Abstract
We previously demonstrated that transgenic tobacco plants expressing cyanobacterial fructose-1,6-/sedoheptulose-1,7-bisphosphatase in the cytosol increased the number of lateral shoots and leaves at elevated CO2 levels. These findings suggest that alterations in carbon partitioning affect the development of shoot branching. In order to elucidate the underlying mechanisms at the molecular level, we generated transgenic Arabidopsis plants overexpressing cyanobacterial fructose-1,6-bisphosphatase-II in the cytosol (AcF). At elevated CO2 levels, the number of lateral shoots was significantly increased in AcF plants. Sucrose and hexose levels were also higher in AcF plants than in wild-type plants. The expression levels of MAX1, MAX4, YUCCA8, YUCCA9, and BRC1, which are involved in auxin or strigolactone biosynthesis and responses, were lower in AcF plants than in wild-type plants. These results suggest that alterations in sugar partitioning affect hormone metabolism and responses, resulting in enhanced shoot branching.
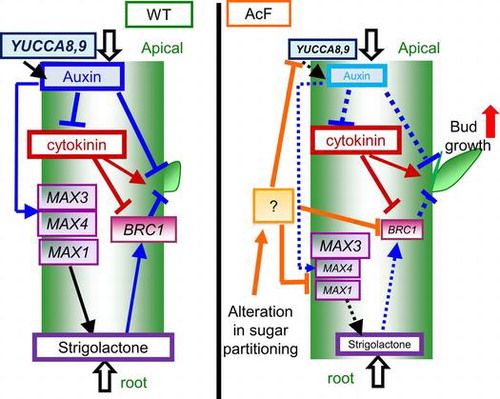
Plant morphogenesis is a key component of productivity and is an important target for crop breeding. Since the pattern of shoot branching may be reflected in sink strength, a clearer understanding of the regulatory mechanisms underlying shoot branching will contribute to an increase in crop yields. Plants adjust their branching capacity according to environmental conditions. Shoot branches develop from axillary meristems initiated in the boundary zone separating the leaf primordial from the shoot apex. Axillary meristems initially form axillary buds with a few leaves and the buds then stay dormant or develop into shoot branches.Citation1) Consequently, axillary bud initiation is a prerequisite for shoot branching.Citation2)
Shoot branching is influenced by a wide range of environmental signals, such as light and nutrient availability.Citation3,4) These environmental signals may be relayed through the effects of plant hormones. Auxin, cytokinins (CKs), and strigolactones (SLs) play important roles in controlling shoot branching. Indole-3-acetic acid (IAA), a bioactive form of the phytohormone auxin, is produced in young leaves at the shoot apexCitation5) and subsequently transported basipetally down the shoot in the polar auxin transport stream.Citation6) In the classical theory of apical dominance, auxin indirectly inhibits shoot branching [Citation7–9]. CKs represent a possible second messenger for auxin signaling in the regulation of bud initiation. In contrast to the indirect inhibitory effects of auxin, CKs directly promote bud initiation. The supply of CKs to buds promotes their outgrowthCitation10,11) and CK levels increase in buds as they activate.Citation12). Zhao et al.Citation13) reported that the apical stem cell niche is controlled by a mechanistic framework for auxin and CKs. On the other hand, SLs and their derivatives were identified as novel long-distance signal molecules controlling shoot branching in an analysis of high-branching mutants.Citation14–18) SLs inhibit bud outgrowth in an auxin independent manner.Citation19–22) Although auxin, CKs, and SLs coordinately control shoot branching, the mechanisms by which they control shoot branching for optimum morphogenesis at various growth stages have not yet been elucidated in detail.
We previously demonstrated that transgenic tobacco plants expressing cyanobacterial fructose-1,6-/sedoheptulose-1,7-bisphosphatase (FBP/SBPase) in the cytosol increased the number of lateral shoots and leaves at elevated CO2 levels.Citation23) On the other hand, enhancements of the Calvin cycle by introduction of FBP/SBPase into chloroplasts lead to elongation growth and longer transgenic plants with increases in sucrose and hexose contents, but no increase in lateral shoots.Citation24,25) These findings indicate that alterations in carbon partitioning in source and sink organs affect shoot branching development.
In the present study, we investigated the effects of alterations in sugar partitioning on the high-branching phenotype in Arabidopsis at the molecular level. We generated transgenic Arabidopsis plants overexpressing cyanobacterial fructose-1,6-bisphosphatase-II in the cytosol (AcF) and analyzed the expression of the genes involved in hormone metabolism in AcF plants. The results obtained revealed that alterations in carbon partitioning regulate the expression of genes involved in auxin and SL biosynthesis and responses. These results have provided a novel insight into the regulatory mechanisms underlying shoot branching via carbon partitioning at respective growth stages.
Materials and methods
Plant materials and growth conditions
Arabidopsis thaliana ecotype Columbia-0 (Col-0) plants were grown on 1:1 perlite/soil mix in three independent growth chambers under a 12-h light (23 °C) and 12-h dark (23 °C) cycle with a light intensity of 100–150 μmol photons m−2 s−1, 400 ppm (ambient) or 1000 ppm (elevated) CO2, and 60% relative humidity. The three growth chambers were, in turn, used for ambient and elevated CO2 conditions, and all data were then statistically analyzed.
Generation of transgenic plants expressing cyanobacterial FBPase-II in the cytosol
The genes encoding cyanobacterial FBPase-II isolated from Synechococcus elongatus PCC7942 (S. 7942) (FBPase-II: Accession number D49680)Citation26) were fused with the CaMV35S promoter in pDH121Citation27) (Fig. (A)). This construct was then introduced into wild-type Arabidopsis by Agrobacterium tumefaciens (C58)-mediated transformation utilizing the floral dip method.Citation28) Transgenic plants were selected on MS medium plates containing 0.8% (w/v) agar and 50 μg mL−1 hygromycin. Homozygous T3 generation plants harboring the transgene were used in subsequent experiments.
Fig. 1. Generation of transgenic Arabidopsis plants that expressed FBPase-II in the cytosol.
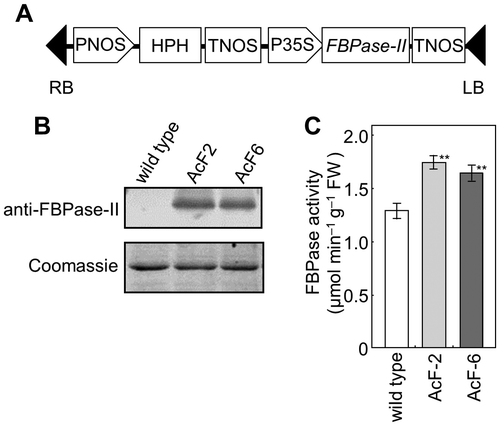
Preparation of a crude extract, enzyme assays, and immunoblotting
Leaf tissue (60–100 mg) was harvested at 6 h in the light phase and ground into a fine powder in liquid N2 using a pestle and mortar. The preparation and assays of enzymes involved in carbon metabolism were performed according to previously described methods.Citation24) Immunoblotting was performed as previously described.Citation24) Total soluble protein (15 μg) from wild-type and transgenic Arabidopsis plants was used for Western blotting with mouse antibodies raised against S. 7942 FBPase-II, as described.Citation26) Chlorophyll was measured by the method of Arnon.Citation29) Protein levels were measured by the method of BradfordCitation30) with bovine serum albumin as a standard.
Measurement of photosynthetic activity
CO2 fixation was measured as described previouslyCitation24) with the portable photosynthesis system Li-6400XT (Li-Cor, Lincoln, NE). Net CO2 assimilation rates were measured using rosette leaves under the following conditions: 0–1000 μmol photons m−2 s−1, 0–1200 μmol CO2 mol−1, 25 °C, and 60% relative humidity.
Measurement of metabolite and carbohydrate levels
Plant tissues (100 mg FW) were immediately placed into liquid N2, ground in liquid N2 using a pestle and mortar with 1 ml of 6% perchloric acid, and centrifuged at 15,000×g for 5 min. Pellets were used for starch measurements and supernatants were enzymatically examined for sugars (glucose, fructose, and sucrose) as described by Galtier et al.Citation31)
Quantitative real-time PCR experiments
Total RNA was extracted from the basal tissues of 5-week-old plants immediately after bolting. Quantitative Real-Time PCR (q-PCR) experiments were performed with the Roche LightCycler 96 system using FastStart Universal SYBR Green Master (ROX). Primer pairs for q-PCR were designed using PRIMER EXPRESS software (Applied Biosystems); primer sequences are shown in Supplemental Table S1. Actin2 was used as an internal standard in all experiments. At least three experimental replicates were used for one biological replicate.
Results
Generation of transgenic Arabidopsis expressing cyanobacterial FBPase-II in the cytosol
We generated transgenic Arabidopsis plants that expressed cyanobacterial FBPase-II in the cytosol and selected two T3-generation lines (AcF-2, AcF-6) displaying different FBPase-II overexpression patterns by Western blotting using an antibody raised against S. 7942 FBP/SBPase (Fig. (B)). The total activities of FBPase derived from endogenous FBPase (plastidic and cytosolic) and cyanobacterial FBPase-II were 1.35- and 1.27-fold higher in AcF-2 and AcF-6 plants, respectively, than in wild-type plants (Fig. (C)).
Six weeks after planting, the growth of AcF plants was indistinguishable from that of wild-type plants at ambient CO2 levels (Fig. (A)). No significant differences were observed in the dry weight of shoots, number of primary rosette branches, or number of lateral branches between wild-type and ApFS plants at 6 weeks at ambient CO2 levels (Fig. (B–D)).
Fig. 2. Phenotypes of wild-type and transgenic (AcF-2 and 6) plants grown with an ambient (400 ppm) CO2 level (A–D) and elevated (1000 ppm) CO2 level (E–H).
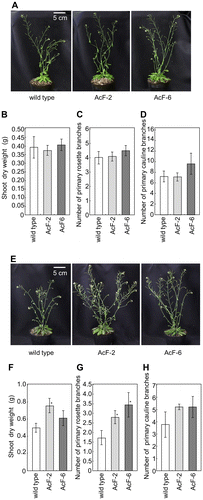
Phenotypes of AcF plants under ambient and elevated CO2 levels
The phenotypes of wild-type and AcF plants were compared under ambient and elevated CO2 levels after 6 weeks of planting. At elevated CO2 levels, rosette branches and cauline branches were thick, but the number of both branches decreased in both wild-type and AcF plants compared with those at ambient CO2 levels (Fig. ). Furthermore, at elevated CO2 levels, many secondary cauline branches were observed in AcF plants (Fig. (E)). In AcF plants, the number of primary rosette branches was approx. 1.6–2.0-fold larger than that of wild-type plants at elevated CO2 levels (Fig. (G)). Accordingly, AcF plants was bushy and the total dry weight of AcF-2 and 6 plants were approximately 1.3–1.5-fold larger than that of wild-type plants at elevated CO2 levels (Fig. (E–H)).
Photosynthetic activities in 5-week-old plants grown at elevated CO2 levels were measured under high CO2 (1400 ppm) and various light intensities (0–1000 μmol photons m−2 s−1). Furthermore, photosynthetic activities in 5-week-old plants grown at elevated CO2 levels were measured under saturated light intensities (500 μmol photons m−2 s−1) and various CO2 (0–1400 ppm) (Fig. ). At irradiance greater than 100 μmol photons m−2 s−1, photosynthetic activities were significantly stronger in AcF plants than in wild-type plants grown under high CO2 levels (Fig. (A)). At CO2 levels greater than 400 ppm, photosynthetic activities were significantly stronger in AcF plants than in wild-type plants grown under high CO2 levels (Fig. (B)).
Fig. 3. Photosynthetic parameters of wild-type and transgenic (AcF-2 and 6) plants grown with an elevated (1000 ppm) CO2 level for 5 weeks.
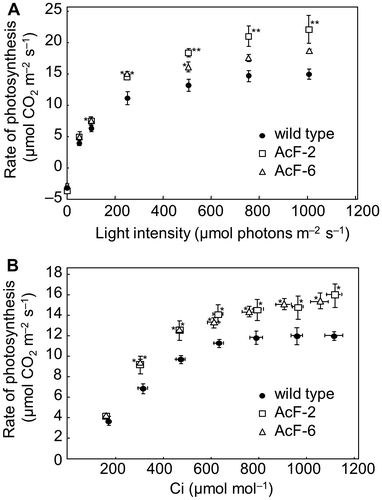
Sugar and starch levels in AcF plants at elevated CO2 levels
Hexose, sucrose, and starch levels were measured in the rosette leaves of 5-week-old plants at 6 h in the light phase (Fig. ). Under ambient CO2 levels, no significant differences were observed in the levels of sucrose, glucose, fructose, or starch between wild-type and AcF plants (data not shown). At elevated CO2 levels, starch levels were similar, whereas sucrose, glucose, and fructose levels were significantly higher in AsF-2 and AcF-6 plants than in wild-type plants. Accordingly, the sucrose/hexose/starch ratio in AcF plants differed from that in wild-type plants.
Fig. 4. Contents of starch (A), sucrose (B), glucose (C), and fructose (D) in 5-week-old leaves of wild-type and transgenic (AcF-2 and 6) plants grown with an elevated (1000 ppm) CO2 level.
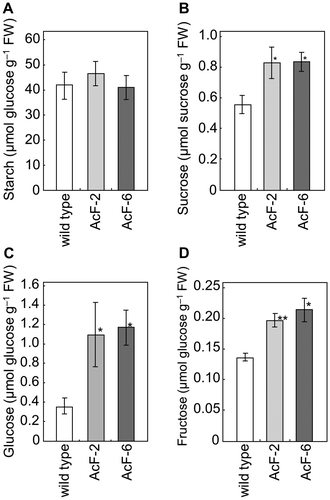
Transcript levels of genes involved in the biosynthesis of hormones and genes in response to hormones
In order to investigate whether plant hormones are involved in the branching phenotypes of AcF plants, we quantified the expression levels of genes involved in plant hormone biosynthesis and signaling in the rosette leaves of 5-week-old plants at 6 h in the light phase (Fig. ).
Fig. 5. Expression of genes involved in biosynthesis and signaling of auxin (A), CK (B) or SL (C) in 5-week-old leaves of wild-type and transgenic (AcF-2 and 6) plants grown with an elevated (1000 ppm) CO2 level.
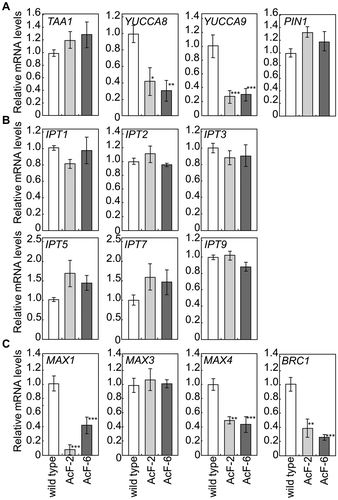
Auxin is synthesized from tryptophan through alternative pathways including the indole-3-pyruvic acid pathway. In these pathways, TRYPTOPHAN AMINOTRANSFERASE OF ARABIDOPSIS 1 (TAA1) and YUCCA flavin monooxygenase-like proteins play an important role in auxin biosynthesis.Citation32–34) Moreover, PIN-FORMED efflux carrier proteins (PINs) and AUX are involved in intercellular auxin transport.Citation35,36) In order to confirm whether auxin is related to the branching phenotypes of AcF plants, the expression levels of TAA1, YUCCA8, YUCCA9, and PIN1 were compared between wild-type and AcF plants. The transcript levels of YUCCA8 and YUCCA9 were markedly lower in ApFS plants than in wild-type plants (Fig. (A)). On the other hand, no significant differences were observed in the transcript levels of TAA1 or PIN1 between wild-type and ApFS plants (Fig. (A)).
In the CK biosynthesis pathway, the enzymatic reaction catalyzed by the ISOPENTENYL TRANSFERASE (IPT1, 2, 3, 5, 7, 9) family is the rate-limiting step.Citation37,38) In order to confirm whether CKs are related to the branching phenotypes of AcF plants, the expression levels of IPT1, IPT2, IPT3, IPT5, IPT7, and IPT9 were compared between wild-type and AcF plants. No significant differences were observed in the transcript levels of these genes between wild-type and AcFS plants (Fig. (B)).
SLs are produced from carotenoids catalyzed by CAROTENOID CLEAVAGE OXYGENASE (CCD7/MAX3 and CCD8/MAX4) and P450 oxygenase (MAX1) in Arabidopsis.Citation39–41) Moreover, Aguilar-Martínez et al.Citation42) reported that BRANCHED1 (BRC1) acts as an integrator of the branching signals controlling bud outgrowth. In order to confirm whether SLs are related to the branching phenotypes of AcF plants, the expression levels of MAX1, MAX3, MAX4, and BRC1 were compared between wild-type and AcF plants. The transcript levels of MAX1, MAX4, and BRC1 were lower in AcFS plants than in wild-type plants (Fig. (C)). On the other hand, no significant differences were observed in the transcript levels of MAX3 between wild-type and AcFS plants (Fig. (C)).
Discussion
We previously reported that transgenic tobacco plants with an enhanced sucrose biosynthesis capacity showed increased numbers of lateral shoots and leaves at elevated CO2 levels.Citation23) This phenotype suggests that alterations in carbon partitioning control branching. In the present study, in order to investigate the effects of alterations in sugar partitioning on shoot branching at the molecular level, we generated transgenic Arabidopsis plants expressing cyanobacterial FBPase-II in the cytosol. When AcF plants were grown at ambient CO2 levels, the increase observed in FBPase activity in the cytosol did not affect growth, shoot dry weight, or the number of branches (Fig. (A–D)). In transgenic potato plants, a 45% reduction in cytosolic FBPase activity did not cause any change in growth, yield, or photosynthetic activity at ambient CO2,Citation43) suggesting that cytosolic FBPase was not a rate-limiting enzyme for sucrose biosynthesis at ambient CO2 levels. On the other hand, at elevated CO2 levels, the number of primary rosette branches was significantly increased in AcF plants, resulting in an increase in shoot dry weight (Fig. (E–H)). Moreover, the photosynthetic CO2 assimilation rate was higher in AcF plants than in wild-type plants (Fig. ). Plants grown at elevated CO2 levels show higher photosynthetic activity than those grown at ambient CO2 levels. Enhanced photosynthetic activity at elevated CO2 levels commonly leads to increased photosynthetic intermediates including triose phosphates which were transported from chloroplasts to the cytosol in exchange for Pi. When the cytosolic Pi concentration is low, triose phosphate is retained within the chloroplasts, and causes down regulation of photosynthesis.Citation44) Our findings suggest that enhanced FBPase activity contributes to the efficient conversion of increased fructose 1,6-bisphosphate to fructose 6-phosphate and Pi in the cytosol, resulting in a reduction in the limitation of photosynthesis imposed by accumulation of triose phosphate and depletion of Pi in chloroplasts. These phenotypes were similar to those of transgenic tobacco plants expressing FBP/SBPase in the cytosol. Sucrose, glucose, and fructose levels were higher in AcF plants than in wild-type plants at elevated CO2 levels (Fig. ). This increase may be dependent on an increased capacity for sucrose biosynthesis and photosynthetic CO2 assimilation. However, in the source leaves of transgenic tobacco plants expressing FBP/SBPase in the cytosol, the significant increases detected in hexose levels in wild-type tobacco plants were markedly reduced.Citation23) We speculate that the effects of an enhanced sucrose biosynthesis capacity on carbon partitioning depend on the plant architecture of source and sink organs between tobacco and Arabidopsis. These results suggest that an enhanced sucrose biosynthesis capacity leads to alterations in carbon partitioning in plants and that these alterations may affect the regulatory mechanisms underlying shoot branching.
Since shoot branching is coordinately controlled by plant hormones, we expected alterations in carbon partitioning to affect the metabolism of plant hormones in AcF plants. It is preferable to determine the contents of plant hormones to obtain direct evidence for branching phenotype of AcF plants. However, auxin and SLs are present in minute amounts in confined tissues, and these amounts may decrease in AcF plants. Accordingly, it is difficult for us to determine the amount of these plant hormones. Therefore, the transcript levels of genes involved in plant hormone biosynthesis and responses were analyzed using basal tissues of AcF and wild-type plants just after bolting (Fig. ). The transcript levels of YUCCA8 and YUCCA9, which are involved in auxin biosynthesis, were lower in AcF plants than in wild-type plants. Furthermore, the transcript levels of MAX1 and MAX4, which are involved in SL biosynthesis, were lower in AcF plants than in wild-type plants. Hentrich et al.Citation32) reported that yucca8/yucca9 double knockout mutant showed 50% of endogenous IAA content compared with those in wild-type plants. Abe et al.Citation45) reported that endogenous contents of carlactone, a precursor of SLs in Arabidopsis, were below the detection limit in max1 and max4 mutants. Furthermore, the transcript levels of BRC1, a key transcriptional regulator responsible for maintaining bud dormancy modulated by SLs,Citation42,46) were lower in AcF plants than in wild-type plants. These findings support our speculation that the contents of auxin and SLs may be lower in AcF plants than in wild-type plants.
Sairanen et al.Citation47) previously reported that an external glucose treatment induced the expression of auxin biosynthesis genes and contents of IAA metabolites in Arabidopsis seedlings. Furthermore, Barbier et al.Citation48) showed that a sucrose treatment induced bud elongation in Rosa hybrida. In the buds of Rosa hybrida, the sucrose treatment upregulated early auxin biosynthesis genes (RhTAR1 and RhYUC1) and an auxin efflux carrier gene (RhPIN1), and downregulated RwMAX2, which is involved in the SL transduction pathway, and RhBRC1, a repressor of branching, at the early bud outgrowth stage. These findings support our hypothesis that changes in sugar partitioning regulate the transcript levels of genes involved in auxin and SL biosynthesis in AcF plants. However, in contrast to previous findings, SL and auxin levels were lower in AcF plants than in wild-type plants. According to the classic model, auxin produced in the shoot tip is transported down the stem and inhibits bud outgrowth. Li and BangerthCitation49) reported that polarly transported IAA is responsible for the inhibition of lateral buds. On the other hand, Brewer et al.Citation22,50) found that SLs act downstream of auxin to regulate bud outgrowth in pea and Arabidopsis. Furthermore, Mason et al.Citation51) showed that sugars, not auxin, are important regulators of axillary bud release from the apical dominance in decapitated plants. Therefore, increases in auxin levels in seedlings and buds with an artificial supply of sugar may not be related to bud outgrowth, and repress the transcript levels of BRC1 in order to regulate branching.
Our results support a model in which sugars induce bud outgrowth with alterations in the expression of genes involved in hormone metabolism. However, the mechanisms by which sugars regulate shoot branching via the transcriptional regulation of these genes currently remain unclear. Hexokinase 1-overexpressing Arabidopsis showed enhanced shoot.Citation52) PIF (phytochrome-interacting factor) proteins are involved in the regulation of auxin homeostasis, and sucrose may regulate the expression of PIF genes.Citation53,54) Although mitogen-activated protein kinase (MAPK/MPK) signaling pathways are responsible for various physiological responses, the MAP kinase kinase 7 (MKK7)-MPK6 cascade controls shoot branching by the phosphorylation state of the PIN1 protein.Citation55) Liu et al.Citation56 reported that SL biosynthesis was regulated by the GRAS-type transcription factors NODULATION SIGNALING PATHWAY1 (NSP1) and NSP2. If these factors are regulated by alterations in sugar partitioning, the mechanisms by which sugars regulate shoot branching via the transcriptional regulation of genes involved in SL and auxin biosynthesis may become clearer.
Supplemental materials
The supplemental material for this paper is available at https://doi.org/10.1080/09168451.2017.1321954.
Author contributions
M.T. and S.S. conceived and designed the research. K.O. and N.T. performed the experiments and analyzed the data. M.T. wrote the manuscript with assistance of S.S. All authors read and discussed the manuscript.
Funding
This work was supported by JST CREST, Japan [grant number JPMJCR12B3].
Disclosure statement
No potential conflict of interest was reported by the authors.
TBBB_1321954_Supplemental_TableS1.docx
Download MS Word (32.3 KB)Notes
Abbreviations: CK, cytokinin; FBP, fructose 1,6-bisphosphate; FBPase, fructose-1,6-bisphosphatase; FBP/SBPase, fructose-1,6-/sedoheptulose-1,7-bisphosphatase; IAA, indole-3-acetic acid; SL, strigolactone
References
- McSteen P, Leyser O. Shoot branching. Annu Rev Plant Biol. 2005;56:353–374.10.1146/annurev.arplant.56.032604.144122
- Guo D, Zhang J, Wang X, et al. The WRKY transcription factor WRKY71/EXB1 controls shoot branching by transcriptionally regulating RAX genes in arabidopsis. Plant Cell. 2015;27:3112–3127.10.1105/tpc.15.00829
- Snowden KC, Napoli CA. A quantitative study of lateral branching in petunia. Funct Plant Biol. 2003;30:987–994.10.1071/FP03081
- Cline MG. Apical dominance. Bot Rev. 1991;57:318–358.10.1007/BF02858771
- Ljung K, Bhalerao RP, Sandberg G. Sites and homeostatic control of auxin biosynthesis in Arabidopsis during vegetative growth. Plant J. 2001;28:465–474.
- Blakeslee JJ, Peer WA, Murphy AS. Auxin transport. Curr Opin Plant Biol. 2005;8:494–500.10.1016/j.pbi.2005.07.014
- Snow R. The young leaf as the inhibiting organ. New Phytol. 1929;28:345–358.10.1111/nph.1929.28.issue-5
- Cline MG. Exogenous auxin effects on lateral bud outgrowth in decapitated shoots. Ann Bot. 1996;78:255–266.10.1006/anbo.1996.0119
- Thimann KV, Skoog F. Studies on the growth hormone of plants. III. The inhibiting action of the growth substance on bud development. Proc Nat Acad Sci USA. 1993;19:714–716.
- Sachs T, Thimann kV. The role of auxins and cytokinin in the release of buds from dominance. Am J Bot. 1967;54:136–144.10.2307/2440896
- Miguel LC, Longnecker NE, Ma Q, et al. Branch development in Lupinus angustifolius L.I. Not all branches have the same potential growth rate. J Exp Bot. 1998;49:547–553.
- Emery RJ. cis-Isomers of cytokinins predominate in chickpea seeds throughout their development. Plant Physiol. 1998;117:1515–1523.10.1104/pp.117.4.1515
- Zhao Z, Andersen SU, Ljung K, et al. Hormonal control of the shoot stem-cell niche. Nature. 2010;465:1089–1092.10.1038/nature09126
- Umehara M, Hanada A, Yoshida S, et al. Inhibition of shoot branching by new terpenoid plant hormones. Nature. 2008;455:195–200.10.1038/nature07272
- Ongaro V, Leyser O. Hormonal control of shoot branching. J Exp Bot. 2007;59:67–74.10.1093/jxb/erm134
- Ferguson BJ, Beveridge CA. Roles for auxin, cytokinin, and strigolactone in regulating shoot branching. Plant Physiol. 2009;149:1929–1944.10.1104/pp.109.135475
- McSteen P. Hormonal regulation of branching in grasses. Plant Physiol. 2009;149:46–55.10.1104/pp.108.129056
- Beveridge CA, Kyozuka J. New genes in the strigolactone-related shoot branching pathway. Curr Opin Plant Biol. 2010;13:34–39.10.1016/j.pbi.2009.10.003
- Beveridge CA, Symons GM, Turnbull CGN. Auxin inhibition of decapitation-induced branching is dependent on graft-transmissible signals regulated by genes Rms1 and Rms2. Plant Physiol. 2000;123:689–698.10.1104/pp.123.2.689
- Beveridge CA, Dun EA, Rameau C. Pea has its tendrils in branching discoveries spanning a century from auxin to strigolactones. Plant Physiol. 2009;151:985–990.10.1104/pp.109.143909
- Beveridge CA. Axillary bud outgrowth: sending a message. Curr Opin Plant Biol. 2006;9:35–40.10.1016/j.pbi.2005.11.006
- Brewer PB, Dun EA, Gui R, et al. Strigolactone inhibition of branching independent of polar auxin transport. Plant Physiol. 2015;168:1820–1829.10.1104/pp.15.00014
- Tamoi M, Hiramatsu Y, Nedachi S, et al. Increase in the activity of fructose-1,6-bisphosphatase in cytosol affects sugar partitioning and increases the lateral shoots in tobacco plants at elevated CO2 levels. Photosynth Res. 2011;108:15–23.10.1007/s11120-011-9645-1
- Miyagawa Y, Tamoi M, Shigeoka S. Overexpression of a cyanobacterial fructose-1,6-/sedoheptulose-1,7-bisphosphatase in tobacco enhances photosynthesis and growth. Nature Biotechnol. 2001;19:965–969.10.1038/nbt1001-965
- Yabuta Y, Tamoi M, Yamamoto K, et al. Molecular design of photosynthesis-elevated chloroplasts for mass accumulation of a foreign protein. Plant Cell Physiol. 2008;49:375–385.10.1093/pcp/pcn014
- Tamoi M, Ishikawa T, Takeda T, et al. Molecular characterization and resistance to hydrogen peroxide of two fructose-1,6-bisphosphatases from Synechococcus PCC 7942. Arch Biochem Biophys. 1996;334:27–36.10.1006/abbi.1996.0425
- Daimon Y, Takabe K, Tasaka M. The CUP-SHAPED COTYLEDON genes promote adventitious shoot formation on calli. Plant Cell Physiol. 2003;44:113–121.10.1093/pcp/pcg038
- Clough SJ, Bent AF. Floral dip: a simplified method for Agrobacterium-mediated transformation of Arabidopsis thaliana. Plant J. 1998;16:735–743.10.1046/j.1365-313x.1998.00343.x
- Arnon DI. Copper enzymes in isolated chloroplasts. polyphenoloxidase in Beta vulgaris. Plant Physiol. 1949;24:1–15.10.1104/pp.24.1.1
- Bradford MM. A rapid and sensitive method for the quantitation of microgram quantities of protein utilizing the principle of protein-dye binding. Anal Biochem. 1976;72:248–254.10.1016/0003-2697(76)90527-3
- Galtier N, Foyer C, Murchie1 E, et al. Effects of light and atmospheric carbon dioxide enrichment on photosynthesis and carbon partitioning in the leaves of tomato (Lycopersicon esculentum L.) plants over-expressing sucrose phosphate synthase. J Exp Bot. 1995;46:1335–1344.10.1093/jxb/46.special_issue.1335
- Hentrich M, Böttcher C, Düchting P, et al. The jasmonic acid signaling pathway is linked to auxin homeostasis through the modulation of YUCCA8 and YUCCA9 gene expression. Plant J. 2013;74:626–637.10.1111/tpj.2013.74.issue-4
- Mashiguchi K, Tanaka K, Sakai T, et al. The main auxin biosynthesis pathway in Arabidopsis. Proc Nat Acad Sci USA. 2011;108:18512–18517.10.1073/pnas.1108434108
- Tao Y, Ferrer JL, Ljung K, et al. Rapid synthesis of auxin via a new tryptophan-dependent pathway is required for shade avoidance in plants. Cell. 2008;133:164–176.10.1016/j.cell.2008.01.049
- Teale WD, Paponov IA, Palme K. Auxin in action: signalling, transport and the control of plant growth and development. Nat Rev Mol Cell Biol. 2006;7:847–859.10.1038/nrm2020
- Habets MEJ, Offringa R. PIN-driven polar auxin transport in plant developmental plasticity: a key target for environmental and endogenous signals. New Phytol. 2014;203:362–377.10.1111/nph.12831
- Sakakibara H, Takei K, Hirose N. Interactions between nitrogen and cytokinin in the regulation of metabolism and development. Trends Plant Sci. 2006;11:440–448.10.1016/j.tplants.2006.07.004
- Miyawaki K, Matsumoto-Kitano M, Kakimoto T. Expression of cytokinin biosynthetic isopentenyltransferase genes in Arabidopsis: tissue specificity and regulation by auxin, cytokinin, and nitrate. Plant J. 2004;37:128–138.10.1046/j.1365-313X.2003.01945.x
- Al-Babili S, Bouwmeester HJ. Strigolactones, a novel carotenoid-derived plant hormone. Annu Rev Plant Biol. 2015;66:161–186.10.1146/annurev-arplant-043014-114759
- Booker J, Auldridge M, Wills S, et al. MAX3/CCD7 is a carotenoid cleavage dioxygenase required for the synthesis of a novel plant signaling molecule. Curr Biol. 2004;14:1232–1238.10.1016/j.cub.2004.06.061
- Zhang Y, van Dijk AD, Scaffidi A, et al. Rice cytochrome P450 MAX1 homologs catalyze distinct steps in strigolactone biosynthesis. Nat Chem Biol. 2014;10:1028–1033.10.1038/nchembio.1660
- Aguilar-Martínez JA, Poza-Carrión C, Cubas P. Arabidopsis BRANCHED1 acts as an integrator of branching signals within axillary buds. Plant Cell. 2007;19:458–472.10.1105/tpc.106.048934
- Zrenner R, Krause KP, Apel P, et al. Reduction of the cytosolic fructose-1,6-bisphosphatase in transgenic potato plants limits photosynthetic sucrose biosynthesis with no impact on plant growth and tuber yield. Plant J. 1996;9:671–681.10.1046/j.1365-313X.1996.9050671.x
- Sharkey TD. Photosynthesis in intact leaves of C3 plants: physics, physiology and rate limitations. Bot Rev. 1985;51:53–105.10.1007/BF02861058
- Abe S, Sado A, Tanaka K, et al. Carlactone is converted to carlactonoic acid by MAX1 in Arabidopsis and its methyl ester can directly interact with AtD14 in vitro. Proc Nat Acad Sci USA. 2014;111:18084–18089.10.1073/pnas.1410801111
- Braun N, de Saint Germain A, Pillot JP, et al. The pea TCP transcription factor PsBRC1 acts downstream of strigolactones to control shoot branching. Plant Physiol. 2012;158:225–238.10.1104/pp.111.182725
- Sairanen I, Novak O, Pencik A, et al. Soluble carbohydrates regulate auxin biosynthesis via pif proteins in arabidopsis. Plant Cell. 2012;24:4907–4916.10.1105/tpc.112.104794
- Barbier F, Peron T, Lecerf M, et al. Sucrose is an early modulator of the key hormonal mechanisms controlling bud outgrowth in Rosa hybrida. J Exp Bot. 2015;66:2569–2582.10.1093/jxb/erv047
- Li CJ, Bangerth F. Autoinhibition of indoleacetic acid transport in the shoots of two-branched pea (Pisum sativum) plants and its relationship to correlative dominance. Physiol Plant. 1999;106:415–420.10.1034/j.1399-3054.1999.106409.x
- Brewer PB, Dun EA, Ferguson BJ, et al. Strigolactone acts downstream of auxin to regulate bud outgrowth in pea and arabidopsis. Plant Physiol. 2009;150:482–493.10.1104/pp.108.134783
- Mason MG, Ross JJ, Babst BA, et al. Sugar demand, not auxin, is the initial regulator of apical dominance. Proc Nat Acad Sci USA. 2014;111:6092–6097.10.1073/pnas.1322045111
- Kelly G, David-Schwartz R, Sade N, et al. The pitfalls of transgenic selection and new roles of AtHXK1: a high level of AtHXK1 expression uncouples hexokinase1-dependent sugar signaling from exogenous sugar. Am Soc Plant Biol. 2012;159:47–51.
- Franklin KA, Lee SH, Patel D, et al. Phytochrome-interacting factor 4 (PIF4) regulates auxin biosynthesis at high temperature. Proc Nat Acad Sci USA. 2011;108:20231–20235.10.1073/pnas.1110682108
- Stewart JL, Maloof JN, Nemhauser JL. PIF genes mediate the effect of sucrose on seedling growth dynamics. PLoS ONE. 2011;6:e19894.10.1371/journal.pone.0019894
- Jia W, Li B, Li S, et al. Mitogen-activated protein kinase cascade MKK7-MPK6 plays important roles in plant development and regulates shoot branching by phosphorylating PIN1 in arabidopsis. PLoS Biol. 2016;14:e1002550.10.1371/journal.pbio.1002550
- Liu W, Kohlen W, Lillo A, et al. Strigolactone biosynthesis in medicago truncatula and rice requires the symbiotic GRAS-type transcription factors NSP1 and NSP2. Plant Cell. 2011;23:3853–3865.10.1105/tpc.111.089771