with that observed for the uptake of PHPs by plant cells. No adsorption of long PHPs in the yeast cell walls is also speculated to result from the negligible influence of the positive charge on PHPs.
In conclusion, we demonstrated the cellular uptake of PHPs in plant and yeast cells. Our results indicate some similarities in the cellular uptake of PHPs by both plant and yeast cells, for example, (1) selectivity of short PHPs; (2) intracellular localization to vacuole; (3) temperature-dependent endocytosis. However, positive charge plays a crucial role in only plant cellular uptake of PHPs. These observations suggest that PHPs penetrate into plant and yeast cells via similar uptake routes. Although further studies are required, the findings support the potential capacity of PHPs as molecular delivery tools for plant and yeast cells.
Author contributions
Sayaka Kimura, Tsuyoshi Kawano, and Takashi Iwasaki conceived and designed the experiments. Sayaka Kimura performed the experiments and analyzed the data. Sayaka Kimura and Takashi Iwasaki wrote the paper with assistance from Tsuyoshi Kawano.
Disclosure statement
No potential conflict of interest was reported by the authors.
Funding
This work was supported in part by a grant from the KATO Memorial Bioscience Foundation; the Takahashi Industrial and Economic Research Foundation; the Japan Society for the Promotion of Science KAKENHI [grant number 15625058].
Acknowledgments
We thank Dr Yukinori Yabuta, Graduate School of Agricultural Sciences, Tottori University, for kindly providing the yeast cells (NMY51).
Notes
Abbreviations: CPP, cell-penetrating peptide; CLSM, confocal laser scanning microscopy; EDTA, ethylenediaminetetraacetic acid; PHPs, polyhistidine peptides; TAMRA, tetramethyl rhodamine.
References
- Tsuji H, Tachibana C, Tamaki S, et al. Hd3a promotes lateral branching in rice. Plant J. 2015;82:256–266.10.1111/tpj.2015.82.issue-2
- Löfke C, Dünser K, Scheuring D, et al. Auxin regulates SNARE-dependent vacuolar morphology restricting cell size. Elife. 2015;2015:e05868.
- Guterman I, Masci T, Chen X, et al. Generation of phenylpropanoid pathway-derived volatiles in transgenic plants: rose alcohol acetyltransferase produces phenylethyl acetate and benzyl acetate in petunia flowers. Plant Mol. Biol. 2006;60:555–563.10.1007/s11103-005-4924-x
- Tanaka Y, Brugliera F. Flower colour and cytochromes P450. Philos. Trans. R. Soc. Lond. B. Biol. Sci. 2013;368:20120432.10.1098/rstb.2012.0432
- Zou HW, Tian XH, Ma GH, et al. Isolation and functional analysis of ZmLTP3, a homologue to arabidopsis LTP3. Int. J. Mol. Sci. 2013;14:5025–5035.10.3390/ijms14035025
- MXHe, BWu, HQin, et al. Zymomonas mobilis: a novel platform for future biorefineries. Biotechnol. Biofuels. 2014;7. doi: 10.1186/1754-6834-7-101
- Bechara C, Sagan S. Cell-penetrating peptides: 20 years later, where do we stand? FEBS Lett. 2013;587:1693–1702.10.1016/j.febslet.2013.04.031
- Koren E, Torchilin VP. Cell-penetrating peptides: breaking through to the other side. Trends Mol. Med. 2012;18:385–393.10.1016/j.molmed.2012.04.012
- Heitz F, Morris MC, Divita G. Twenty years of cell-penetrating peptides: from molecular mechanisms to therapeutics. Br. J. Pharmacol. 2009;157:195–206.10.1111/j.1476-5381.2009.00057.x
- Vives E, Brodin P, Lebleu B. A truncated HIV-1 tat protein basic domain rapidly translocates through the plasma membrane and accumulates in the cell nucleus. J. Biol. Chem. 1997;272:16010–16017.10.1074/jbc.272.25.16010
- Derossi D, Joliot AH, Chassaing G, et al. The third helix of the Antennapedia homeodomain translocates through biological membranes. J. Biol. Chem. 1994;269:10444–10450.
- Futaki S, Suzuki T, Ohashi W, et al. Arginine-rich Peptides. An abundant source of membrane-permeable peptides having potential as carriers for intracellular protein delivery. J. Biol. Chem. 2001;276:5836–5840.10.1074/jbc.M007540200
- Poon GM, Gariépy J. Cell-surface proteoglycans as molecular portals for cationic peptide and polymer entry into cells. Biochem. Soc. Trans. 2007;35:788–793.10.1042/BST0350788
- Futaki S, Nakase I, Tadokoro A, et al. Arginine-rich peptides and their internalization mechanisms. Biochem. Soc. Trans. 2007;35:784–787.10.1042/BST0350784
- Iwasaki T, Tokuda Y, Kotake A, et al. Cellular uptake and in vivo distribution of polyhistidine peptides. J. Control. Release. 2015;210:115–124.10.1016/j.jconrel.2015.05.268
- Otegui MS, Spitzer C. Endosomal functions in plants. Traffic. 2008;9:1589–1598.10.1111/tra.2008.9.issue-10
- Feyder S, De Craene JO, Bär S, et al. Membrane trafficking in the yeast Saccharomyces cerevisiae Model. Int. J. Mol. Sci. 2015;16:1509–1525.10.3390/ijms16011509
- Richard JP, Melikov K, Vives E, et al. Cell-penetrating peptides A reevaluation of the mechanism of cellular uptake. J. Biol. Chem. 2003;278:585–590.
- Tréhin R, Merkle HP. Chances and pitfalls of cell penetrating peptides for cellular drug delivery. Eur. J. Pharm. Biopharm. 2004;58:209–223.10.1016/j.ejpb.2004.02.018
- Mizuno T, Miyashita M, Miyagawa H. Cellular internalization of arginine-rich peptides into tobacco suspension cells: a structure–activity relationship study. J. Pept. Sci. 2009;15:259–263.10.1002/psc.v15:4
- Chang M, Chou JC, Lee HJ. Cellular internalization of fluorescent proteins via arginine-rich intracellular delivery peptide in plant cells. Plant Cell Physiol. 2005;46:482–488.10.1093/pcp/pci046
- Keegstra K. Plant cell walls. Plant Physiol. 2010;154:483–486.10.1104/pp.110.161240
- Alberts B, Johnson A, Lewis J, et al. The plant cell wall. In: Molecular Biology of the Cell. 4th ed.. New York: Garland Science; 2002. p. 1118–1124.
- Roman K, Reinhold BB, Gilbert A. Architecture of the yeast cell wall. J. Biol. Chem. 1997;272:17762–17775.
- Lipke PN, Ovalle R. Cell wall architecture in yeast: new structure and new challenges. J. Bacteriol. 1998;180:3735–3740.
- Jigami Y, Odani T. Mannosylphosphate transfer to yeast mannan. Biochim. Biophys. Acta. 1999;1426:335–345.10.1016/S0304-4165(98)00134-2