Abstract
Phytocassanes and momilactones are the major diterpenoid phytoalexins inductively produced in rice as bioactive substances. Regardless of extensive studies on the biosynthetic pathways of these phytoalexins, bioconversion of diterpene hydrocarbons is not shown in planta. To elucidate the entire biosynthetic pathways of these phytoalexins, uniformly 13C-labeled ent-cassadiene and syn-pimaradiene were enzymatically synthesized with structural verification by GC–MS and 13C-NMR. Application of the 13C-labeled substrates on rice leaves led to the detection of 13C-labeled metabolites using LC-MS/MS. Further application of this method in the moss Hypnum plumaeforme and the nearest out-group of Oryza species Leersia perrieri, respectively, resulted in successful bioconversion of these labeled substrates into phytoalexins in these plants. These results demonstrate that genuine biosynthetic pathways from these diterpene hydrocarbons to the end product phytoalexins occur in these plants and that enzymatically synthesized [U-13C20] diterpene substrates are a powerful tool for chasing endogenous metabolites without dilution with naturally abundant unlabeled compounds.
Graphical abstract
Uniformly labeled 13C-diterpene: A powerful tool for tracing endogenous metabolites without dilution with naturally abundant unlabeled compounds
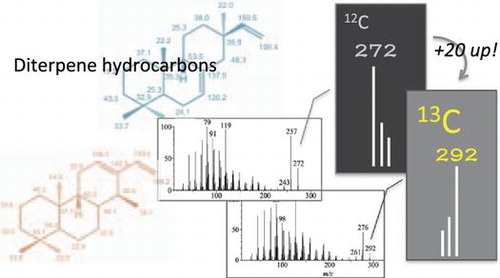
Plants exhibit self-defense response against pathogens and abiotic stress. One of their defense systems is the production of phytoalexins, which are low-molecular weight secondary metabolites (specialized metabolites) whose synthesis is induced by stressful conditions. In a well-studied model system, compounds of the diterpenoid phytoalexin family are produced in rice plants when they are infected with rice blast fungus or exposed to abiotic stress such as heavy metal ions or UV irradiation.Citation1,2) Diterpenoid phytoalexins, momilactones, phytocassanes, and oryzalexins are compounds in rice, with strong growth inhibition effects on micro-organisms and/or plants. In addition to rice, momilactones are also produced in the moss Hypnum plumaeforme as an allelopathic compound.Citation3) Furthermore, we recently reported that Leersia perrieri, the nearest outgroup of Oryza species produces distinctive types of phytocassanes (phytocassanes C and E).Citation4)
All diterpenoid phytoalexins in plants are biosynthesized in plastids from geranylgeranyl diphosphate (GGDP) via the 2C-methyl-d-erythritol 4-phosphate pathway. Committed steps of the biosynthesis of momilactones A and B are cyclization reactions of GGDP to syn-pimara-7,15-diene via syn-copalyl diphosphate, catalyzed by two diterpene cyclases OsCPS4 and OsKSL4 (Fig. ).Citation5) A bifunctional diterpene cyclase HpDTC1 having both of OsCPS4 and OsKSL4 activities is responsible for the production of syn-pimara-7,15-diene in H. plumaeforme.Citation6) However, the pathway from syn-pimara-7,15-diene to momilactones in H. plumaeforme is still largely unknown. Meanwhile, the biosynthesis of phytocassanes requires committed steps from GGDP to ent-cassa-12,15-diene via ent-copalyl diphosphate, which are catalyzed by two diterpene cyclases OsCPS2 and OsKSL7.Citation5–7) Although many parts of the biosynthetic pathways for both phytoalexins remain unclear, most of the biosynthetic genes responsible for the downstream steps in further conversions of these diterpene hydrocarbons have been tentatively determined.Citation6–9) Genes encoding P450 monooxygenases are involved in the oxidation step of syn-pimara-7,15-diene to syn-pimara-7,15-dienoic acid (CYP99A2 and A3) in momilactone biosynthesis and the oxidation step of ent-cassa-12,15-diene to phytocassanes (CYP71Z7, CYP76M7, M8). Several lines of evidence from both in vivo and in vitro experiments have shown that many P450 genes are putatively responsible for the biosynthesis of diterpenoid phytoalexins in rice.Citation11,12) However, there are still substrate–enzyme specificities among P450s that remain unclear and unexplained in phytoalexin biosynthesis.
Fig. 1. The putative biosynthetic pathways of phytocassanes and momilactones from GGDP.
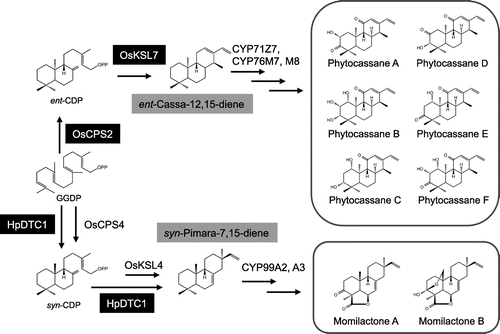
Feeding of labeled substrates to plant cells and the chasing of metabolites after bioconversion in planta is a promising approach for determining biosynthetic pathways of endogenous compounds. It is known that 13C-labeled compounds are useful for tracing the signals in metabolism, using mass spectrometry and NMR analyses. In a previous study, we reported that enzymatically synthesized [U-13C6] mevalonate (MVA) could be used as the starting point for in vitro synthesis of the diterpenoid phytohormone, gibberellin.Citation13) In another study, isotope-labeled compounds (ent-[17-3H2] kaurenoic acid or [18-2H1] GA12-aldehyde) were successfully fed to the pea Pisum sativum L. to trace the incorporation of ent-[17-3H2] kaurenoic acid or [18-2H1] GA12-aldehyde into the precursors of gibberellins.Citation14) Hence, a feeding approach with the use of 13C-labeled diterpene hydrocarbons is employed for the investigation of biosynthetic pathways of diterpenoid phytoalexins.
This study describes the feeding of biosynthetic precursors of phytocassanes and momilactones to rice leaves and the subsequent analysis of the metabolites to reveal the genuine biosynthesis occurring in rice. Two hydrocarbons, ent-cassa-12,15-diene and syn-pimara-7,15-diene, were enzymatically synthesized, and the carbon atoms were substituted with the 13C-isotope (>99%). The structures of these diterpenes were determined using GC–MS and 13C—13C COSY NMR spectroscopy. 13C-labeled ent-cassa-12,15-diene and syn-pimara-7,15-diene were incorporated into each end product (phytocassanes and momiactones, respectively), without dilution with naturally abundant unlabeled compounds. This evidence indicates that in planta biosynthetic pathways from these diterpenes definitely exist in rice. We also applied this feeding method in non-model plants that can also produce diterpenoid phytoalexins. Thus, enzymatic synthesis of uniform labeled diterpene precursors with 13C and feeding them to model and non-model plants are powerful tools for elucidating the possible diterpene biosynthetic pathways occurring in planta.
Materials and methods
Plant growth condition and chemicals
Rice Oryza sativa L. “Nipponbare” was used as the plant material in this study. Rice seeds were sterilized with 70% ethanol for 2 min and 5% HClO solution for 15 min after the removal of husks. They were then washed with sterile water, planted in 0.5% agar, and grown in a greenhouse at 28 °C. We also used the moss H. plumaeforme grown for one month as described by Okada et al. in 2016,Citation6) and L. perrieri, closely related to Oryza species, maintained under vegetative growth. 13C-labeled mevalonate used in this study was prepared with the support of ADEKA Co. Ltd.Citation13)
Enzymatic synthesis of [U-13C20] ent-cassa-12,15-diene and [U-13C20] syn-pimara-7,15-diene from [U-13C6] MVA in vitro
As previously reported, pQE30 vectors, each harboring one of the five ORFs encoding GGDP synthetic enzymes (NcMVK, NcPMVK, NcMVD, NcIPI, and SaGGPS) were used for the preparation of recombinant histidine-tagged (His-tag) fusion enzymes in E. coli JM109,Citation13) and these five recombinant enzymes were purified with HiTrap Chelating HP column (GE Healthcare, Uppsala, Sweden).
For the synthesis of ent-cassa-12,15-diene, two previously cloned diterpene biosynthetic genes, rice ent-copalyl diphosphate synthase 2 (OsCPS2, Os02t0571100-01) and rice ent-kaurene synthase-like 7 (OsKSL7, Os02t0570400-01) in pGEX-4T vectors were used to produce GST-fused proteins.Citation5,7,13,14) These two recombinant cyclases (0.8 mg of OsCPS2 and 1.0 mg of OsKSL7) were incubated with 2 mg of [U-13C6] MVA (hydrolyzed form) in a GGDP synthetic enzyme cocktail as described by Shimane et al. in 2014.Citation13) Following overnight incubation at 30 °C, the reaction products were extracted four times with n-hexane and concentrated in vacuo. The amount of [U-13C20] ent-cassa-12,15-diene from each synthetic experiment was estimated using an internal standard of ent-kaurene by GC–MS analysis. The total yield was reached at 0.86 mg of [U-13C20] ent-cassa-12,15-diene.
For the synthesis of syn-pimara-7,15-diene, the HpDTC1 gene in the moss H. plumaeforme, inserted in a pCold I expression vector (TAKARA, Shiga, Japan), was used for the production of a His-tagged HpDTC1 recombinant protein.Citation6) The recombinant HpDTC1 protein (2.7 mg) was added into the GGDP synthetic enzyme cocktail as described above. [U-13C6] MVA (hydrolyzed form, 1.5 mg) was applied to the above reaction mixture, and [U-13C20] syn-pimara-7,15-diene was retrieved (0.47 mg).
GC–MS and NMR analysis
[U-13C20] ent-cassa-12,15-diene and [U-13C20] syn-pimara-7,15-diene were analyzed using the Agilent 6890 N GC-5973 N MSD mass selective detector system (ionization voltage 70 eV) coupled with a capillary column InertCap 5MS/Sil (diameter 0.25 mm, length 15 m, film thickness 0.25 μm; GL Science Inc., Tokyo, Japan). The flow rate of the carrier gas (He) was controlled at 1 mL/min. The sample was injected into the injector at 250 °C. The oven temperature after injection was set as follows: held at 70 °C for 1 min, then increased by 10 °C/min to 280 °C, and finally held at 280 °C for 5 min. The mass fragmentations of both naturally occurring ent-cassa-12,15-diene and synthesized [U-13C20] ent-cassa-12,15-diene in GC–MS spectra were listed below: for natural ent-cassa-12,15-diene, m/z 272 (M+, 33%), 257 (75%), 243 (7%), 119 (97%), 91 (75%), 79 (100%); for [U-13C20] ent-cassa-12,15-diene, m/z 292 (M+, 12%), 276 (47%), 261 (7%), 128 (100%), 98 (62%), 85 (93%). Similarly, the mass fragmentations of both naturally occurring syn-pimara-7,15-diene and [U-13C20] syn-pimara-7,15-diene in GC–MS spectra were listed below: for natural syn-pimara-7,15-diene, m/z 272 (M+, 24%), 257 (60%), 243 (23%), 133 (42%), 119 (55%), 109 (100%); for [U-13C20] syn-pimara-7,15-diene, m/z 292 (M+, 6%), 276 (34%), 261 (17%), 143 (49%), 128 (61%), 117 (100%).
NMR analysis was performed using the JEOL JNM-A600 FT-NMR system. The samples [U-13C20] ent-cassa-12,15-diene and [U-13C20] syn-pimara-7,15-diene were dissolved in cyclohexane-d12 and benzene-d6, respectively, and each was placed in an NMR microtube (inner diameter 5 mm; Shigemi, Tokyo, Japan). One-dimensional and two-dimensional NMR spectral data for [U-13C20] ent-cassa-12,15-diene and [U-13C20] syn-pimara-7,15-diene were collected and processed using a previously described methodology.Citation13,15,16)
13C NMR data for ent-cassa-12,15-diene (150 MHz, CDCl2) δ: 14.3 (d, C-20, J10–20), 14.7 (d, C-17, J14–17), 19.5 (dd, C-2, J1–2, 2–3), 22.4 (dd, C-6, J5–6, 6–7), 22.5 (d, C-18, J4–18), 25.8 (dd, C-11, J9–11, 11–12), 31.9 (dd, C-7, J6–7, 7–8), 32.9 (ddd, C-14, J8–14, 13–14, 14–17), 33.7 (dddd, C-4, J3–4, 4–5, 4–18, 4–19), 33.9 (d, C-19, J4–19), 36.1 (ddd, C-8, J7–8, 8–9, 8–14), 37.7 (dddd, C-10, J1–10, 5–10, 9–10, 10–20), 40.2 (dd, C-1, J1–2, 2–10), 43.0 (dd, C-3, J2–3, 3–4), 45.2 (ddd, J8–9, 9–10, 9–11), 56.3 (ddd, C-5, J4–5, 5–6, 5–10), 109.2 (d, C-16, J15–16), 128.3 (dd, C-12, J11–12, 12–13), 139.4 (dd, C-15, J13–15, 15–16), 142.5 (ddd, C-13, J12–13, 13–14, 13–15).
[U-13C20] syn-pimara-7,15-diene (150 MHz, C6D6). δc: 19.2 (dd, C-2, J1–2,2–3), 22.0 (d, C-17, J13–17), 22.2 (d, C-20, J10–20), 22.9 (d, C-19, J4–19), 24.1 (dd, C-6, J5–6,6–7), 25.3 (ddd, C-5, J4–5,5–6,5–10), 25.3 (dd, C-11, J9–11,11–12), 32.9 (dddd, C-4, J3–4,4–5,4–18,4–19), 33.7 (d, C-18, J4–18), 35.3 (dddd, C-10, J1–10,5–10,9–10,10–20), 37.1 (dd, C-1, J1–2,1–10), 38.0 (dd, C-12, J11–12,12–13), 39.0 (dddd, C-13, J12–13,13–14,13–15,13–17), 43.3 (dd, C-3, J2–3,3–4), 48.3 (dd, C-14, J8–14, 13–14), 53.5 (ddd, C-9, J8–9,9–10,9–11), 109.4 (d, C-16, J15–16), 120.2 (dd, C-7, J6–7,7–8), 137.0 (ddd, C-8, J7–8,8–9,8–14), 150.5 (dd, C-15, J13–15,15–16).
Feeding of [U-13C20] ent-cassa-12,15-diene and [U-13C20] syn-pimara-7,15-diene in plants
Rice plants (Oryza sativa L. “Nipponbare”), grown for six days after germination, were used for the feeding experiment. Fifteen micrograms of the [U-13C20] ent-cassadiene substrate (1 μg/μL, dissolved in 99.5% v/v ethanol) were applied to one expanded leaf, and placed on cotton gauze soaked with 0.5 mM CuCl2 solution to induce the expression of phytoalexin biosynthetic enzymes. After incubating for 72 h, the leaf was excised and phytoalexins were extracted with 0.5 mL of 100% v/v methanol. The sample was then centrifuged (4 °C, 10 min, 15,000 ×g). The supernatant was collected and analyzed by LC-MS/MS.
The [U-13C20] syn-pimaradiene feeding experiment was performed in a similar manner to the [U-13C20] ent-cassadiene experiment, except for the leaf material used. Fresh leaf disks (diameter 6 mm) prepared from expanded leaves from 8-month-old rice plants were used. One microgram of the [U-13C20] syn-pimaradiene substrate (1 μg/μL, dissolved in 99.5% v/v ethanol) was applied to each leaf disk placed on cotton gauze soaked with 0.5 mM CuCl2 solution in a microtiter plate. After a 72-h incubation period, 24 leaf disks were collected, and subjected to phytoalexin extraction with 8 mL of 100% v/v methanol. The extract was centrifuged, and the concentrated supernatant collected and analyzed by LC-MS/MS.
A wild specimen of L. perrieri was used for the feeding experiments. Nine micrograms of [U-13C20] ent-cassa-12,15-diene (1 μg/μL, dissolved in 99.5% v/v ethanol) were applied to L. perrieri leaves and incubated in a capped glass tube (diameter 38 mm) for 72 h with cotton gauze soaked in 0.5 mM CuCl2 solution. After the incubation, each leaf was extracted with 0.5 mL of 100% v/v methanol for 48 h in 4 °C. The extracts were centrifuged and the supernatant was analyzed with LC-MS/MS.
The moss H. plumaeforme was fed with [U-13C20] syn-pimara-7,15-diene. The substrate [U-13C20] syn-pimara-7,15-diene (1 μg/μL, dissolved in 99.5% v/v ethanol) was fed to the moss gametophores (30 mg per well of microtiter plate). The treated gametophores were placed on cotton gauze soaked with 150 μL CuCl2 on a microtiter plate and incubated for 72 h. The moss gametophores collected from eight wells were combined and extracted with 6 mL of 100% v/v methanol for 48 h in 4 °C. The extracts were centrifuged and the supernatant was concentrated and subjected to LC-MS/MS analysis.
Determination of phytoalexins
Metabolites in plants fed with [U-13C20] ent-cassa-12,15-diene or [U-13C20] syn-pimara-7,15-diene were extracted from the leaf samples using 100% v/v methanol. The methanol extracts were concentrated to 100 μL in vacuo, and 5 μL of the concentrated extract was subjected to phytoalexin analysis by LC-MS/MS, comprising an API-3000 spectrometer with an electrospray ion source and an Agilent 1100 HPLC instrument equipped with a Pegasil ODS SP100 C18 column (length 150 mm, diameter 2.1 mm; Senshu Scientific Co., Ltd, Japan), as previously described.Citation17)
Results
Enzymatic synthesis of 13C-labeled diterpene precursors for diterpenoid phytoalexins and assignment of their NMR signals
In this study, we established a 13C-labeling-based approach, which can be useful for elucidating the biosynthetic pathway for rice diterpenoid phytoalexins. The fully 13C-labeled ent-cassa-12,15-diene and syn-pimara-7,15-diene, which are putative precursors of phytocassanes and momilactones, respectively, were enzymatically synthesized from [U-13C20] GGDP according to our previously reported methodologies.Citation7,13) The synthesis of ent-cassa-12,15-diene from GGDP required two monofunctional diterpene cyclases, OsCPS2 and OsKSL7, the former involved in ent-CDP synthesis from GGDP, and the latter in the synthesis of ent-cassa-12,15-diene from ent-CDP.Citation7,11) The synthesis of syn-pimara-7,15-diene from GGDP can be performed using HpDTC1, a diterpene cyclase identified from the moss H. plumaeforme involved in sequential two-step cyclizations from GGDP to syn-pimara-7,15-diene via syn-CDP (Fig. ).Citation6) Affinity-purified enzymes used in either of the reactions were shown in Fig. . The total yields of labeled ent-cassa-12,15-diene and syn-pimara-7,15-diene from mevalonate reached approximately 90 and 66%, respectively.
Fig. 2. Affinity purified enzymes for in vitro synthesis of [U-13C20] ent-cassa-12,15-diene and [U-13C20] syn-pimara-7,15-diene.
![Fig. 2. Affinity purified enzymes for in vitro synthesis of [U-13C20] ent-cassa-12,15-diene and [U-13C20] syn-pimara-7,15-diene.](/cms/asset/84ac846e-e215-4eba-9e80-402214acc5b3/tbbb_a_1285689_f0002_b.gif)
The GC–MS chromatograms showed unique peak, and the spectra of naturally occurring ent-cassa-12,15-diene and synthesized [U-13C20] ent-cassa-12,15-diene showed identical patterns, even though each molecular ion peak and the fragment ion peaks were shifted depending on the number of 13C stable isotope (Fig. (A)). Similarly, the GC–MS chromatograms showed unique peak, and the spectra of naturally occurring syn-pimara-7,15-diene and [U-13C20] syn-pimara-7,15-diene were identical, although each molecular ion peak and the fragment ion peaks were shifted depending on the number of 13C stable isotope (Fig. (B)).
Fig. 3. GC-MS analyses of [U-13C20] ent-cassa-12,15-diene and [U-13C20] syn-pimara-7,15-diene.
![Fig. 3. GC-MS analyses of [U-13C20] ent-cassa-12,15-diene and [U-13C20] syn-pimara-7,15-diene.](/cms/asset/41e0b333-99af-4535-ba36-d8888dea747e/tbbb_a_1285689_f0003_b.gif)
The one-dimensional 13C-NMR spectra could obtain a clear 20-carbon signal derived from both [U-13C20] ent-cassa-12,15-diene and [U-13C20] syn-pimara-7,15-diene (Fig. (A) and (D)). The two-dimensional 13C-NMR spectra of both labeled compounds clearly showed carbon—carbon connectivity, and all carbons could be assigned (Fig. (B) and (E)). Thus, the structures of [U-13C20] ent-cassa-12,15-diene and [U-13C20] syn-pimara-7,15-diene were determined, as shown in Fig. (C) and (F). Therefore, we were able to successfully prepare biosynthetic precursors labeled with 13C isotope for chasing the metabolites leading to diterpenoid phytoalexins.
Fig. 4. NMR spectra of [U-13C20] ent-cassa-12,15-diene and [U-13C20] syn-pimara-7,15-diene.
![Fig. 4. NMR spectra of [U-13C20] ent-cassa-12,15-diene and [U-13C20] syn-pimara-7,15-diene.](/cms/asset/40be9f32-3d19-4c9e-aa5e-9636694cf5b6/tbbb_a_1285689_f0004_oc.gif)
Tracking of the biosynthetic pathway of diterpenoid phytoalexins by feeding of [U-13C20] ent-cassa-12,15-diene or [U-13C20] syn-pimara-7,15-diene on rice plants
To investigate the in planta bioconversion of the diterpene hydrocarbon precursor into phytoalexins, both labeled precursors were fed into leaf disks (6 mm diameter) or young leaves (30 mm in length) of wild-type rice plants (O. sativa “Nipponbare”), and analyzed by LC-MS/MS, to confirm the presence of 13C-labeled compounds. The combinations of molecular ion and precursor ion of m/z (315/271) for momilactone A; m/z (331/269) for momilactone B; m/z (316/299) for phytocassanes A, D, and E; m/z (335/317) for phytocassane B; and m/z (319/301) for phytocassane C were used for the detection of unlabeled naturally occurring phytoalexins. For the detection of [U-13C20] momilactone A and [U-13C20] momilactone B, the molecular ion peak and the fragment ion peaks, which are shifted according to the number of 13C stable isotope present, were used: m/z (335/290) for [U-13C20] momilactone A and m/z (351/288) for [U-13C20] momilactone B.
As shown in Fig. (A), the inductions of unlabeled momilactones and phytocassanes were detected in leaf disks under these experimental conditions. After the application of [U-13C20] syn-pimara-7,15-diene, [U-13C20] momilactone A and [U-13C20] momilactone B were detected to be accumulated in the leaf disk (Fig. (B) left). Similar to those of [U-13C20] momilactones, combinations of molecular ions and precursor ions of [U-13C20] phytocassanes were set as follows: m/z (336/319) for [U-13C20] phytocassane A, D, and E; m/z (355/337) for [U-13C20] phytocassane B; and m/z (339/321) for [U-13C20] phytocassane C. One fresh leaf blade was used for the application of 15 μg [U-13C20] ent-cassa-12,15-diene (as 15 μL of ethanol solution) in the feeding experiment. LC-MS/MS analysis clearly revealed that major phytocassanes (phytocassane B, C, and E) were also detected as 13C-labeled compounds (Fig. (B) right). Accumulation levels of 13C-labeled and unlabeled phytoalexins are shown in Figure S1. These results suggest in planta conversion in rice plants of momilactones and phytocassanes from syn-pimara-7,15-diene and ent-cassa-12,15-diene, respectively. Therefore, the biosynthetic pathways of diterpenoid phytoalexins from these diterpenes are definitely active in rice.
Fig. 5. LC-MS/MS analysis of the incorporation of 13C-diterpene hydrocarbons into momilactones and phytocassanes in rice plant.
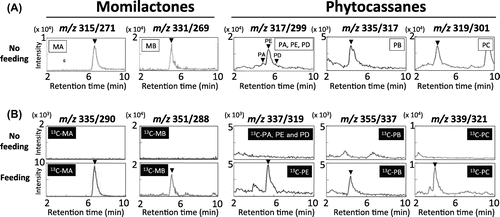
Investigation of phytoalexin biosynthetic pathways in wild plants capable of producing diterpene phytoalexins
In a recent study, we reported findings of the evolutionary trajectory of diterpenoid phytoalexin biosynthetic genes in wild rice.Citation16) In that, we also found that L. perrieri could produce distinct two types of phytocassanes (phytocassane C and E), and that homologous genes presumably involved in phytocassane biosynthesis exist in the L. perrieri genome. However, there was no evidence that L. perrieri possesses the same biosynthetic route for phytocassanes as seen in O. sativa. To investigate whether the in planta biosynthetic pathway for the synthesis of phytocassanes C and E is maintained in L. perrieri, we used our feeding approach with [U-13C20] ent-cassa-12,15-diene.
When [U-13C20] ent-cassa-12,15-diene was fed to leaf blades of L. perrieri and incubated with CuCl2 for 72 h, 13C-labeled phytocassane C and phytocassane E were detected during LC-MS/MS analysis, along with unlabeled phytocassanes (Fig. (A)). This result suggests that the biosynthetic pathway of phytocassanes is derived from ent-cassadiene, as seen in O. sativa, is definitely maintained in L. perrieri. In another feeding experiment, we analyzed the incorporation of [U-13C20] syn-pimara-7,15-diene into [U-13C20] momilactone A and [U-13C20] momilactone B in the moss H. plumaeforme, which we recently reported as a momilactone producer with the identification of pimaradiene synthase gene HpDTC1 other than Oryza species.Citation6) H. plumaeforme can produce both momilactone A and momilactone B, but the latter is accumulated in higher amounts and exhibits stronger allelopathic effects on surrounding plants. As shown in Fig. (B), the feeding of [U-13C20] syn-pimara-7,15-diene obviously resulted in a distinct accumulation of [U-13C20] momilactone B and [U-13C20] momilactone A (in a smaller amount) in gametophores of H. plumaeforme, as detected by LC-MS/MS analysis. This indicates that H. plumaeforme possesses the biosynthetic pathway of momilactones derived from syn-pimaradiene.
Fig. 6. LC-MS/MS analysis of incorporation of 13C-diterpene hydrocarbons into phytocassanes in L. perrieri and momilactones in H. plumaeforme.
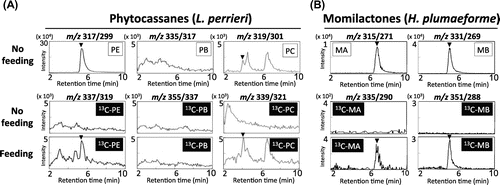
Discussion
There have been intensive efforts to elucidate the biosynthetic pathways of diterpenoid phytoalexins in rice. It has been demonstrated that rice mutants defective in the expression of OsCPS4 or OsKSL4 genes responsible for the production of syn-pimaradiene exhibit a reduction in endogenous momilactone production,Citation9,10) suggesting that syn-pimaradiene could be a possible intermediate in momilactone biosynthesis. ent-Cassa-12,15-diene, a precursor for phytocassanes, is believed to be a promising intermediate for the phytocassane biosynthetic pathway because of its inductive accumulation in rice and demonstrated in vitro conversion by P450 monooxygenase to a possible phytocassane intermediate.Citation7,18–20) We, herein, enzymatically synthesized both 13C-labeled ent-cassadiene and syn-pimaradiene, and obtained in planta evidence that they are involved in the biosynthetic pathways for phytocassanes and momilactones, respectively.
To synthesize the 13C-diterpene hydrocarbon, we first obtained 13C-MVA according to our in vitro reaction system from 13C-acetate, described in a previous publication.13) The starting material 13C-MVA was further converted into ent-cassadiene or syn-pimaradiene via geranylgeranyl diphosphate (GGDP) with the aid of a series of recombinant enzymes for the production of isopentenyl diphosphate and dimethylallyl diphosphate (both building blocks of terpenoids), GGDP synthase, and diterpene cyclases involved in the production of ent-cassadiene (OsCPS2 and OsKSL7) and syn-pimaradiene (HpDTC1) (Fig. ). The conversion efficiency of the substrate 13C-MVA into these diterpene hydrocarbons was more than 60%, implying that all of the in vitro reaction steps, including diterpene cyclization, must be appropriately conducted.
Using the prepared 13C-labeled diterpene hydrocarbons, we clearly showed 13C labeling of the end products of the pathway in our feeding experiment (Figs. and ). As the next step, it is important to find as-yet-unknown intermediates of the pathway, and our feeding system could be used for this. However, the incorporation of 13C-diterpene hydrocarbons into the diterpene phytoalexins was somewhat limited in the present study (Table S1). To overcome this issue, it would be possible to use AMO-1618, a potent chemical inhibitor for CPS enzymes, which would repress the metabolic flow leading to a natural abundance of cassadiene and momilactone, thus enhancing the incorporation of 13C-diterpene hydrocarbons into these phytoalexins. An alternative way to enhance the 13C incorporation in plants would be using genetically modified plants that are defective in the production of syn-pimaradiene or ent-cassadiene.
In this study, in addition to the evidence of the biosynthetic pathway leading to ditepenoid phytoalexins from ent-cassadiene and syn-pimaradiene in rice, we also successfully traced the 13C-signal in L. perrieri (a phytocassanes producer) and H. plumaeforme (a momilactone-producing moss). Previously, we reported that L. perrieri could produce non-C2-hydroxylated-type phytocassane C and phytocassane E, but not C2-hydroxylated types of phytocassane A, phytocassane B, and phytocassane D (Fig. ), suggesting that C2-hydroxylation does not occur in L. perrieri.Citation4) Our labeling experiment showed that phytocassane C and phytocassane E were the only 13C-labeled phytocassanes detected, further supporting the existence of a distinctive biosynthetic pathway for phytocassanes in L. perrieri.
In the experiment with the moss H. plumaeforme, we confirmed that 13C-labeled syn-pimaradiene was successfully incorporated into momilactones A and B. In our previous report, a bifunctional syn-pimaradiene synthase gene HpDTC1 was identified on the basis of RNA-seq analysis of CuCl2-treated moss gametophore.Citation6) Since syn-pimaradiene accumulation in moss treated with CuCl2 was not observed, while it was clearly detected in rice leaves after UV irradiation, the metabolic flow via syn-pimaradiene in H. plumaeforme might be rather faster compared to that in rice. Besides, the subsequent biosynthetic pathway consisting of P450 monooxygenases in the moss seems to be somehow different from the pathway in rice. CYP99A2 and A3, or CYP71Z7, CYP76M7, and M8 have all been shown to be responsible for either momilactone or phytocassane production in rice; however, similar homologs with high homology were not found in the RNA-seq data. Seeking 13C-labeled intermediates of 13C-pimaradiene might be an approach that would be able to find a biosynthetic pathway committed by as-yet-unknown P450 enzymes distinctive to the moss. In order to understand the in vivo conversion is really committed by the P450 enzymes, we currently plan to perform our feeding experiment with rice mutants defective in P450 monooxygenases CYP71Z7 or CYP76M7/M8 as well. Alternatively, further studies could also use biotransformed 13C-labeled compounds and focus on the degradation of diterpenoid phytoalexins in the natural environment, which might occur in the rhizosphere around plants producing such bioactive diterpenoid phytoalexins.
In conclusion, our feeding approach using uniformly labeled 13C-diterpene substrates is a powerful tool, which will aid in elucidating the biosynthetic pathways of diterpenoid phytoalexins in all plants, and determining the unknown precursors or metabolites of diterpenoid phytoalexins in future studies.
Author contribution
K.O., H.K. designed the study; Z.Y., K.N. performed the experiments and data analyses; K.O., H.K., Z.Y. wrote manuscript with the assistance of H.N., M.N. K.O. supervised the study.
Disclosure statement
No potential conflict of interest was reported by the authors.
Supplemental materials
Supplemental material for this article can be accessed at http://dx.doi.org/10.1080/09168451.2017.1285689
Funding
This work was supported in part by JSPS KAKENHI to KO [grant number 26450136] and to HK [grant number 15K07407].
TBBB_1285689_Supplementary_Files.zip
Download Zip (235.2 KB)Acknowledgments
We appreciate ADEKA Co. Ltd. for supplying us with 13C-MVA and technical support. We are grateful to Dr. Nori Kurata (National Institute of Genetics) for providing us with L. perrieri plants used in this study and to Professors Hisakazu Yamane, Tomonobu Toyomasu, and Dr. Koji Miyamoto for a critical reading of the manuscript.
References
- VanEtten HD, Mansfield JW, Bailey J, et al. Two classes of plant antibiotics: phytoalexins versus phytoanticipins. Plant Cell. 1994;6:1191–1192.10.1105/tpc.6.9.1191
- Dixon RA. Natural products and plant disease resistance. Nature. 2011;411:843–847.
- Nozaki H, Hayashi K, Nishimura N, et al. Momilactone A and B as allelochemicals from moss hypnum plumaeforme: first occurrence in bryophytes. Biosci Biotechnol Biochem. 2007;71(12):3127–3130.10.1271/bbb.70625
- Miyamoto K, Fujita M, Shenton MR, et al. Evolutionary trajectory of phytoalexin biosynthetic gene clusters in rice. Plant J. 2016;87(3):293–304.10.1111/tpj.2016.87.issue-3
- Otomo K, Kanno Y, Motegi A, et al. Diterpene cyclases responsible for the biosynthesis of phytoalexins, momilactones A, B, and oryzalexins A–F in rice. Biosci Biotechnol Biochem. 2004;68(9):2001–2006.10.1271/bbb.68.2001
- Okada K, Kawaide H, Miyamoto K, et al. HpDTC1, a stress-inducible bifunctional diterpene cyclase involved in momilactone biosynthesis, functions in chemical defence in the moss Hypnum plumaeforme. Sci Rep. 2016;6:25316.10.1038/srep25316
- Cho EM, Okada A, Kenmoku H, et al. Molecular cloning and characterization of a cDNA encoding ent-cassa-12,15-diene synthase, a putative diterpenoid phytoalexin biosynthetic enzyme, from suspension-cultured rice cells treated with a chitin elicitor. Plant J. 2004;37:1–8.10.1046/j.1365-313X.2003.01926.x
- Kanno Y, Otomo K, Kenmoku H, et al. Characterization of a rice gene family encoding type-A diterpene cyclases. Biosci Biotechnol Biochem. 2006;70(7):1702–1710.10.1271/bbb.60044
- Peters RJ. Uncovering the complex metabolic network underlying diterpenoid phytoalexin biosynthesis in rice and other cereal crop plants. Phytochemistry. 2006;67(21):2307–2317.10.1016/j.phytochem.2006.08.009
- Toyomasu T. Recent advances regarding diterpene cyclase genes in higher plants and fungi. Biosci Biotechnol Biochem. 2008;72(5):1168–1175.10.1271/bbb.80044
- Okada K. The biosynthesis of isoprenoids and the mechanisms regulating it in plants. Biosci Biotechnol Biochem. 2011;75(7):1219–1225.10.1271/bbb.110228
- Schmelz EA, Huffaker A, Sims JW, et al. Biosynthesis, elicitation and roles of monocot terpenoid phytoalexins. Plant J. 2014;79(4):659–678.10.1111/tpj.2014.79.issue-4
- Sugai Y, Miyazaki S, Mukai S, et al. Enzymatic total synthesis of gibberellin A4 from acetate. Biosci Biotechnol Biochem. 2011a;75(1):128–135.10.1271/bbb.100733
- Ingram TJ, Reid JB. Internode length in pisum: gene na may block gibberellin synthesis between ent-7alpha-hydroxykaurenoic acid and gibberellin A12-aldehyde. Plant Physiol. 1987;83(4):1048–1053.10.1104/pp.83.4.1048
- Shimane M, Ueno Y, Morisaki K, et al. Molecular evolution of the substrate specificity of ent-kaurene synthases to adapt to gibberellin biosynthesis in land plants. Biochem J. 2014;462(3):539–546.10.1042/BJ20140134
- Sugai Y, Ueno Y, Hayashi K, et al. Enzymatic (13)C labeling and multidimensional NMR analysis of miltiradiene synthesized by bifunctional diterpene cyclase in Selaginella moellendorffii. J Biol Chem. 2011b;286(50):42840–42847.10.1074/jbc.M111.302703
- Shimizu T, Jikumaru Y, Okada A, et al. Effects of a bile acid elicitor, cholic acid, on the biosynthesis of diterpenoid phytoalexins in suspension-cultured rice cells. Phytochemistry. 2008;69:973–981.10.1016/j.phytochem.2007.10.005
- Swaminathan S, Morrone D, Wang Q, et al. CYP76M7 is an ent-cassadiene C11alpha-hydroxylase defining a second multifunctional diterpenoid biosynthetic gene cluster in rice. Plant Cell. 2009;21(10):3315–3325.10.1105/tpc.108.063677
- Wang Q, Hillwig ML, Okada K, et al. Characterization of CYP76M5-8 indicates metabolic plasticity within a plant biosynthetic gene cluster. J Biol Chem. 2012;287(9):6159–6168.10.1074/jbc.M111.305599
- Kitaoka N, Wu Y, Xu M, et al. Optimization of recombinant expression enables discovery of novel cytochrome P450 activity in rice diterpenoid biosynthesis. Appl Microbiol Biotechnol. 2015;99(18):7549–7558.10.1007/s00253-015-6496-2