Abstract
Schizophyllum commune is a basidiomycete equipped with an efficient cellulolytic enzyme system capable of growth on decaying woods. In this study, production of lignocellulose-degrading enzymes from S. commune mutant G-135 (SC-Cel) on various cellulosic substrates was examined. The highest cellulase activities including CMCase, FPase, and β-glucosidase were obtained on Avicel-PH101 while a wider range of enzymes attacking non-cellulosic polysaccharides and lignin were found when grown on alkaline-pretreated biomass. Proteomic analysis of SC-Cel also revealed a complex enzyme system comprising seven glycosyl hydrolase families with an accessory carbohydrate esterase, polysaccharide lyase, and auxiliary redox enzymes. SC-Cel obtained on Avicel-PH101 effectively hydrolyzed all agricultural residues with the maximum glucan conversion of 98.0% using corn cobs with an enzyme dosage of 5 FPU/g-biomass. The work showed potential of SC-Cel on hydrolysis of various herbaceous biomass with enhanced efficiency by addition external β-xylosidase.
Graphical abstract
Enzymatic hydrolysis of alkaline-pretreated biomass by Sc-Cel and yield enhancement by external β-xylosidase (BX).
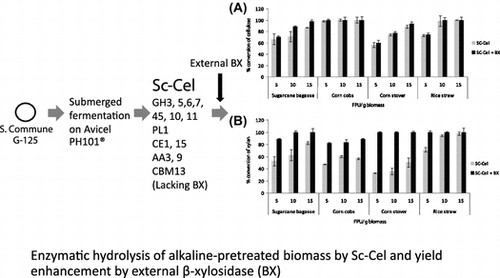
Lignocellulosic biomass is a promising renewable starting material for production of biofuels and commodity chemicals with the key advantage on its carbon-neutral nature. Lignocellulose is a complex multi-component structure consisted mainly of three types of biopolymers: (i) cellulose, a linear homopolymer of glucose organized into highly crystalline microfibers; (ii) hemicellulose, an amorphous branched heterogenous polysaccharide composed of pentoses, hexoses, and sugar acids acting as linkages between the cellulose fibers; and (iii) lignin, a heteropolymer of phenolic alcohols which shields cell wall polysaccharides from external physical, chemical, and biological attacks.Citation1) Due to its enzymatic-resistant structure, hydrolysis of lignocelluloses to sugars is a major bottleneck of biorefinery process in term of yield and titer. Thus, the search for an efficient and versatile cellulolytic enzyme system for saccharification of diverse lignocellulosic substrates of different types and origins is essential for developing an economic feasible biorefinery industry.
In nature, decomposition of plant biomass involves cooperative actions of a variety of microorganisms, particularly bacteria and fungi, under different environmental conditions. Lignocellulose-degrading enzymes e.g. cellulases and hemicellulases from various ascomycetes, for example, genera Trichoderma, Aspergillus, and Talaromyces, have been extensively studied and applied for a variety of industrial processes related to biomass saccharification and modification.Citation2,3) Fungi in phylum of Basidiomycota or mushroom-forming fungi play an important role in organic carbon recycling in aerobic ecosystem; however, studies of their biomass-degrading enzyme systems for bio-industry application are relatively limited. Schizophyllum commune is commonly found on woods and plant waste piles and used as food in many Asian countriesCitation4). For the molecular biology point of view, S. commune is considered an important model organism for genetic study of mushroom-forming fungi and its genome has been elucidated.Citation5) On the other hand, the ability of S. commune to produce lignocellulose-degrading enzymes has been firstly reported by Jurasek et al. since 1968.Citation6) Study on optimization of cellulase and xylanase from this fungal species and their biochemical characteristics were later reported.Citation7,8) Application of S. commune for ethanol production from wood chips by consolidated bioprocessing has been demonstrated.Citation9) Analysis of its genome and secretome revealed that it contained a large array of enzymes attacking lignocelluloses, including a variety of core and accessory hydrolytic enzymes in different glycosyl hydrolase (GH) families as well as various non-hydrolytic polysaccharide degrading enzymes and ligninases with different selectivities and catalytic mechanisms working cooperatively on attacking lignocelluloses.Citation5,10) However, studies on cellulase production capability by S. commune and its applicability on saccharifying agricultural wastes in biorefinery have been limited while more in-depth study on its complex biomass degrading enzyme system is required in order to develop its enzyme system for further biotechnological application.
According to our extensive screening program on plant litter and wood decay fungi including ascomycetes, basidiomycetes and others, S. commuue BCC23363 has been identified as a potent biomass-degrading enzyme producer. To develop an efficient candidate for future on-site cellulolytic enzyme production in biorefinery industry, the strain was further improved by random mutation. This resulted in selection of a stable mutant G-135 which exhibited a higher capability on cellulase and xylanase production compared to the wild-type strain. In this study, cellulolytic enzyme production of S. commune G-135 on different carbon sources using aerobic submerged fermentation was investigated. The secreted enzyme complex (SC-Cel) was characterized for their biochemical properties and the composite proteomic profile of the fungal secretome was analyzed. The performance of SC-Cel on hydrolysis of various local agricultural residues for biorefinery industry was also evaluated. This work demonstrated the potential of S. commune cellulase system as an efficient alternative for biomass saccharification and modification in bio-industries.
Materials and methods
Materials and strains
Sugarcane bagasse was obtained from Phu Kiaew Bioenergy, Chaiyaphoom, Thailand. Rice straw was collected from a local paddy field in Pathumthani, Thailand. Corn cobs and corn stover were kindly provided by the National Corn and Sorghum Research Center, Nakorn Ratchasrima, Thailand. The biomass was physically processed using a cutting mill (Retsch SM 2000, Hann, Germany) and simultaneously sieved to particles size of 0.5–1.0 mm (18–35 mesh). The processed biomass with this size range (more than 98% of the starting material) was then used as a starting material for experimental studies. The biomass was pretreated using 0.5 g/L NaOH with a solid and liquid ratio of 1:3 and heated at 90 °C for 90 min under air atmosphere. The solid was washed with tap water until neutral pH and dried at 50 °C. Chemical composition of the raw materials and the pretreated biomass according to National Renewable Energy Laboratory (NREL) analytical proceduresCitation11) are shown in Table . S. commune BCC23363 was obtained from the BIOTEC Culture Collection (www.biotec.or.th/bcc). This strain was identified as a potent biomass-degrading enzyme producer based on screening of the total of 222 fungal strains comprising 34 genera and 50 unidentified fungus previously isolated from plant litter and decayed wood samples collected from different regions of Thailand (supplementary Table S1). All fungal isolates were cultivated in 3% (w/v) wheat bran, 1% (w/v) soybean, and 40 g/L peptone under aerobic conditions at 30 °C for 7 d and screened for the ability to produce lignocellulolytic enzymes including endo-glucanase (CMCase), xylanase, mannanase, and laccase (as stated below). Thermostable β-xylosidase from Aspergillus sp. BCC125 was produced as a secreted enzyme in recombinant Pichia pastoris KM71 culture and used in the complementation experiment with no prior chromatographic purification.Citation12)
Table 1. Cellulose, hemicellulose, and lignin content of native and alkaline pretreated lignocellulosic biomass.
Isolation of G-135 cellulase hyper-producing strain
Mutants with increased cellulase production efficiency were generated by random mutagenesis using UV-induced mutation according to the method described by Fujii et al.Citation13) S. commune BCC23363 was cultured for 16 h in 500 mL Erlenmeyer flask containing 100 mL of GY medium (3% glucose and 2% yeast extract) and incubated at 30 °C with shaking at 120 rpm. The fungal mycelium was harvested and suspended in 50 mL of 10 mM sodium phosphate buffer, pH 6.5 containing 2% (w/v) Lysing enzyme from Trichoderma harzianum (Sigma Aldrich, St. Louis, MO, USA) and 0.5% (w/v) Driselase (Sigma Aldrich) and gently agitated at 30 °C for 2 h. The resulting protoplasts were centrifuged at 750×g for 10 min and resuspended in 3 mL of 10 mM Tris-HCl buffer pH 7.5 containing 1.2 M sorbitol. The protoplast suspension (107 CFU/mL) was gently agitated by magnetic stirrer in 30 mm plate and UV irradiated (UV light intensity of 100 μW/cm2) for 8 min. The UV irradiated protoplasts were regenerated on potato dextrose agar (BD Difco, Franklin Lakes, NJ, USA) containing 1 M sucrose and then pick up for the screening of cellulolytic enzyme production. The mutants were screened for cellulolytic activity using Avicel-PH101 medium (40 g/L Avicel-PH101, 0.1 g/L peptone, 0.005 g/L yeast extract, 0.014 g/L (NH4)2SO4, 0.24 g/L KH2PO4 0.003 g/L CaCl2 and 0.003 g/L MgSO47H2O) and cultivated at 30 °C with rotary shaking at 200 rpm for 5 d. The fungal supernatant was harvested by centrifugation at 6,500×g and further assayed for filter paper degrading activity for cellulose (FPase) using dinitrosalicylic acid (DNS method).Citation14,15) The mutant strain S. commune G-135 with the highest improvement in the cellulase activities was isolated from the mutant screening process. The strain was maintained on potato dextrose agar and used in all experimental studies.
Cellulolytic enzyme production
S. commune G-135 was cultivated aerobically in submerged fermentation by inoculating five plugs (plunging by a cork borer No. 2) of the fungal mycelium grown on PDA into 50 mL of the enzyme production basal medium (0.1 g/L peptone, 0.005 g/L yeast extract, 0.014 g/L (NH4)2SO4, 0.24 g/L KH2PO4, 0.003 g/L CaCl2, 0.003 g/L MgSO4.7H2O) with 4% (w/v) Avicel-PH101 or 4% (w/v) of alkaline pretreated agricultural residues (sugarcane bagasse, corn cobs, corn stover, or rice straw pretreated as mentioned above) as the main carbon source. The cultures were incubated at 30 °C with rotary shaking at 200 rpm for 7 d. The crude enzymes prepared using Avicel-PH101 (AC), sugarcane bagasse (BA), corn cobs (CC), corn stover (CS), and rice straw (RS) were harvested by separating the fungal mycelium through a muslin cloth and clarified by centrifugation at 6500×g for 10 min at 4 °C and kept at 4 °C for subsequent studies.
Enzyme activity analysis
All enzyme activities were measured according to the standard procedure of the Commission on Biotechnology, IUPAC with some modifications.Citation14–16) FPase expressed as Filter paper unit (FPU) was analyzed using Whatman No.1 filter paper stripe (1 × 6 cm) as a substrate and incubated at 50 °C in 50 mM sodium acetate buffer (pH 5.0) for 60 min. The CMCase, xylanase, mannanase, and polygalacturonase (PGase) activity assays were performed using 2% (w/v) carboxymethyl cellulose (CMC), 2% (w/v) birchwood xylan, 1% (w/v) locust bean gum, and 1% (w/v) pectin from citrus peel as the substrates, respectively in 50 mM sodium acetate buffer pH 5.0 and incubated at 50 °C for 30 min. The released reducing sugars were measured using the DNS method. One unit (U) or FPU was defined as the amount of enzyme that produced 1 μmole of reducing sugar equivalence as glucose, xylose, mannose, or galacturonic acid per min under the assay conditions. The β-glucosidase and β-xylosidase activities were analyzed at 50 °C in 50 mM sodium acetate buffer (pH 5.0) for 60 min according to the standard method recommended by IUPAC.Citation16) Laccase activity was determined using ABTS (2, 2′-azinobis 3-thylbenzothiazoline-6-sulfonic acid) as a substrate with an extinction coefficient of 36,000 M−1cm−1 at 30 °C in 50 mM sodium acetate buffer (pH 5.0) for 30 min.Citation17) One unit of laccase activity was expressed as the amount of enzyme that produced 1 nmole of ABTS free radicals in one min. Protein concentration was measured using the Bio-Rad’s Protein Assay Reagent (Bio-Rad, Hercules, CA, USA) based on the Bradford’s method.Citation18)
Proteomic analysis of S. commune G-135 secretome
The crude S. commune enzyme was applied to a 10% SDS-PAGE using a MiniProtean Tetra system (Bio-Rad). The protein bands were visualized using Coomassie blue R-250 staining and were manually excised into fractions according to their apparent molecular weights (up to 116.0 kDa). The polypeptides were then in-gel digested with trypsin (Ettan Spot Handling Workstation User Manual 18-1153-55 Edition AC, GE Healthcare Biosciences, Uppsala, Sweden). Nanoscale LC separation of tryptic peptides was performed with a Nano Acquity system (Waters Corp., Milford, MA, USA) equipped with a Symmetry C18 5 μm, 180-μm × 20-mm Trap column and a BEH130 C18 1.7 μm, 100-μm × 100-mm analytical reversed phase column (Waters Corp., Milford, MA, USA). The tryptic peptides were analyzed on a SYNAPT HDMS mass spectrometer (Waters Corp.). All MS/MS spectra were searched using the Mascot® search engine (Matrix Science, Boston, MA, USA) against the NCBI-nr database using the following criteria: enzyme trypsin, static modification of cysteine (+57.05130 Da), and differential modification of methionine (+15.99940). The search results were filtered by cross-correlation versus charge state (+1 ≥ 1.5, +2 ≥ 2.0, +3 ≥ 2.5) and protein probability (minimum 1.00E-3). The candidate protein queries were mapped to the UniProt Knowledge base (UniProtKB).Citation19)
Evaluation of biomass hydrolysis efficiency
The hydrolysis reactions (1 mL) contained 5% (w/v) alkaline pretreated biomass with the enzyme loading of 2–15 FPU/g-biomass (on dried weight basis) in 50 mM sodium acetate buffer, pH 5.0. The reactions were incubated at 40 °C for 48 h on a rotator mixer at 220 rpm. Activity complementation study was performed by supplementing 2 U/g-biomass of the recombinant β-xylosidase to the hydrolysis reactions. The experiments were done in triplicate. The amount of released reducing sugars was determined using the DNS method.Citation15) The sugar profiles were determined on a high-performance liquid chromatograph Dionex Ultimate-3000 (Thermo Fisher Scientific, Sunnyvale, CA, USA) equipped with a refractive index detector and a Shodex Sugar Column SC1011 (Showa Denko, Tokyo, Japan) with the column temperature of 65 °C using 5 mM H2SO4 as the mobile phase at a flow rate of 0.5 mL/min. Conversion efficiency was calculated as the percentage of glucose and xylose recovery from enzymatic hydrolysis based on the percent cellulose (×1.11) and xylan (×1.13), respectively in the pretreated biomass on a dried weight basis.
Statistical analysis
Data obtained from biomass hydrolysis experiments were analyzed using Analysis of Variance method followed by Tukey’s range test incorporated in the SPSS Statistics for Windows version 11.5 (SPSS Inc., Chicago, IL). Differences among means were considered to be significant at p < 0.05.
Results and discussion
Screening of cellulase producing wild-type strains
Capability of fungal isolates on production of four key enzymes, cellulase, xylanase, mannanase, and laccase were screened based on the activities in the crude culture supernatant. According to the screening (supplementary Table S2), S. commune BCC23363 was found as the most prominent strain for production of cellulase (highest CMCase) and among the top two strains for xylanase activities while it was the second most potent strain for mannanase activity. Laccase activity was not detected in most fungal strains under the screening condition, while low activity of laccase was observed in Mycena sp. and Phoma sp. and some unidentified fungi. However, they could produce relatively low cellulase, xylanase, and mannanase activities and therefore, not selected for further study. Based on these criteria, S. commune BCC23363 was finally selected for subsequent experiment.
Isolation of G-135 mutant strain
In order to further improve the enzyme producing capability of S. commune BCC23363 as an initial step toward development of a cellulase hyper producing strain, S. commune BCC23363 was used as parental strain for the isolation of mutants with increased cellulase activities. In total, 240 isolates were screened in Avicel-PH101 medium. Among them, G-135 showed the highest improvement on cellulase activity with > 2 folds increased of FPase, Avicelase, CMCase, and β-glucosidase compared to the parental strain according to quantitative analysis of the crude enzymes preparation. Comparison of polysaccharide-degrading enzyme activities between the mutant and parental strains is shown in Table . Activities of the enzymes produced by the mutant strain were not significantly changed after subcultivation of G-135 for 22 generations, indicating stability of the mutant strain on enzyme production.
Table 2. Comparison of cellulolytic and hemicellulolytic enzyme activities between the parental strain S. commune BCC23363 and mutant strain G-135 cultivated on Avicel-PH101 (n = 4).
Lignocellulolytic enzyme production by S. commune mutant
Effects of carbon sources on cellulolytic enzyme production of the S. commune mutant G-135 were studied. The fungus demonstrated robust growth on microcrystalline cellulose Avicel-PH101 and on all alkaline pretreated agricultural substrates as the sole carbon source. Degree of the composite cellulase and hemicellulase activities from the mutant strain was varied on the different carbon substrates (Tables and ). Avicel-PH101 was found to be a potential carbon source for inducing both cellulase and hemicellulase activities (Table ). High endo-xylanase activity was induced in all carbon sources with the highest volumetric activity of 1326 U/mL (equivalent to a specific activity of 1326 U/mg with the protein concentration of 1.0 mg/mL) when alkaline pretreated sugarcane bagasse was used as the carbon source (Table ). Lower polygalacturonase and amylase activities were obtained from the fungus grown on Avicel-PH101 compared to those found on the alkaline-pretreated biomass with alkaline pretreatment. A very low laccase activity was detected in the crude enzymes prepared on pretreated agricultural residues but not on Avicel-PH101 (the unit activity of laccase was expressed in nmole/mL), reflecting the significance of substrate composition on enhancing of specific enzyme activities toward hydrolysis of the respective biopolymers.
Table 3. Lignocellulose-degrading enzyme production by S. commune G-135 cultivated on different carbon sources (n = 4).
The composite cellulase (CMCase, FPase, and β-glucosidase) and hemicellulase (xylanase, mannanase, and β-xylosidase) activities in SC-Cel obtained from the medium containing Avicel-PH101 showed optimal activities at 50 °C under an acidic condition with the highest activity at pH 5.0–6.0 for most enzymes, except the CMCase and xylanase activities which exhibited the maximal activity under a more acidic pH range (3.0–4.0) (Figure ). The crude enzyme was stable at the temperature up to 40 °C with 100% residual activity retained for most enzymes after incubation for 16 h. However, more than 50% of FPase and other hydrolytic enzymes activities, including xylanase and β-glucosidase, were lost after treatment of the enzyme solution at 50 °C for 2 h in the absence of substrates.
Fig. 1. Effects of temperature (A) and pH (B) on composite enzyme activities produced by S. commune G-135.
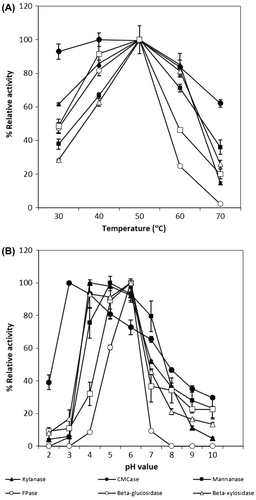
Fig. 2. Conversion efficiency of different pretreated agricultural biomass using SC-Cel and effects of downstream enzyme supplementation.
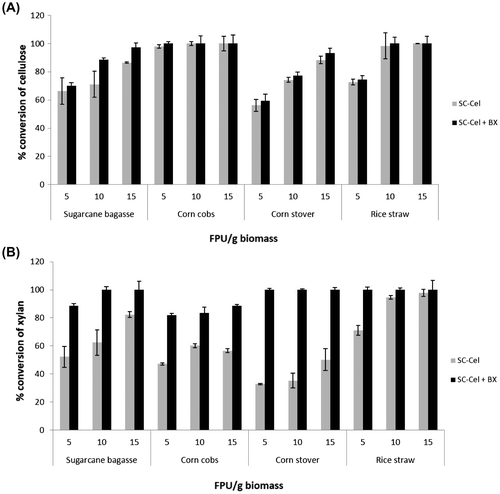
Proteomic analysis of SC-Cel
The secretome of S. commune G-135 cultivated on Avicel-PH101 was analyzed by LC/MS/MS for identification of the composite proteins. Proteomic analysis showed that SC-Cel comprised a complex mixture of hydrolytic and non-hydrolytic enzymes with different activities attacking different plant biopolymers. The annotated proteins were summarized according to their functions in Table . Various glycosyl hydrolases including a variety of cellulases, hemicellulases, and other non-starch polysaccharide degrading enzymes were found. A complete cellulase system comprising endo-glucanases (GH5, GH6, GH7, and GH45), cellobiohydrolases (GH6 and GH7), and β-glucosidases (GH3) were identified, consistent with the efficiency of SC-Cel on hydrolysis of cellulose to glucose. Endo-glucanases and exo-glucanases in these families were found common in the S. commune enzyme and those from fungal origins e.g. A. acculeatus, T. reesei.Citation10,20–22) A variety of hemicellulases was found including the main-chain attacking enzymes e.g. GH10, GH 11, and GH 5 as well as a number of non-hydrolytic debranching enzymes e.g. a carbohydrate esterase (CE15) and a polysaccharide lyase (PL1). In addition, auxiliary enzymes, for example, a copper-dependent lytic polysaccharide monooxygenases (AA9), cellobiose dehydrogenase (AA3), and carbohydrate-binding module family 13 protein (CBM13) were also annotated in the crude enzyme. The results thus suggested that the efficient hydrolysis of plant biomass by S. commune could be achieved by cooperative actions of various hydrolytic and non-hydrolytic composite enzymes with different mechanisms and specificities. It should be noted that a β-xylosidase was not found in the proteomic profile. However, a very low activity of this downstream xylan processing enzyme was detected in the crude SC-Cel from both the parental and mutant strains. This suggested limitation in the sensitivity of the proteomic analysis method.
Table 4. Summary of peptide identified in S. commune G-135 secretome.
The high complexity of the biomass-degrading enzyme system of S. commune has been revealed by the elucidated genome of S. commune strain H4-85, in which 240 candidates of glycoside hydrolases, 75 candidate glycosyltransferases, 16 candidate polysaccharide lyases, and 30 candidate carbohydrate esterases were identified in addition to a number of auxiliary enzymes attacking the lignin-carbohydrate complex.Citation5) According to the recent comparative secretome analysis, S. commune enzyme contained more diverse enzymes hydrolyzing non-cellulosic polysaccharides e.g. xylan and pectin as well as a larger set of enzymes generating hydroxyl radicals compared to other basidiomycetes, including Phanerochaete chrysosporium, Ceriporiopsis subvermispora, and Gloeophyllum trabeum. The crude enzyme derived from S. commune grown on Jerusalem artichoke stalk reported by Zhu et al. was shown to have higher performance on lignocellulose hydrolysis compared to the commercial cellulase from Trichoderma longbrachiatum based on the same enzyme dosage.Citation10) These works thus suggest potential of the S. commune cellulolytic enzyme for application in bioindustry, although supplementation an external β-xylosidase may be required in certain cases according to our study.
Synergism between conventional glycosyl hydrolases and auxiliary enzymes are the basis of efficient degradation of lignocelluloses. A lytic polysaccharide monooxygenase (AA9) and a glucoronoyl esterase annotated in the proteomic analysis in this study have been previously identified in S. commune.Citation10,24) An additional AA3 cellobiose dehydrogenase was also identified in our analysis but not in the previous report.Citation10) AA9 and AA3 have been shown to function as auxiliary components to the conventional glycosyl hydrolases on lignocellulose degradation. However, the significance of these enzymes on attacking the alkaline-pretreated biomass as used in our study still needs further investigation.
Evaluation of biomass hydrolysis efficiency
The efficiency of SC-Cel prepared from cultures grown on selected carbon sources was evaluated on enzymatic hydrolysis of the alkaline-pretreated agricultural biomass. In lignocellulosic materials, lignin and hemicelluloses exist as lignin-carbohydrate complexes (LCCs) mainly linked by ester or ether linkages.Citation25,26) According to Table , alkaline pretreatment led to decrease in the lignin and hemicellulose contents with respective increase in the cellulose fraction. Alkalis are believed to breakdown the hydrolysable linkages in LCCs leading to disruption of the lignin structure and leaching of the associated lignin.Citation27) Alkaline pretreatment under the conditions in this study can also cause partial hydrolysis of glycosidic bonds in polysaccharides with the loss of pectic substances.Citation28) These are accompanied with its effects on swelling of fibers, increasing accessible surface area of the substrates, and reducing in the degree of cellulose polymerization.Citation29) Altogether this led to improved enzymatic digestibility of the pretreated substrates.
The enzymatic hydrolysis reactions were performed at 40 °C, pH 5.0, resembling the conditions used in simultaneous saccharification and fermentation processes for bioethanol production with varying enzyme dosages (2, 4, and 6 FPU/g biomass). The maximal reducing sugar yield (885.2 ± 16.8 mg/g biomass) was achieved after incubation at 40 °C for 24 h using pretreated corn cobs as substrate (Table and Supplementary data Figure S1). Although AC appeared to give the highest reducing sugar yield on corn cob hydrolysis, there was no statistical difference found among the reducing sugar yields obtained from the hydrolysis of corn cobs using AC, BA, CC, CS, and RS. Furthermore, the statistical analysis data also showed no significant difference between the enzyme loading of 4 and 6 FPU/g biomass. The lower reducing sugar yields were obtained from hydrolysis of sugarcane bagasse, rice straw, and corn stover depending on the variation in enzymatic susceptibility of cellulosic substrates. According to the concept of substrate specificity, we expected the SC-Cel prepared from cultures grown on rice straw would be preferable for the hydrolysis of rice straw. However, the hydrolysis results showed that at the enzyme loading of 4 and 6 FPU/g biomass the SC-Cel produced on Avicel-PH101 was more effective for the hydrolysis of rice straw as indicated by the significant increased reducing sugar yields compared to other SC-Cel preparations (p < 0.05). Overall, SC-Cel prepared from cultures grown on Avicel-PH101 was more efficient for hydrolysis of various cellulosic substrates tested. Using Avicel-PH101 as the carbon source in the cultivation medium for enzyme production in bio-industry can also be advantageous from the point of the variation in compositions of the agricultural wastes. Statistical significance in three-way interactions between enzyme dosage, type of biomass and enzyme preparation together with two-way interactions between enzyme dosage/type of biomass, enzyme dosage/ enzyme preparation and type of biomass/enzyme preparation were also investigated (Table ). Considering the main effects (enzyme dosage, type of biomass, and enzyme preparation), all had significant effect on the reducing sugar yields (p < 0.05). Although, no significant interactions were observed under the hydrolysis condition, type of biomass/enzyme preparation tended to interact (p = 0.11) on the reducing sugar yields. In addition to the hydrolysis of alkaline-pretreated biomass, the efficiency of SC-Cel cultivated on Avicel-PH101 on the hydrolysis of native agricultural biomass was also tested. However, the amount of reducing sugar yields was very low (15.9–75.3 mg/g substrate) compared with those obtained from the alkaline pretreated biomass (Supplementary data Fig. S2). This could be due to the intact surface structure of the native lignocellulosic substrates and the shielding effect of lignin to the carbohydrate fractions as existed in the form of LCCs as well as the physicochemical stage of the cellulose fibers.Citation1)
Table 5. The reducing sugar release from the hydrolysis of various biomass at the enzyme dosage of 2, 4, and 6 FPU/g biomass (n = 3)
Complementation of SC-Cel with β-xylosidase
The efficiency of SC-Cel produced in the culture containing Avicel-PH101 was then evaluated with a wider enzyme dosage ranging from 5 to 15 FPU/g biomass under the hydrolysis condition at 40 °C for 48 h. The conversion of cellulose and xylan were calculated according to the available cellulose and xylan in the pretreated biomass. Variation in conversion efficiency depended on types of the pretreated biomass (Figure (A)). Increasing enzyme dosage led to higher glucose recovery for most substrates, except for corn cobs in which the maximal conversion yield (98.0%) was already achieved using the enzyme dosage of 5 FPU/g, suggesting high efficiency of the enzyme on cellulose hydrolysis. The highest recoveries of glucose of 100.0, 86.5, and 88.4% were obtained using the SC-Cel at an enzyme dosage of 15 FPU/g biomass, for rice straw, sugarcane bagasse, and corn stover, respectively. A marked increasing trend on xylose recovery was also found. However, relatively lower conversion efficiencies for xylan hydrolysis were observed for all pretreated biomass, particularly for corn cobs and corn stover at all enzyme dosages. The results seem to be related to the very low β-xylosidase activity in the crude SC-Cel (Table ).
Activity complementation of SC-Cel produced on Avicel-PH101 with recombinant β-xylosidase expressed in P. pastoris KM711Citation2) was studied at varying FPU dosages (Figure (A) and (B)). Supplementation of β-xylosidase into SC-Cel showed no significant improvement on the cellulose conversion yield of all biomass feedstock, except for bagasse in which 5.9–19.6% increase in glucose recovery was observed. However, supplementing β-xylosidase at 2 U/g biomass to SC-Cel led to 17.6–67.2% enhancement on xylose release for sugarcane bagasse, corn cobs, and corn stover under all cellulase dosages. The highest increase of xylose yield was observed on corn stover. Near completion of xylan conversion (88.5–100.0%) was obtained for all biomass feedstock using the enzyme dosage of 15 FPU/g.
Unlike the lignocellulolytic enzyme system of S. commune produced on Jerusalem artichoke stalk under solid state fermentation condition,Citation10) SC-Cel produced by submerged fermentation on Avicel-PH101 in this study contained a relatively low level of β-xylosidase. Complementation of SC-Cel with recombinant β-xylosidase was able to compensate an insufficient amount of β-xylosidase in the S. commune enzyme as shown by a remarkable improving in xylan conversion. Activity enhancement on xylan conversion by SC-Cel could reflect the lack of the downstream processing β-xylosidase activity in the enzyme preparation. Activity complementation by an external β-xylosidase could also lower the loading dosage of SC-Cel to achieve the maximum xylan conversion in the biomass hydrolysis step. Thus, addition of β-xylosidase to crude SC-Cel derived from other microorganisms or modification of the enzyme production media and fermentation conditions may be necessary in order to increase xylose conversion yield of biomass hydrolysis. Combined to the high cellulose conversion obtained by SC-Cel, the enhanced xylan conversion will provide additional xylose in the crude hydrolysate, which can be co-fermented with glucose to ethanol by some yeast strains e.g. Pichia stipitis and Candida shehate.30) Further strain improvement through targeted-genetic modification, e.g. expression of β-xylosidase as well as fermentation process optimization are essential in order to further increase the enzyme production level suitable for industrialization.
Conclusion
In conclusion, the lignocellulolytic enzyme system of S. commune mutant G-135 (SC-Cel) was shown to possess a complex activity profile attacking different parts of lignocellulosic materials with various core glycosyl hydrolases and auxiliary components in different families. Overall, SC-Cel produced from Avicel-PH101 effectively hydrolyzed all agricultural residues with the maximum glucan conversion up to 98.0% on corn cobs. The complementation of SC-Cel with an extra-recombinant β-xylosidase could compensate an insufficient amount of β-xylosidase as shown by a marked improvement in xylan conversion.
Supplemental materials
Supplemental material for this article can be accessed at https://doi.org/10.1080/09168451.2017.1320937.
Author contribution
WS and PR conducted the experimental works and prepared the first draft. SK and SR performed proteomic analysis. VC, TF, and HI designed the experiments, analyzed the results, and prepared the final manuscript for submission. LE provided comments and suggestion to the experiments.
Disclosure statement
No potential conflict of interest was reported by the authors.
Funding
This project was financially supported by the National Science and Technology Development Agency [grant number P-15-50502].
TBBB_1320937_Supplemental_Material.docx
Download MS Word (51.8 KB)Acknowledgments
WS was granted with a research fellowship from the New Energy Foundation (NEF).
References
- Fengel D, Wegener G. Wood: chemistry, ultrastructure, reactions. Berlin: De Gruyter; 1984.
- Inoue H, Decker SR, Taylor LE, et al. Identification and characterization of core cellulolytic enzymes from Talaromyces cellulolyticus (formerly Acremonium cellulolyticus) critical for hydrolysis of lignocellulosic biomass. Biotechnol Biofuels. 2014;7:151.10.1186/s13068-014-0151-5
- Dashtban M, Schraft H, Qin W. Fungal bioconversion of lignocellulosic residues; opportunities & perspectives. Int J Bio Sci. 2009;5:578–595.10.7150/ijbs.5.578
- Boa ER. Wild edible fungi: a global overview of their use and importance to people. Rome, Italy: Food and Agriculture Organization of the United Nations; 2004.
- Ohm RA, de Jong JF, Lugones LG, et al. Genome sequence of the model mushroom Schizophyllum commune. Nat Biotechnol. 2010;28:957–963.
- Jurasek L, Sopko R, Varadi J. Decomposition of beech wood holocellulose by supernatants of stationary cultures of wood destroying fungi. Ceska Mykol. 1968;22:43–49.
- Steiner W, Lafferty RM, Gomes I, et al. Studies on a wild strain of Schizophyllum commune: cellulase and xylanase production and formation of the extracellular polysaccharide Schizophyllan. Biotechnol Bioeng. 1987;30:169–178.10.1002/(ISSN)1097-0290
- Haltrich D, Preiss M, Steiner W. Optimization of a culture medium for increased xylanase production by a wild strain of Schizophyllum commune. Enzyme Microb Technol. 1993;15:854–860.10.1016/0141-0229(93)90097-L
- Horisawa S, Ando H, Ariga O, et al. Direct ethanol production from cellulosic materials by consolidated biological processing using the wood rot fungus Schizophyllum commune. Bioresour Technol. 2015;197:37–41.10.1016/j.biortech.2015.08.031
- Zhu N, Liu J, Yang J, et al. Comparative analysis of the secretomes of Schizophyllum commune and other wood-decay basidiomycetes during solid-state fermentation reveals its unique lignocellulose-degrading enzyme system. Biotechnol Biofuels. 2016;9:42.10.1186/s13068-016-0461-x
- Sluiter A, Hames B, Ruiz R, et al. Determination of structural carbohydrates and lignin in biomass. Golden, Colorado, USA: National Renewable Energy Laboratory; 2008.
- Wongwisansri S, Promdonkoy P, Matetaviparee P, et al. High-level production of thermotolerant β-xylosidase of Aspergillus sp. BCC125 in Pichia pastoris: characterization and its application in ethanol production. Bioresour Technol. 2013;132:410–413.10.1016/j.biortech.2012.11.117
- Fujii T, Iwata K, Murakami K, et al. Isolation of uracil auxotrophs of the fungus acremonium cellulolyticus and the development of a transformation system with the pyrF gene. Biosci Biotechnol Biochem. 2012;76:245–249.10.1271/bbb.110498
- Ghose TK. Measurement of cellulase activities. Pure Appl Chem. 1987;59:257–268.
- Miller G. Use of dinitrosalicylic acid reagent for detection of reducing sugar. Anal Chem. 1959;4:26–38.
- Ghose TK, Bisaria VS. Measurement of hemicellulase activities part 1: xylanase. Pure Appl Chem. 1987;59:1739–1752.
- Eggert C, Temp U, Eriksson KE. The ligninolytic system of the white rot fungus Pycnoporus cinnabarinus: purification and characterization of the laccase. Appl Environ Microbiol. 1996;62:1151–1158.
- Bradford MM. A rapid and sensitive method for the quantitation of microgram quantities of protein utilizing the principle of protein-dye binding. Anal Biochem. 1976;72:248–254.10.1016/0003-2697(76)90527-3
- Magrane M, UniProt Consortium. UniProt Knowledgebase: a hub of integrated protein data. Database, Vol. 2011, Article ID bar009. DOI:10.1093/database/bar009
- Suwannarangsee S, Bunterngsook B, Arnthong J, et al. Optimisation of synergistic biomass-degrading enzyme systems for efficient rice straw hydrolysis using an experimental mixture design. Bioresour Technol. 2012;119:252–261.10.1016/j.biortech.2012.05.098
- Zhao Z, Liu H, Wang C, et al. Comparative analysis of fungal genomes reveals different plant cell wall degrading capacity in fungi. BMC Genomics. 2013;14:274.10.1186/1471-2164-14-274
- Riley R, Salamov AA, Brown DW, et al. Extensive sampling of basidiomycete genomes demonstrates inadequacy of the white-rot/brown-rot paradigm for wood decay fungi. Proc Nat Acad Sci USA. 2014;111:9923–9923.
- Špániková S, Biely P. Glucuronoyl esterase – novel carbohydrate esterase produced by Schizophyllum commune. FEBS Lett. 2006;580:4597–4601.10.1016/j.febslet.2006.07.033
- Imamura T, Watanabe T, Kuwahara M, et al. Ester linkages between lignin and glucuronic acid in lignin-carbohydrate complexes from Fagus crenata. Phytochemistry. 1994;37:1165–1173.10.1016/S0031-9422(00)89551-5
- Scalbert A, Monties B, Lallemand JY, et al. Ether linkage between phenolic acids and lignin fractions from wheat straw. Phytochemistry. 1985;24:1359–1362.10.1016/S0031-9422(00)81133-4
- Sun S, Cheng JY. Hydrolysis of lignocellulosic materials for ethanol production: a review. Bioresour Technol. 2002;83:1–11.10.1016/S0960-8524(01)00212-7
- Sun R, Lawther JM, Banks WB. Influence of alkaline pre-treatments on the cell wall components of wheat straw. Ind Crops Prod. 1995;4:127–145.10.1016/0926-6690(95)00025-8
- Kumar P, Barrett DM, Delwiche MJ, et al. Methods for pretreatment of lignocellulosic biomass for efficient hydrolysis and biofuel production. Ind Eng Chem Res. 2009;48:3713–3729.10.1021/ie801542g
- du Preez JC, Bosch M, Prior BA. Xylose fermentation by Candida shehatae and Pichia stipitis: effects of pH, temperature and substrate concentration. Enzyme Microb. Technol. 1986;8:360–364.10.1016/0141-0229(86)90136-5