Abstract
In response to environmental stressors such as blast fungal infections, rice produces phytoalexins, an antimicrobial diterpenoid compound. Together with momilactones, phytocassanes are among the major diterpenoid phytoalexins. The biosynthetic genes of diterpenoid phytoalexin are organized on the chromosome in functional gene clusters, comprising diterpene cyclase, dehydrogenase, and cytochrome P450 monooxygenase genes. Their functions have been studied extensively using in vitro enzyme assay systems. Specifically, P450 genes (CYP71Z6, Z7; CYP76M5, M6, M7, M8) on rice chromosome 2 have multifunctional activities associated with ent-copalyl diphosphate-related diterpene hydrocarbons, but the in planta contribution of these genes to diterpenoid phytoalexin production remains unknown. Here, we characterized cyp71z7 T-DNA mutant and CYP76M7/M8 RNAi lines to find that potential phytoalexin intermediates accumulated in these P450-suppressed rice plants. The results suggested that in planta, CYP71Z7 is responsible for C2-hydroxylation of phytocassanes and that CYP76M7/M8 is involved in C11α-hydroxylation of 3-hydroxy-cassadiene. Based on these results, we proposed potential routes of phytocassane biosynthesis in planta.
Graphical abstract
C2-hydoroxylation and C11-hydorxylation are active in planta catalyzed by CYP71Z7 and CYP76M7/M8 leading to phytocassanes. New intermediates are found in this study.
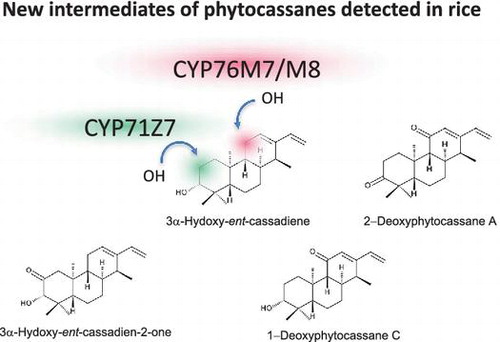
Phytoalexins are a group of low-molecular-weight antimicrobial compounds in plants that are induced under biotic and abiotic stresses, including blast fungus infection, insect herbivory, and UV irradiation [Citation1]. Notably, genes encoding phytoalexin synthesis in rice comprise a gene cluster on chromosomes. The existence of gene cluster formation for specialized metabolites has been demonstrated in several plant species: the avenacin cluster in oats [Citation2], the benzoxazinone cluster in maize [Citation3], the thalianol and marneral clusters in Arabidopsis [Citation4,5], potential clusters in cucumber [Citation6], as well as a new momilactone (major diterpenoid phytoalexin class) cluster in paddy weeds barnyard grass Echinochloa crus-galli [Citation7].
We previously showed that in rice, momilactone biosynthetic genes are clustered on chromosome 4 and comprise two diterpene cyclases (OsCSP4 and OsKSL4), two cytochrome P450 monooxygenases (P450) (CYP99A2 and A3), as well as two dehydrogenease (OsMAS1 and 2) [Citation8,9]. The other major diterpenoid phytoalexin in rice is the phytocassanes, and their biosynthetic genes are also thought to exist as a cluster – two for diterpenoid cyclases (OsCPS2 and OsKSL7) and six for P450s (CYP71Z6 and Z7, CYP76M5 to M8) – on rice chromosome 2.
The biological function of these P450s has been studied extensively using in vitro biochemical approaches. Both CYP76M7 and M8 catalyze the 11α-hydroxylation of ent-cassa-12,15-diene, a precursor to phytocassane [Citation10]. CYP71Z7 is also involved in phytocassane production, hydroxylating the phytocassane framework at position C2 to produce 2α-hydroxy-ent-cassadiene; this is then likely followed by the production of a 2α-hydroxy group of phytocassanes, phytocassanes A and B [Citation11]. These studies suggest a plausible functional cluster for phytocassanes in chromosome 2, similar to the momilactone biosynthetic gene cluster in chromosome 4.
P450 gene clusters may also be present in chromosomes besides chromosome 2. For instance, CYP701A8, which is clustered with other P450 genes of CYP701A family on chromosome 6, exhibits biochemical activity related to C3α-hydroxylation of several ent-copalyl diphosphate-derived diterpene olefins, including ent-cassadiene. Such characteristics are consistent with a role in phytocassane biosynthesis [Citation12].
Despite these data, we still lack sufficient information regarding in planta involvement of the relevant P450 genes and their possible substrates and hydroxylated products to fully depict the entire phytocassane biosynthesis pathway. In this study, we characterized loss-of-function plants defective in each P450 gene to demonstrate that both are involved in phytocassane production in planta. We also found possible phytocassane precursors (1-deoxyphytocassane C and 2-deoxyphytocassane A) in the cyp71z7 mutant. Additionally, 3-hydroxy-cassadiene and its ketone derivative at position C2 were also detected in CYP76M7/M8 knock-down plants. Based on these results, we proposed an in planta biosynthetic pathway of phytocassanes based on our results showing that CYP71Z7 and CYP76M7/M8 are part of the functional phytocassane biosynthesis genes in the cluster on chromosome 2.
Material and methods
Plant materials
Rice O. sativa L. “Nipponbare” and “Dongjin” were used as the plant materials in this study. To grow the rice plants, the seeds were sterilized with 70% ethanol for 2 min, followed by treatment with 1% sodium hypochlorite solution (Kanto Chemical) for 20 min; the seeds were then washed with autoclave-sterilized water. The surface-sterilized seeds were incubated on 0.5% agar medium in a chamber (14-h light and 10-h darkness at 28 °C). The leaf blades of 10-days-old rice seedlings (for metabolites analysis) or 1-month-old adult plants (for RNA preparation) were cut off and treated with CuCl2 as described previously [Citation13]. The treated leaf blades were collected and frozen in liquid N2 used for both cDNA preparation and phytoalexin measurement as described hereinbelow. Calli of O. sativa L. “Nipponbare” were cultured as described previously [Citation14]. The treatment with a chitin oligosaccharide elicitor or cholic acid was also performed as previously described [Citation15,16]. The cyp71z7 mutant (PFG_3A-00433, Oryza sativa L. “Dongjin”) was searched for in the Rice Functional Genomic Express Database as a T-DNA insertion mutant of Os02g0570700 (TIGR ID, LOC_Os02g36190) and purchased from Postech Biotech Center. The cyp71z7 homozygous mutant was selected by genomic PCR using following specific primers for CYP71Z7 and T-DNA to separate cyp71z7 homozygotes, heterozygotes, and wild-type rice plants; CYP71Z7-ORF-F, 5′-AGGAGACCCCCGGAATAGTT-3′ and CYP71Z7-3′UTR-R, 5′-TTGTTTTTATGGAAGTGGTAACAA-3 for native CYP71Z7 or T-DNA Seq2 RB outer R, 5′-TTGGGGTTTCTACAGGACGTAAC-3 for T-DNA fragment. The CYP76M7/8 RNAi plants used were generated previously as described in Wang et al. [Citation10]. Three independent transgenic lines were redifferentiated from transgenic calli and confirmed the suppression of CYP76M7/M8 mRNA by qRT-PCR using the primer set as described previously [Citation10].
Expression analysis
Total RNA was extracted from each elicitor-treated leaf-blade sample using Sepasol(R)-RNA Ⅰ Super G (Nacalai Tesque). cDNA was synthesized using PrimeScript™ RT reagent Kit with gDNA Eraser (Perfect Real Time) (TaKaRa BioInc., Japan). qRT-PCR was performed using the Power SYBR® Green PCR Master Mix (Applied Biosystems, CA, USA) and Applied Biosystems 7300 Real-Time PCR System (Applied Biosystems) as described previously [Citation13]. Primer set was designed based on the genome sequences of each targeted gene: CYP71Z6-F, 5′-GTTTATTGAAAAAATAACGTGCTGG-3′ and CYP71Z6-R, 5′-AGTGGTAGCATTACACACTGGAA-3′ for CYP71Z6; CYP71Z7-F, 5′-CCCTATGTATTCTCAAGTTGCTTTG-3′ and CYP71Z7-R, 5′-TTGTTTTTATGGAAGTGGTAACAA-3′ for CYP71Z7; OsCPS2-F, 5′-TTAGGAAAATGGTTGACTAC-3′ and OsCPS2-R, 5′-ATCGACTAAATTCATCTCAC-3′ for OsCPS2; OsKSL7-F, 5′-TTCATCTCTGTCACTTTTTCTTTTT-3′ and OsKSL7-R 5′-ATCCCAACTAAGTCATCCAC-3′ for OsKSL7. To analyze expression of CYP76M5, M6, M7, M8, primers described before were used [Citation10].
GC-MS analysis
Methanol extracts from the elicited-plant samples were analyzed by the JMS-G1000GC K9 (JEOL) mass selective detector system (ionization voltage 70 eV) coupled with a capillary column InertCap 5MS/Sil (diameter 0.25 mm, length 15 m, film thickness 0.25 μm; GL Science Inc. Tokyo, Japan) as described previously [Citation17]. To trace incorporation of [U-13C20] ent-cassa-12,15-diene, selected ion monitoring (SIM) focusing on the mass fragments distinctive to [U-13C20] ent-cassa-12,15-diene was used. For analysis of 2-deoxyphytocassane A, only GC-MS with SIM could be applicable because 2-deoxyphytocassane A cannot be detected by LC–MS/MS.
LC–MS/MS analyses of phytoalexins
The each treated sample (roughly 100 mg) was submerged in 4 ml of 80% methanol at 4 °C for 24 h, and 5 μl of the extract was subjected to liquid chromatography–tandem mass spectrometry (LC–MS/MS) analysis with the following selected reaction monitoring (SRM) transitions used as previously reported [Citation9] : momilactone A, (m/z 315 → 271); momilactone B, (m/z 331 → 269); phytocassanes A, D, and E, (m/z 317 → 299); phytocassane B, (m/z 335 → 317); phytocassane C, (m/z 319 → 301); 1-deoxyphytocassane C, (m/z 303 → 147); sakuranetin, (m/z 287 → 167). For detection of [U-13C20]-labeled phytocassane derivatives, combinations of molecular ions and precursor ions were set as described previously [Citation17]: (m/z 336 → 319) for [U-13C20] phytocassane A, D and E; (m/z 355 → 337) for [U-13C20] phytocassane B; and (m/z 339 → 321) for [U-13C20] phytocassane C.
Results
Coordinated expression of CYP71Z6–Z7 and CYP76M5–M8 mRNAs after elicitation
We previously showed that momilactone biosynthetic genes (including CYP99A2 and CYP99A3) are clustered on chromosome 4 with two diterpene cyclases (OsCPS4 and OsKSL4) and one dehydrogenase (OsMAS); we also showed that they are coordinately expressed after chitooligosaccharide elicitor treatment in suspension-cultured rice cells and in rice leaves treated with jasmonic acid or CuCl2 [Citation8,13]. Here, using rice 44 k microarray data that we previously generated with chitin-elicitor-treated rice suspension cells (GEO accession number: GSE39633), we examined the expression profiles of the phytocassane biosynthetic gene cluster on chromosome 2 (Figure (A)). The inductive expression of all genes in the cluster (except CYP71Z6) was evident, peaking around at 8 h post-elicitation (Figure (B)). These expression patterns were confirmed with quantitative real-time polymerase chain reaction (qRT-PCR), revealing a nearly identical expression profile (Figure (C)). Such coordinated expression was also detected in rice leaves treated with CuCl2 in our reported microarray data, but again, CYP71Z6 expression was not induced, indicating that this gene is likely regulated via a process different from the other genes in the cluster [Citation13]. In contrast, CYP71Z7 is located on the cluster’s central region with CYP71Z6, but was coordinately regulated with other clustered genes, including two cyclase genes (OsCPS2 and OsKSL7) and another four P450 genes (CYP76M5, M6, M7, and M8).
Figure 1. Expression profiles of phytocassane biosynthetic genes on the cluster in rice chromosome 2. (A) Schematic diagram of clustered genes for phytocassane biosynthesis. (B) Heat map of expression dynamics of the biosynthetic genes in the cluster on rice 44k microarray. Red color reveals higher expression on the microarray. (C) Validation of inductive expressions of P450 genes on the cluster by qRT-PCR using suspension cells after the elicitor treatment. Expressions of all six P450 genes except for of CYP71Z6 was shown.
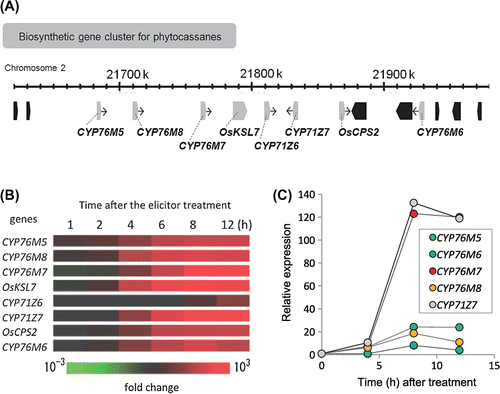
Although CYP71Z6 and CYP71Z7 exhibited high sequence similarity: 91.2% in terms of DNA and 92.3% in terms of amino acids, CYP71Z7, but not CYP71Z6, is only expressed after the elicitation, suggesting that CYP71Z7 might be catalytic enzymes that are involved in phytocassane biosynthesis. Therefore, we further focused on the in planta biological functions of CYP71Z7.
Limited forms of phytocassane production in CYP71Z7-knockout mutant
To demonstrate that CYP71Z7 is responsible for phytocassane biosynthesis, we characterized a T-DNA insertion mutant (PFG-3A-00433) obtained from Postech Biotech Center in Korea. As the mutant was originally generated with an activation enhancer vector pGA2715 [Citation18], we first checked how the insertion affected gene expression around the insertion site. We used PCR-genotyping to select the homozygous mutant population, then examined expression levels of genes in the cluster, including CYP71Z6 and CYP71Z7. We found that, in some mutant progenies, T-DNA insertion severely suppressed inductive expression of CYP71Z7, but other genes had expression that was comparable to wild type (Figure (A)). Additionally, CYP71Z6 expression levels were comparable to wild-type parental plants in some progenies, while others had higher CYP71Z6 expression than wild type (line #2 and #5), probably due to activation from the enhancer. Therefore, we used cyp71z7 knockout plants with moderate or strongly increased CYP71Z6 expression in subsequent analyses (Figure (B)).
Figure 2. Expression of P450 genes on the cluster and accumulation of phytoalexins in cyp71z7 T-DNA tagged line treated with CuCl2. (A) qRT-PCR analysis of two diterpene cyclase genes and five P450 genes. Two independent plants of cyp71z7 T-DNA mutants were analyzed with wild-type plant. (B) Expression of CYP71Z6 in the mutants and wild-type plant. (C–E) Accumulation of phytoalexins in wild-type and cyp71z7 T-DNA mutants. (C) Momilactones, (D), phtocassane A, B, D (C2-oxygenated), (E) phytocassane C and E (C2-non-oxygenated). Relative expression level was calculated using the standard curve method normalized by the raw data of the ubiquitin domain-containing protein gene (OsUBQ).
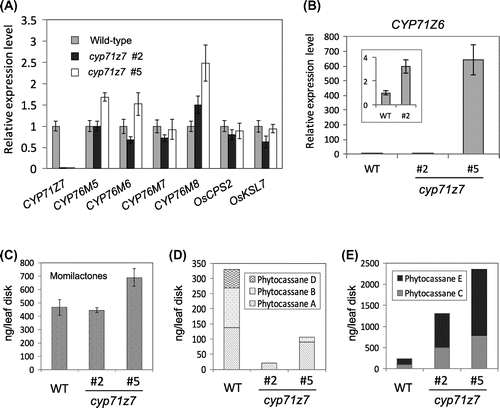
The leaves of cyp71z7 homozygous mutant #2 (with moderate enhancement of the CYP71Z6 expression) was treated with CuCl2 for measuring phytoalexin accumulation. The mutant leaves produced momilactones at the similar levels as seen in wild-type (Figure (C)), but did not produce phytocassane A, B, and D (Figure (D)). However, we observed a predominant accumulation of phytocassane C and phytocassane E in the mutant leaves (Figure (E)). These results suggested that CYP71Z7 is involved only in biosynthesis of C2-oxygenated phytocassanes.
In the cyp71z7 mutant #5 overexpressing CYP71Z6 (600-times increased expression to that of wild-type), we observed partial compensation of the mutant’s original low C2-oxygenated phytocassanes accumulation (Figure (B) and (D)). Although native CYP71Z6 expression was not enough to fully counteract CYP71Z7 loss-of-function, our result implied that CYP71Z6 is potentially able to catalyze position C2-hydroxylation besides CYP71Z7.
Discovery of possible intermediates for phytocassane in CYP71Z7–defective mutant
Previously, we synthesized several phytocassane-derivative compounds to understand the absolute configuration of natural phytocassanes [Citation19]. These synthesized compounds include 1-deoxyphytocassane C and 2-deoxyphytocassane A, both possible precursors of phytocassane C (due to position 1 hydroxylation of 1-deoxyphytocassane C), as well as phytocassane A (due to position 2 hydroxylation of 2-deoxyphytocassane A). Alternatively, 1-deoxyphytocassane C can be metabolized into phytocassane B through the intermediate 1-deoxyphytocassane B, generated via position 2 hydroxylation of 1-deoxyphytocassane C. Additionally, 2-deoxyphytocassane A can also be metabolized into phytocassane E through position 1 hydroxylation. Hence, both 1-deoxyphytocassane C and 2-deoxyphytocassane A are likely important intermediates of phytocassanes, but so far no evidence is available to indicate that these compounds naturally exist in rice. Here, gas chromatography-mass spectrometry (GC-MS) analysis revealed that methanol extracts from cholic acid-treated rice suspension cells contain 1-deoxyphytocassane C and 2-deoxyphytocassane A as endogenous compounds (Figure ), strongly suggesting that they are intermediates for phytocassane biosynthesis.
Figure 3. GC-MS analysis of methanol extract from suspension cultured rice cells treated with cholic acids. (A) Total ion chromatogram of the methanol extract. (B) Mass spectrum of peak 1. (C) Mass spectrum of authentic 2-deoxyphytocassane A. (D) Mass spectrum of peak 2. (E) Mass spectrum of authentic 1-deoxyphytocassane C.
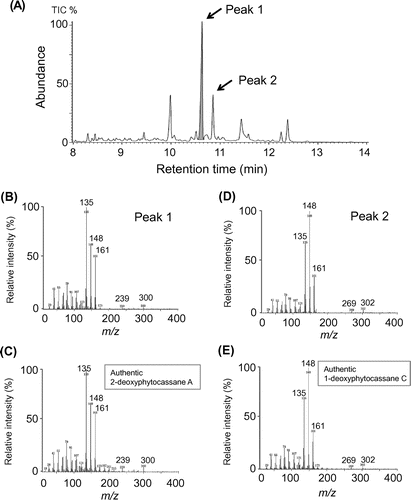
We next examined whether non-C2-oxygenated phytocassanes intermediates (e.g., 1-deoxyphytocassane C and 2-deoxyphytocassane A) are measurably accumulated in the cyp71z7 mutant. Liquid chromatography–mass spectrometry (LC–MS/MS) and GC-MS analyses clearly demonstrated that 1-deoxyphytocassane C (compound III) and 2-deoxyphytocassane A (compound IV) were highly accumulated in post-elicitation mutant leaves (Figure ). Therefore, given that the cyp71z7 mutant cannot produce phytocassanes with a position C2 hydroxyl group, the substrate of CYP71Z7 is probably position C2-non-oxygenated phytocassane derivatives.
Figure 4. GC-MS and LC–MS/MS analyses of methanol extract from leaves of wild-type and cyp71z7 mutant treated with CuCl2. (A) Mass chromatogram by selected ion monitoring at m/z 161 for 2-deoxyphytocassane A. (B) LC–MS/MS SRM chromatogram (m/z 303 → 147) for 1-deoxyphytocassane C. Amount of the accumulation was calculated per leaf disk from peak area at RT = 8 min.
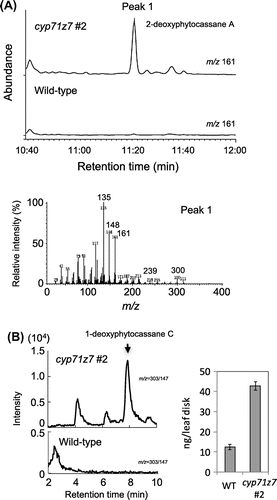
Phytocassane deficiency and phenotype of CYP76M7/8 knockdown plants
Expression analysis has shown that post-elicitation expression of CYP76M5, M6, M7, and M8 was coordinately regulated. This P450 family of P450 has various biochemical functions that do not all involve phytocassane production [Citation20]. CYP76M7 is known to catalyze 11α-hydroxylation of ent-cassa-12,15-diene, while CYP76M8 is a multifunctional/promiscuous hydroxylase that can perform position C11-hydroxylation of ent-cassa-12,15-diene. To better understand in planta CYP76M7/M8 function, we tested previously generated CYP76M7/M8 knock-down RNAi lines [Citation12].
CYP76M5–M8 expression levels in three independent CYP76M7/M8 RNAi plants were first confirmed via qRT-PCR at 24 h post-CuCl2 treatment. We found that CYP76M7 and M8 mRNA were selectively suppressed, but not other CYP genes in the cluster, including CYP71Z7 (Figure (A)). This suppression profile is consistent with previous results from suspension-cultured rice cells of this RNAi line.
Figure 5. Expression of P450 genes on the cluster in CYP76M7/8 RNAi. (A) qRT-PCR analysis of four P450 genes in CYP76M family and CYP71Z7. Three independent lines were analyzed together with wild-type plant. (B) Accumulation of phytoalexins in those plants. T-DNA mutants. Total amount of phytocassanes and momilactoes were shown as per leaf disk.
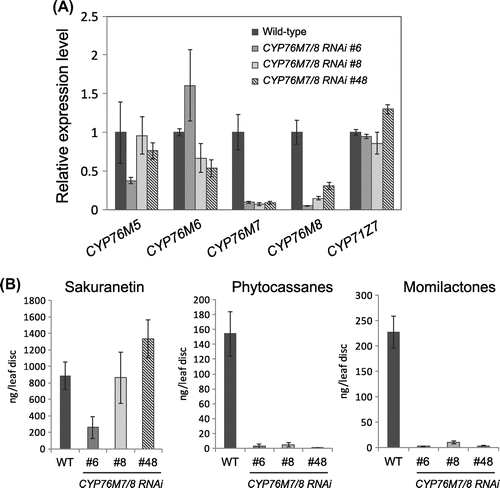
Phytoalexin accumulation in leaves of the CYP76M7/M8 RNAi lines was measured after CuCl2 treatment. The mutant leaves did not exhibit accumulation of any phytocassane (A, B, C, D, and E); furthermore, momilactone accumulation decreased, while sakuranetin (a flavonoid phytoalexin) accumulated at a wild-type levels (Figure (B)). These results suggested that either CYP76M7 or M8, or both, is involved in phytocassane and momilactone production.
We then used GC-MS to identify possible intermediate compounds, so as to pinpoint which step CYP76M7/M8 catalyzes in the biosynthetic pathway. The analysis detected two peaks (Peak 3 and 4) in the CYP76M7/M8 RNAi lines that were enhanced compared with peaks in CuCl2-treated wild-type plants (Figure (A)). Based on the mass spectra pattern, we confirmed that peak 3 was identical to 3α-hydroxy-ent-cassadiene, identified as the product of an in vitro assay with CYP701A8 using ent-cassa-12,15-diene as a substrate (Figure (B)) [Citation12]. We also found that peak 4’s mass spectrum was almost identical to the spectrum of 3α-hydroxy-ent-cassadien-2-one, the compound previously produced in vitro when CYP71Z7 was used to catalyze ent-cassa-12,15-diene as a substrate (Figure (C)) [Citation21]. These results imply that the presence of two successive intermediates derived from ent-cassa-12,15-diene that lead to phytocassanes: 3α-hydroxy-ent-cassadiene (compound I) and 3α-hydroxy-ent-cassadien-2-one (compound II). Both are likely substrates for CYP76M7 or M8.
Figure 6. GC-MS analysis of methanol extract from leaves of CYP76M7/8 RNAi lines treated with CuCl2 (A) Total ion chromatogram of the methanol extract. (B) Mass spectrum of peak 3. (C) Mass spectrum of peak 4. Based on the reported mass spectra by Kitaoka et al. [Citation21] and Wang et al. [Citation10], these compounds could be assigned as 3α-hydroxy-ent-cassadiene (B) and 3α-hydroxy-ent-cassadien-2-one (C).
![Figure 6. GC-MS analysis of methanol extract from leaves of CYP76M7/8 RNAi lines treated with CuCl2 (A) Total ion chromatogram of the methanol extract. (B) Mass spectrum of peak 3. (C) Mass spectrum of peak 4. Based on the reported mass spectra by Kitaoka et al. [Citation21] and Wang et al. [Citation10], these compounds could be assigned as 3α-hydroxy-ent-cassadiene (B) and 3α-hydroxy-ent-cassadien-2-one (C).](/cms/asset/4e60f98c-487e-4855-a6d8-e14f34f6fd89/tbbb_a_1398067_f0006_b.gif)
In addition to phytocassane depletion in the CYP76M7/M8 RNAi lines, we observed a lesion-mimic-like phenotype in the expanded adult leaves. Because the RNAi line does not produce phytocassanes or momilactones (except sakuranetin), microbial infections may have caused the lesions. To test this hypothesis, we observed the surface and sections of CYP76M7/M8 RNAi leaves under a microscope. We did not observe typical disease symptoms and few microbes- or fungal spore-like structures were detected (Supplemental Figure 1), implying that microbial infection did not cause the lesions. These results suggested that accumulated diterpenoid intermediates (e.g., 3α-hydroxy-ent-cassadiene) could have a deleterious effect in the CYP76M7/M8 RNAi plants.
Discussion
Here, we examined the in planta function of CYPs in the phytocassane biosynthetic gene cluster on rice chromosome 2. To date, momilactone gene clusters have been found in cultivated rice (Oryza sativa), wild rice (e.g., O. rufipogon and O. punctata) [Citation9], and possibly barnyard grass (Echinochloa crus-galli) [Citation7]. Similarly, Leersia perrieri, a close relative to Oryza, also contains a phytocassane biosynthetic gene cluster. This plant only produces non-C2-oxygenated phytocassanes (e.g., phytocassanes C and E), not C2-oxygenated types (e.g., phytocassanes A, B, D). Genome information of the phytocassane biosynthetic gene cluster in L. perrieri chromosome 2 reveals that CYP71Z6/7-related P450 genes are not present, but CYP76M5/6/14-related P450 genes are. Indeed, CYP71Z7 is most likely involved in position C2-hydroxylation of phytocassane, based on our results from the cyp71z7 T-DNA mutants. Presumably, phytocassane C and E are conserved phytocassanes during the evolution of chemical structure.
Although the expression of tandemly located CYP71Z6 in the CYP71Z7 mutant was strongly enhanced due to the enhancer module inserted into the CYP71Z7 coding region, we still observed a clear decrease in C2-oxygenated phytocassanes A, B, D in the mutant. This result clearly indicates that CYP71Z7, but not CYP71Z6, is involved in phytocassane C2-hydroxylation. A possible function of CYP71Z6 is to perform position C2-hydroxylation on ent-isokaurene, leading to the constitutively produced antimicrobial compound, Oryzaride A (a phytoanticipin) [Citation10]. CYP71Z6 is not likely responsible for phytocassane production, given its constitutive low expression that cannot be induced to the levels of the CYP71Z7 expression (Figure ). Moreover, the phytocassane-deficient phenotype of CYP76M7/8 RNAi lines unequivocally indicated CYP76M7/8 involvement in the early steps of phytocassane biosynthesis after ent-cassadiene formation.
Possible phytocassane intermediates were discovered when analyzing the cyp71z7 T-DNA mutant and CYP76M7/8 RNAi lines. The former accumulated 1-deoxyphytocassane C (compound III) and 2-deoxyphytocassane A (compound IV), both potential substrates of CYP71Z7 to yield 1-deoxyphytocassane B. Further modification via position C1-hydroxylation or ketone formation could then lead to phytocassane B and D, respectively. In contrast, CYP76M7/8 RNAi lines could not produce phytocassanes but accumulated 3α-hydroxy-ent-cassadiene and 3α-hydroxy-ent-cassadien-2-one; both are possible substrates of 1-deoxyphytocassane C and phytocassane D after position C11-hydroxylation or ketone formation, respectively. Additionally, 3α-hydroxy-ent-cassadiene is a plausible substrate of CYP76M7/8, forming 1-deoxyphytocassane C. This conclusion is based on previous reports showing that 3α-hydroxy-ent-cassadiene is the in vitro product after CYP701A8 catalysis, while CYP76M7/8 has been shown to catalyze C11-hydroxylation of ent-cassadiene. Both CYP76M7 and M8 catalyze C11-hydroxylation, but the former is more critical to the reaction, whereas the latter can also hydroxylate other types of diterpene hydrocarbons besides ent-cassadiene [Citation10]. Taken together, these results suggest that C11-hydroxylation of 3α-hydroxy-ent-cassadiene and 3α-hydroxy-ent-cassadien-2-one by CYP76M7/8 occurs after C3-hydroxylation of ent-cassadiene.
Based on current and previous findings, we propose several hypothetical pathways of phytocassane biosynthesis in rice (Figure ). After OsCPS2 and OsKSL7 synthesize ent-cassadiene from geranylgeranyl diphosphate (GGDP), CYP701A8 hydroxylates ent-cassadiene on position C3 to produce 3α-hydroxy-ent-cassadiene (compound I). This intermediate is further oxidized at position C11 by CYP76M7/8 to yield 1-deoxyphytocassane C (compound III), whereas position C11 ketone formation could be catalyzed by an unknown enzyme probably dehydrogenase. Subsequently, CYP71Z7 catalyzes position C2-hydroxylation on 1-deoxyphytocassane C to yield 1-deoxyphytocassane B, which is then converted into phytocassane B and D. Additionally, dehydrogenation of the position C3-hydroxy group in 1-deoxyphytocassane C yields 2-deoxyphytocassane A (compound IV), a potential substrate that forms phytocassane A and E. To obtain phytocassane C and E, 1-deoxyphytocassane C and 2-deoxyphytocassane A are hydroxylated by an unknown enzyme at position C1. Both biochemical and genetic approaches will be necessary to identify the missing enzymes in these reactions.
Figure 7. Proposed potential routes of phytocassane biosynthesis occurring in planta. Solid arrows indicate the biosynthetic process with experimental evidences of enzymes involved. Dashed arrows indicate the steps with unknown enzymes or chemical reaction in the biosynthetic pathway. Possible candidate enzymes involving unidentified dehydrogenation or monooxygenation were indicated as DHase and OHase, respectively, on the pathway.
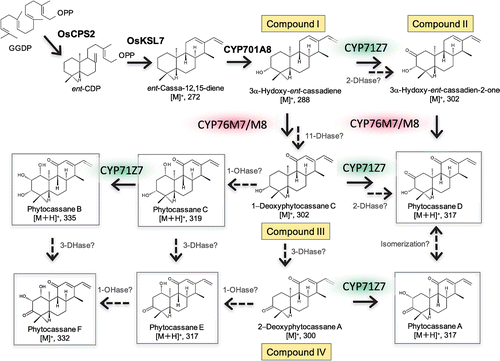
CYP76M7/M8 suppression caused lesions on expanded leaves 3α-hydroxy-ent-cassadiene accumulation. Microscopic observation revealed that pathogenic microorganisms were not the source of lesions. Instead, lesions were possibly induced by accumulated C3-hydroxylated ent-cassadiene, an intermediate that is harmful to plants. Similarly, in Arabidopsis, dwarfing resulted after thalianol synthase overexpression caused triterpene thalianol accumulation, probably because thalianol was metabolized to desaturated thaliana-diol, another compound detrimental to plant growth [Citation4].
We have been attempting to trace candidate intermediates in the phytocassane biosynthetic pathway using our recently established approach involving uniformly 13C-labeled ent-cassadiene [Citation17]. The mutant plants accumulate significantly more 13C-labeled phytocassane C and E, but not 13C-labeled phytocassane B, compared to wild type (Supplemental Figure 2), consistent with the result that mutants contain high concentrations of position 2 non-hydroxylated phytocassanes. The GC-MS analysis using SIM of potential intermediates 1-deoxyphytocassane C and 2-deoxyphytocassane A reveals a small but significant 13C-labeled signature distinctive to [U-13C20] ent-cassa-12,15-diene (e.g., m/z 44, 59, 85, 98, 128, 160, 173 and 201), which is not found in wild-type plants (Supplemental Figure 2), suggesting that bioconversion of ent-cassa-12,15-diene to 2-deoxyphytocassane A does occur in planta. The same approach to the CYP76M7/M8 RNAi lines reveals significant signals of [U-13C20]-labeled 3-hydroxylated ent-cassadiene that are distinctive to [U-13C20] ent-cassa-12,15-diene (Supplemental Figure 2). These observations suggest that the intermediates most likely come from ent-cassa-12,15-diene and are probable candidate substrates of CYP76M7/M8 in planta, presumably yielding 1-deoxyphytocassane C, a 11-oxygenated 3α-hydroxy-ent-cassadiene. Currently, the incorporation of 13C-cassadiene into the intermediates are not clear enough to obtain full mass spectra of the [U-13C20]-labeled compound. Thus, the incorporation efficiency of 13C-cassadiene must be improved. One possible method of enhancing 13C-incorporation in plants would be to use genetically modified plants that are defective in ent-cassadiene production, such as generating an OsKSL7-knock-out through genome editing.
In conclusion, based on our characterization of CYP71Z7- and CYP76M7/M8-suppressed plants, we described a promising phytocassane biosynthetic pathway that actually occurs in planta and identified novel candidate intermediates. Our future studies will aim to fill the gaps in the proposed pathway through uncovering currently unknown enzymes that are probably encoded by genes outside of the cluster.
Author contribution
K.O., H.Y. designed and supervised the study; Z.Y., K.Y., H.M., S.M., K.M. perform experiments and data analyses; Z.Y., K.O wrote the manuscript with the assistance of H.N., H.K.
Funding
This work was supported in part by JSPS KAKENHI to K.O. [grant number 17H03811].
Disclosure statement
No potential conflict of interest was reported by the authors.
Supplemental data
Supplemental data for this article can be accessed at https://doi.org/10.1080/09168451.2017.1398067.
supplemental_figs.pdf
Download PDF (764.1 KB)Acknowledgments
We appreciate Prof Jinichirou Koga (Teikyo University) for supplying us with phytocassanes and ADEKA Co. Ltd. for supplying us with 13C-MVA and technical support. We thank Dr. Gynheung An (Institution and Pohang University of Science and Technology) for providing the cyp71z7 mutant.
References
- Ahuja I, Kissen R, Bones AM. Phytoalexins in defense against pathogens. Trends Plant Sci. 2012;17:73–90.10.1016/j.tplants.2011.11.002
- Qi X, Bakht S, Leggett M, et al. A gene cluster for secondary metabolism in oat: Implications for the evolution of metabolic diversity in plants. Proc Natl Acad Sci USA. 2004;101:8233–8238.10.1073/pnas.0401301101
- Frey M, Chomet P, Glawischnig E, et al. Analysis of a chemical plant defense mechanism in grasses. Science. 1997;277:696–699.10.1126/science.277.5326.696
- Field B, Osbourn AE. Metabolic diversification-independent assembly of operon-like gene clusters in different plants. Science. 2008;320:543–547.10.1126/science.1154990
- Field B, Fiston-Lavier AS, Kemen A, et al. Formation of plant metabolic gene clusters within dynamic chromosomal regions. Proc Natl Acad Sci USA. 2011;108:16116–16121.10.1073/pnas.1109273108
- Shang Y, Ma Y, Zhou Y, et al. Biosynthesis, regulation, and domestication of bitterness in cucumber. Science. 2014;346:1084–1088.10.1126/science.1259215
- Guo L, Qiu J, Ye C, et al. Echinochloa crus-galli genome analysis provides insight into its adaptation and invasiveness as a weed. Nat Commun. 2017;8:1031.
- Shimura K, Okada A, Okada K, et al. Identification of a biosynthetic gene cluster in rice for momilactones. J Biol Chem. 2007;282:34013–34018.10.1074/jbc.M703344200
- Miyamoto K, Fujita M, Shenton MR, et al. Evolutionary trajectory of phytoalexin biosynthetic gene clusters in rice. Plant J. 2016;87:293–304.10.1111/tpj.2016.87.issue-3
- Wang Q, Hillwig ML, Okada K, et al. Characterization of CYP76M5–8 indicates metabolic plasticity within a plant biosynthetic gene cluster. J Biol Chem. 2012;287:6159–6168.10.1074/jbc.M111.305599
- Wu Y, Hillwig ML, Wang Q, et al. Parsing a multifunctional biosynthetic gene cluster from rice: Biochemical characterization of CYP71Z6 & 7. FEBS Lett. 2011;585:3446–3451.10.1016/j.febslet.2011.09.038
- Wang Q, Hillwig ML, Wu Y, et al. CYP701A8: a rice ent-kaurene oxidase paralog diverted to more specialized diterpenoid metabolism. Plant Physiol. 2012;158:1418–1425.10.1104/pp.111.187518
- Ogawa S, Miyamoto K, Nemoto K, et al. OsMYC2, an essential factor for JA-inductive sakuranetin production in rice, interacts with MYC2-like proteins that enhance its transactivation ability. Sci Rep. 2017;7:40175.10.1038/srep40175
- Cho EM, Okada A, Kenmoku H, et al. Molecular cloning and characterization of a cDNA encoding ent-cassa-12,15-diene synthase, a putative diterpenoid phytoalexin biosynthetic enzyme, from suspension-cultured rice cells treated with a chitin elicitor. Plant J. 2004;37:1–8.10.1046/j.1365-313X.2003.01926.x
- Okada A, Shimizu T, Okada K, et al. Elicitor induced activation of the methylerythritol phosphate pathway toward phytoalexins biosynthesis in rice. Plant Mol Biol. 2007;65:177–187.10.1007/s11103-007-9207-2
- Shimizu T, Jikumaru Y, Okada A, et al. Effects of a bile acid elicitor, cholic acid, on the biosynthesis of diterpenoid phytoalexins in suspension-cultured rice cells. Phytochemistry. 2008;69(4):973–981.10.1016/j.phytochem.2007.10.005
- Ye Z, Nakagawa K, Natsume M, et al. Biochemical synthesis of uniformly 13C-labeled diterpene hydrocarbons and their bioconversion to diterpenoid phytoalexins in planta. Biosci Biotechnol Biochem. 2017;81:1176–1184.10.1080/09168451.2017.1285689
- Yi J, An G. Utilization of T-DNA tagging lines in rice. J Plant Biol. 2013;56:85–90.10.1007/s12374-013-0905-9
- Yajima A, Mori K. Absolute configuration of phytocassanes as proposed on the basis of the CD spectrum of synthetic (+)-2-deoxyphytocassane A. Tetrahedron Lett. 2000;41:351–354.10.1016/S0040-4039(99)02060-2
- Kitaoka N, Lu X, Yang B, et al. The application of synthetic biology to elucidation of plant mono-, sesqui-, and diterpenoid metabolism. Mol. Plant. 2015;8:6–16.10.1016/j.molp.2014.12.002
- Kitaoka N, Wu Y, Xu M, et al. Optimization of recombinant expression enables discovery of novel cytochrome P450 activity in rice diterpenoid biosynthesis. Appl Microbiol Biotechnol. 2015;99:7549–7558.10.1007/s00253-015-6496-2