Abstract
Malate dehydrogenase (EC 1.1.1.37) was purified to homogeneity from the phototrophic purple non-sulfur bacterium Rhodovulum steppense A-20s. According to gel-chromatography and electrophoretic studies, malate dehydrogenase is present as a dimer, tetramer and octamer depending on cultivation conditions. In phototrophic aerobic conditions only the tetrameric form was present, in chemotrophic aerobic conditions all three forms were detected, while in the absence of oxygen the octameric form disappeared. The malate dehydrogenase oligomers are encoded by a single gene and composed of the same 35 kDa polypeptide but differ in pH and temperature optimum, in affinities to malate, oxaloacetate, NADH and NAD+ and in regulation by cations and citrate. By modulating the cultivation conditions, it has been established that the dimer participates in the glyoxylate cycle; the tetramer operates in the tricarboxylic acid cycle, and the octamer may be involved in the adaptation to oxidative stress.
Graphical abstract
CXCL10 as a novel exercise-reducible myokine.
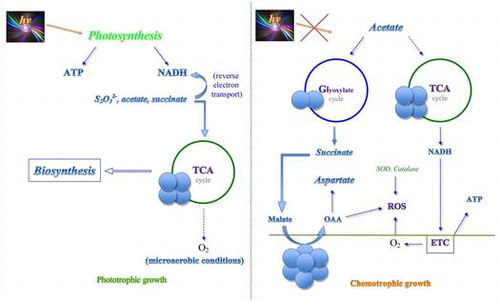
Anoxygenic phototrophic purple non-sulfur bacteria possess a high variability of adaptive mechanisms to stress conditions and are able to survive in alkaline environments at high salt concentrations [Citation1,2]. Purple phototropic bacteria can switch from photosynthesis to heterotrophic growth depending on the presence of light and oxygen. In these processes, the regulation of redox status in cells via interconversion of malate plays a central role. Malate dehydrogenase (MDH; EC 1.1.1.37) catalyzing the interconversion between malate and oxaloacetate (OAA) using NAD+ as a cofactor is a key enzyme of the central metabolism. Its high activity results in rapid equilibration of NADH/NAD+ and malate/OAA ratios [Citation3,4] thus maintaining cellular redox status. MDH has a universal distribution linking the reactions of the tricarboxylic (TCA) cycle to other metabolic pathways and facilitating microbial adaptation to different stress factors [Citation5–7].
Earlier we established the role of MDH oligomeric forms in the adaptive reaction to different types of cultivation in the colorless sulfur bacterium Beggiatoa leptomitiformis [Citation8,9] and in other meso- and thermophilic bacteria [Citation10–12]. It was shown that MDH is a multifunctional enzyme supporting such processes as cellular respiration, TCA cycle, glyoxylate cycle, nitrogen metabolism via aspartate synthesis, osmolyte formation etc. Rhodovulum steppense is a perfect organism to study specific MDH oligomeric forms in differentiating of metabolic fluxes depending on phototrophic or heterotrophic growth. The initial work [Citation13] revealed that MDH exists in these bacteria in different oligomeric forms. The goal of the present study was to isolate individual oligomeric forms of MDH from the bacterium Rhodovulum steppense cultivated in phototrophic conditions and in chemotrophic conditions in the presence or absence of oxygen, to study their regulatory and functional characteristics, and to determine their possible physiological role.
Materials and methods
Growth conditions and lysis of cells
The phototrophic haloalcaliphilic purple non-sulfur bacterium Rhodovulum steppense A-20s [Citation14] (the strain was obtained from Dr. Elena I. Kompantseva, Winogradsky Institute of Microbiology of the Russian Academy of Sciences) was cultivated in aerobic and anaerobic conditions in darkness and in anaerobic conditions in the presence of light. The Pfenning’s cultivation medium contained in 1 L 0.33 g Na2SO4, 0.33 g KCl, 0.33 g NH4Cl, 0.33 g KH2PO4, 0.33 g MgCl2·6H2O, 10 g NaCl, 5 g NaHCO3, 0.3 g Na2S·9H2O, 1 g sodium acetate, 0.1 g yeast extract and 1 mL of 1 mg L−1 microelement and vitamin solution. The cell suspension was obtained by centrifugation at 8,000 g for 10 min at 4 °C. The cells were washed by 50 mM tris-HCl buffer, pH 8.5 and lysed using the ultrasound disintegrator UZDN-2T (Ukrrospribor, Ukraine) at the power 500 W and frequency 22 kHz for 2 min on ice. The supernatant (enzyme extract) from centrifugation at 4000 g for 10 min at 4 °C was used for MDH measurement and purification as well as for the study of other enzymes.
Purification of malate dehydrogenase and measurement of its activity
The activity of malate dehydrogenase (MDH; EC 1.1.1.37) in the forward and reverse reaction was measured spectrophotometrically at 340 nm. Oxaloacetate (OAA) reduction was determined in 50 mM tris-HCl buffer, pH 8.5, containing 1 mM OAA and 0.15 mM NADH. Malate oxidation was measured in the same buffer containing 4 mM malate and 1 mM NAD+. Because of the equilibrium favoring OAA reduction, malate oxidation was measured during first 20 s when NADH formation was strictly linear. One unit of MDH activity corresponded to the amount of enzyme which oxidized 1 μmol of NADH or reduced 1 μmol of NAD+ in 1 min at 25 °C. The kinetic studies and determination of constants were performed according to [Citation15]. Metal ions were applied as chloride salts.
Purification of MDH was performed at 4 °C and included gel filtration of 4 mL enzyme extract on Sephadex G-25 column (1.5 × 10 cm) equilibrated with 50 mM tris-HCl buffer, pH 8.5 and ion-exchange chromatography on DEAE cellulose column (1.5 × 12 cm) equilibrated with 50 mM tris-HCl buffer, pH 8.0. Desorption of MDH was performed using the linear KCl gradient (220–300 mM), 2 mL fractions were collected. Further purification was achieved by loading active fractions from DEAE cellulose to the column (2 × 40 cm) of Sephadex G-200, this column was also used for molecular weight determination according to [Citation16]. The obtained preparations of all three MDH forms were close to electrophoretic homogeneity on the native and SDS-PAGE. The activity during purification was measured in the direction of OAA reduction.
SDS-PAGE was performed at the concentration of PAAG 12.5%. For determination of subunit molecular weight the following marker proteins were used: β-galactosidase (116 kDa), bovine serum albumin (66.2 kDa), lactate dehydrogenase (35 kDa), Bsp98 l restriction enzyme (25 kDa), β-lactoglobulin (18.4 kDa), and lysozyme (14.4 kDa). Determination of the purity of MDH forms was performed by the silver staining method [Citation17].
Native PAGE was performed by the method of Davis [Citation18] with the concentration of acrylamide in running gel of 8%. For the specific staining of MDH the gels were incubated in 50 mM tris-glycine buffer, pH 7.2, containing 10 mM sodium malate, 5 mM MgCl2, 400 μg mL−1 nitrotetrazolium blue 40 μg mL−1 phenazine methosulfate, 2 mM NAD+ [Citation19,20].
Measurement of isocitrate lyase activity
Isocitrate lyase activity was measured spectrophotometrically by an increase of optical density at 324 nm (ε = 0.18 mM−1 cm−1) due to the formation of the complex between glyoxylate and phenylhydrazine [Citation21]. The assay medium contained 50 mM tris-HCl buffer, pH 8.5, 4 mM MgCl2, 4 mM dithiothreitol, 2 mM D,L-isocitrate, 4 mM phenylhydrazine-HCl. The unit of activity was determined as the amount of enzyme producing 1 μmol of glyoxylate in 1 min at 25 °C. Catalase activity was measured according to [Citation22] at 240 nm. SOD activity was measured via photochemical reduction of nitroblue tetrazolium [Citation23] and expressed in arbitrary units. One unit corresponded to the amount of enzyme suppressing the reaction of nitroblue tetrazolium reduction by 50%. Total protein content was measured by the method of Lowry et al. [Citation24].
The specific staining of isocitrate lyase on native gels was performed in 50 mM tris–HCl buffer, pH 7.5, containing 1 mM EDTA, 3 mM MgCl2, 3 mM dithiothreitol, 10 mM D,L-isocitrate, 1.2 mL of modified Schiff reagent [Citation25]. The gels were incubated at 37 °C until the red bands indicating isocitrate lyase were developed. The inhibitor of isocitrate lyase itaconate was added in the cultivation medium in the concentration 5 mM.
Identification of malate dehydrogenase gene
For isolation of total RNA the reagent ExtractRNA (Eurogene, Russia) was used. Visualization of the isolated DNA and RNA was performed by electrophoresis in 1% agarose gel. Reverse transcription of mRNA was performed using the reverse transcriptase of the Moloney virus of mice leucosis M-MULVRT (Fermentas, Lithuania) with a random primer for synthesis of the first chain of cDNA according to manufacturer’s instruction. The degenerate primers were selected on the basis of conserved regions of aligned malate dehydrogenase gene sequences from bacteria of different taxonomic groups with the help of the software AliBee Multiple Alignment and Primer-BLAST. Determination of the nucleotide sequence of obtained amplicon of the mdh gene was performed by the company “Eurogene” (Moscow, Russia). The selection of specific primers was based on the nucleotide sequence of amplicon of the mdh gene from Rhodovulum steppense using Primer-BLAST programme: forward 5′-GCTTGACGCGATGGTTTGGG-3′; reverse, 5′- AGCCCATGTCCACCAGATCG-3′. The PCR was performed using degenerate and specific primers in the amplifier Tercik (DNA-Technology, Moscow, Russia).
RT-PCR was conducted on LightCycler 96 (Roche, Switzerland) using SYBR Green I as a dye. Amplification included the initial denaturation at 95 °C for 5 min, then 35 cycles of 20 s at 95 °C, 20 s at 59 °C, 20 s at 72 °C (detection), followed by the final elongation for 4 min at 72 °C. The amount of template was controlled by parallel amplification of 16S rRNA with gene-specific primers. The total RNA not subjected to reverse transcription was taken as a negative amplification control. The relative level of expression of the studied genes was determined by the 2−ΔΔCT method [Citation26].
Mass spectrometric analysis of malate dehydrogenase peptides
Mass spectrometric measurements were performed using the equipment of “Human Proteome” Core Facility of the Institute of Biomedical Chemistry (Russia, Moscow) which is supported by Ministry of Education and Science of the Russian Federation (unique project ID RFMEFI62117X0017). Protein bands from gels were in situ digested with modified trypsin as described [Citation17]. Peptide mass fingerprint analysis of MS spectra was performed using Ultraflex II MALDI TOF mass spectrometer (Bruker Corporation, Bremen, Germany).
Statistical analysis
The experiments were repeated three times, the analytic assays in each sample were done in triplicate. The data on figures are the means of three biological repeats. The statistically significant differences at p < 0.05 are discussed. The kinetic constants were determined using the methods of linear and parabolic approximation [Citation15].
Results
Oligomeric forms of malate dehydrogenase from Rhodovulum steppense
MDH in the bacteria cultivated chemotrophically in aerobic conditions was detected on non-denaturing gels in three molecular forms, while the enzyme from bacteria cultivated autotrophically in anaerobic conditions was present only in one molecular form. The form having the lowest electrophoretic mobility (octamer) disappeared in the cultures grown chemotrophically but anaerobically (Figure (A)). All forms were purified from the extracts of chemotrophically grown bacteria. They were homogeneous as determined by the SDS-PAGE (Figure (B)) and exhibited specific activities of 4.2, 3.9 and 4.9 μmol NADH min−1 mg−1 protein in the reaction of oxaloacetate reduction with the times of purification of 65, 60 and 71 for MDH1 (having low electrophoretic mobility), MDH2 (intermediate electrophoretic mobility) and MDH3 (high electrophoretic mobility) correspondingly. The subunit molecular weight was the same for all subunits and determined as 35 kDa. The comparison of subunit molecular weight determined by SDS-PAGE with molecular weights of three forms determined by gel chromatography revealed that MDH1 is an octamer, MDH2 is a tetramer, and MDH3 is a dimer (Figure , Table ).
Figure 1. Zymograms of malate dehydrogenase (MDH) from Rhodоvulum steppense A-20s. A – Specific staining of MDH activity from the extracts of bacteria grown phototrophically (a), chemotrophically in the presence of oxygen (b), and chemotrophically in the absence of oxygen (c). B – Silver staining of MDH forms on SDS zymograms after purification. Marker enzymes: 1 – β-galactosidase; 2 – bovine serum albumin; 3 – ovalbumin; 4 – lactate dehydrogenase; 5 – Bsp98 l restriction enzyme; 6 – β-lactoglobulin; 7 – lysozyme; F – bromophenol blue.
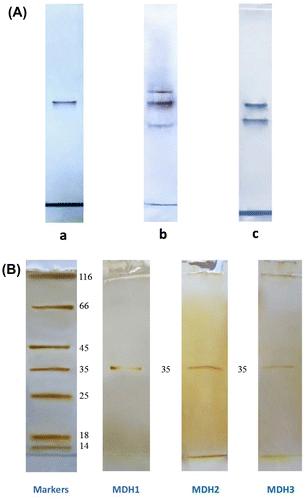
Table 1. Structural, kinetic and regulatory properties of oligomeric forms of MDH from Rhodovulum steppense A-20s. The data are means from three biological replicates. The statistically significant differences at p < 0.05 are discussed in the text.
Purified preparations of MDH oligomeric forms were used to study their physicochemical, kinetic and regulatory properties. The catalytic properties (affinity to substrates and cofactors, inhibition by OAA in the reaction of malate oxidation and by citrate in the reaction of OAA reduction, pH and temperature optimum) differed for all three oligomeric forms (Table ). There were marked differences in the activation by Mg2+ and inhibition by Ba2+. Ca2+ slightly (11–15%) activated all MDH oligomeric forms in the micromolar range and inhibited at millimolar concentrations. Mn2+ was slightly inhibitory for all oligomeric forms (not shown).
Malate dehydrogenase gene and its expression
The PCR analysis of cDNA using degenerate primers followed by the electrophoresis of reaction products in 1% agarose gel revealed one amplicon band on the gel, which confirms the presence in total RNA of the product of expression of the single gene encoding MDH in the bacterium Rhodovulum steppense. The purified nucleotide band was sequenced and compared with the GenBank database. The amplicon had a high homology with MDH genes of other microorganisms. For example, the similarity with Rhodobacter sphaeroides 2.4.1 (CP000143.2) gene was 85%, with Rhodopseudomonas palustris BisB181 (CP000301.1) gene was 82%, and with Rhodovulum sulfidophilum DSM 1374 (CP015418.1) gene it was 91% (Figure ).
Figure 2. Nucleotide sequence of the amplicon of mdh gene from Rhodovulum steppense (Seq) and of the corresponding fragment of mdh gene from Rhodopseudomonas palustris, Rhodobacter sphaeroides, Rhodovulum sp. MB263 and Rhodovulum sulfidophilum.
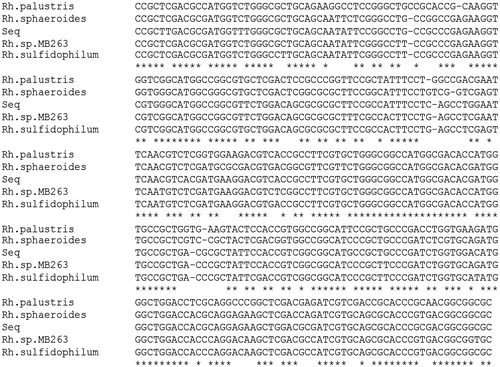
The PCR analysis of cDNA using gene-specific primers was separation by electrophoresis in 1% agarose gel showed one band on the gel. This confirms the specific binding of primers with the cDNA. The length of the product of the PCR analysis was 296 base pairs (Figure (A)). The specificity of primers was confirmed also by re-amplification of the PCR product extracted from the gel.
Figure 3. Amplification of malate dehydrogenase gene and its expression. A – Electrophoregram of the PCR product of mdh gene of Rhodоvulum steppense amplified with specific primers in 1% agarose gel. M – DNA markers (100–1000 b.p.); PCR – the PCR product with specific MDH primers. B – relative levels of transcripts of mdh gene in different cultivation conditions determined by RT-PCR.
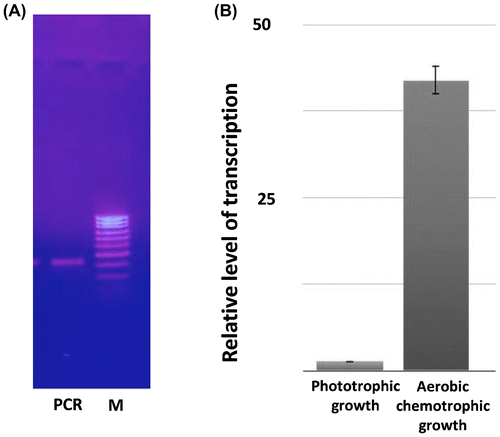
The RT-PCR data showed multifold increase of expression level of the malate dehydrogenase gene during the chemotrophic aerobic growth of Rhodovulum steppense as compared to the mdh level of these bacteria grown anaerobically in the light (Figure (B)).
Mass spectrometric analysis of malate dehydrogenase
Peptide mass spectrometric analysis of all three forms of MDH revealed the presence of same m/z peaks in each sample (Figure ). At least five masses of tryptic peptides can be identified with the m/z values of 602.3, 937.7, 1075.6, 1119.5, and 1246.5 in all three preparations. The results indicate the presence of the identical sets of masses corresponding to each MDH isoform from R. steppense.
Glyoxylate cycle and oxidative stress in aerobically grown bacteria
In the aerobically dark-grown bacteria, the activity of isocitrate lyase was detected (Figure (A)). Its appearance was prevented by the application of isocitrate lyase inhibitor itaconate (Figure (B)). Isocitrate lyase activity was not detectable during the anaerobic growth in the light. Itaconate also prevented the appearance of the form MDH3 during the chemotrophic cultivation of Rhodovulum steppense (Figure (C)). The transition to aerobic heterotrophic growth resulted in the rise of activities of catalase and SOD (Figure (A) and (B)).
Figure 5. Isocitrate lyase activity and appearance of the oligomeric form of MDH participating in the glyoxylate cycle in Rhodоvulum steppense. A – development of isocitrate lyase activity after transferring of bacteria to aerobic cultivation in darkness; B – specific staining of isocitrate lyase on zymograms; C – specific staining of MDH forms: a – chemotrophic aerobic growth; b – chemotrophic aerobic growth in the presence of itaconate; c – phototrophic growth. ICL – isocitrate lyase; F – band of bromophenol blue.
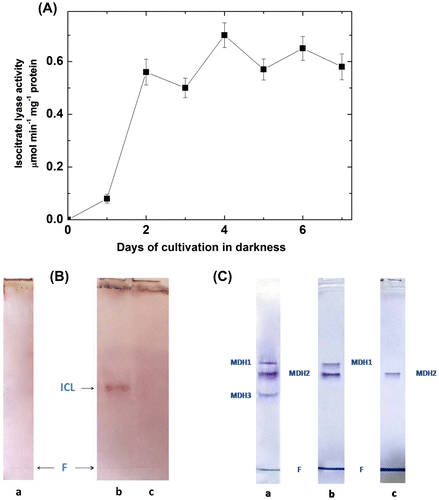
Discussion
The bacterium Rhodovulum steppense A-20s can grow litoautotrophically in the light and organoheterotrophically in darkness. While the main energetic process in these bacteria is photosynthesis, the microaerobic conditions are most optimal for their growth [Citation14]. The transition to aerobic conditions and darkness suppresses their growth and reproduction. In these stress conditions the bacterial metabolism should be reorganized to provide alternative nutrition and defence from the elevated oxygen concentrations.
The existence of MDH in different oligomeric forms was demonstrated for many organisms [Citation27–30]. In the bacteria Chloroflexus aurantiacus, Rhodobacter capsulatus, Rhodospirillum rubrum и Rhodomicrobium vannielii MDH exists in a tetrameric form [Citation31,32]. The multimeric structure of MDH was reported in the numerous investigations [Citation33–39]. When observed, monomers of MDH are usually inactive because the correct geometry of the catalytic site requires the formation of a stabilizing interface between two adjacent monomers. Although dimeric and tetrameric forms are common, the octameric structure is less usual and can be formed by the aggregation of two tetramers. The dimeric and tetrameric structures were attributed to the TCA and glyoxylate cycle in Beggiatoa leptomitiformis [Citation9] and Rhodopseudomonas palustris [Citation12].
Malate dehydrogenase genes are separated into two homologous groups. In eukaryotic organisms, the cytosolic and mitochondrial clade, all the enzymes are dimeric. They cannot form tetramers because of a specific sequence insertion that codes for a secondary structural element that prevents the dimerization of the dimeric state. The prokaryotic MDH group is closely related to the lactate dehydrogenase structure existing primarily in the tetrameric form. However, a structural flexibility is observed resulting in the formation of dimeric and monomeric structures [Citation39]. The analysis of the results obtained using specific primers for identification of the MDH gene as well as the database data retrieved from BLAST unequivocally demonstrate that the genome of Rhodovulum steppense contains only one MDH gene. In the annotated genome of Rhodovulum sulfidofilum, which is the closest relative of Rhodovulum steppense, the only one unique gene encoding the subunit of MDH is present. Peptide mass fingerprint analysis of MS spectra (Figure ) shows the presence of several tryptic peptides identical to the enzyme from the close species R. sulfidophilum which confirms that indeed all three oligomeric forms are formed as the product of a single MDH gene.
In the previous study MDH of Rhodovulum steppense A-20s was investigated under the conditions of photoorganotrophic anaerobic growth and its participation in the TCA cycle was suggested [Citation13]. Here we demonstrate the appearance of additional MDH forms during the chemotrophic aerobic growth, and characterize their oligomeric structure, properties and functional roles (Figure ). The chemotrophic growth on acetate leads to the induction of the glyoxylate cycle which is indicated by the increase of isocitrate lyase activity, the aerobic chemotrophic growth also leads to the development of the oxidative stress as confirmed by the multifold increase of the activities of catalase and SOD (Figure ). In these conditions the oligomeric forms MDH1 and MDH3 appear, one of which (dimer) is attributed to the glyoxylate cycle because it becomes absent when isocitrate lyase is inhibited by itaconate.
The observed properties of oligomers are in the correspondence with their functional role. The tetramer MDH2 participating in the TCA cycle has a lower pH and higher (with OAA) temperature optimum, higher affinities to OAA and NADH and lower affinities to malate and NAD+, thus establishing the important redox equilibrium for operation of the TCA cycle [Citation3,4]. It reveals the highest level of inhibition by OAA and citrate as compared to other forms (Table ). The dimeric form (MDH3) disappearing during the growth on the medium containing isocitrate lyase inhibitor itaconate and thus participating in the glyoxylate cycle (Figure ), has a slightly higher pH optimum, low inhibition by citrate, markedly higher affinities to malate and NAD+ and lower to OAA and NADH as seen from the ratios of Km values in both directions (Table ), which is more favorable for the reaction of OAA formation. The octamer MDH1 exhibits the highest affinity to malate and NAD+ among all oligomeric forms, lowest inhibition by OAA and citrate, which makes it most tuned for the reactions driven by malate accumulation in the aerobic heterotrophic conditions. This form has the unique properties in terms of maximum favourability of the equilibrium towards OAA formation. OAA protects cell compounds against oxidative damage [Citation40]. MDH3 disappeared in the cultures grown chemotrophically but anaerobically (Figure (A)). It may also participate in the reactions of aspartate formation at the absence of photosynthetic products as osmolytes in this halophilic bacterium.
Under chemotrophic stress conditions, Rhodovulum steppense shifts to the associative-dissociative mechanism of formation of MDH oligomers. This mechanism is reflected in a multiple increase of the expression level of the MDH gene during chemotrophic aerobic growth, and the enzyme is detected as a dimer, tetramer and octamer as compared to the mdh level of these bacteria grown anaerobically in the light when the enzyme is present as a single form. The formation of oligomeric proteins depends on many factors, including temperature, ionic strength, and pH value. In the studied conditions the ionic strength may have a major contribution because its value changes during the transition from the phototrophic to chemotrophic growth, however it may not necessarily act directly but can also trigger specific protein modifications. In the light, the products of photosynthesis contribute to the increase of ionic strength facilitating the formation of a tetramer. In darkness, during the chemotrophic growth, the ionic strength could decrease promoting a dissociation of oligomers to dimers. We propose that the formation of octamers may have a different mechanism that includes the processes of aggregation with membranes and formation of vesicles containing MDH to achieve efficient scavenging of ROS. Since each oligomer might be integrated into metabolons in vivo, additional factors to ionic strength, including specific modifications on one form of oligomer, could be applied for the explanation of changes in oligomeric state.
In conclusion, MDH of the purple non-sulfur bacterium Rhodovulum steppense A-20s is present in different oligomeric forms depending on the cultivation conditions. All three forms are encoded by a single gene and assembled of the 35 kDa subunit. While the tetrameric form (MDH2) is present both during the phototrophic and chemotrophic growth and likely participates in the TCA cycle which operates in all cultivation conditions, the dimeric (MDH3) and octameric (MDH1) forms appear only during the chemotrophic growth. The disappearance of the dimeric MDH3 in the presence of itaconate in the cultivation medium indicates its participation in the glyoxylate cycle. The octameric MDH1 appearance is not prevented by itaconate. This form may participate in the anabolic processes not related directly to the glyoxylate cycle and induced by the presence of oxygen, such as osmolyte synthesis, aspartate formation and ROS protection.
Author contributions
ATE, MIF and AUI conceived and designed the experiments. MSL and MIF performed the experiments and analysed the experimental data. IYT performed the fingerprint analysis. ATE and AUI wrote the paper.
Disclosure statement
No potential conflict of interest was reported by the authors.
Funding
This work was supported by the Russian Science Foundation [grant number 14-14-00721] to ATE and AUI; the Natural Sciences and Engineering Research Council of Canada [grant number 355753/2013] to AUI.
Acknowledgment
The authors express sincere gratitude to Dr Elena I. Kompantseva from the Winogradsky Institute of Microbiology of the Russian Academy of Sciences for providing the strain A-20s of Rhodovulum steppense.
References
- Paul S, Bag SK, Das S, et al. Molecular signature of hypersaline adaptation: insights from genome and proteome composition of halophilic prokaryotes. Gen Biol. 2008;9(4):R70.10.1186/gb-2008-9-4-r70
- Graziano G, Merlino A. Molecular bases of protein halotolerance. Biochim Biophys Acta. 2014;1844(4):850–858.10.1016/j.bbapap.2014.02.018
- Igamberdiev AU, Lernmark U, Gardeström P. Activity of the mitochondrial pyruvate dehydrogenase complex in plants is stimulated in the presence of malate. Mitochondrion. 2014;19(B):184–190.
- Igamberdiev AU, Eprintsev AT. Organic acids: the pools of fixed carbon involved in redox regulation and energy balance in higher plants. Front Plant Sci. 2016;7:1042.
- Deutch CE. l-Malate dehydrogenase activity in the reductive arm of the incomplete citric acid cycle of Nitrosomonas europaea. Antonie van Leeuwenhoek. 2013;104(5):645–655.10.1007/s10482-013-9973-6
- Bartholomae M, Meyer FM, Commichau FM, et al. Complex formation between malate dehydrogenase and isocitrate dehydrogenase from Bacillus subtilis is regulated by tricarboxylic acid cycle metabolites. FEBS J. 2014;281(4):1132–1143.10.1111/febs.12679
- Gharib G, Rashid N, Bashir Q, et al. Pcal_1699, an extremely thermostable malate dehydrogenase from hyperthermophilic archaeon Pyrobaculum calidifontis. Extremophiles. 2016;20(1):57–67.10.1007/s00792-015-0797-3
- Eprintsev AT, Falaleeva MI, Stepanova IY, et al. Purification and physicochemical properties of malate dehydrogenase from bacteria of the genus Beggiatoa. Biochemistry-Moscow. 2003;68(2):172–176.10.1023/A:1022693211134
- Eprintsev AT, Falaleeva MI, Grabovich MY, et al. Role of malate dehydrogenase isoforms in the regulation of anabolic and catabolic processes in the colorless sulfur bacterium Beggiatoa leptomitiformis D-402. Mikrobiologia. 2004;73(4):437–442.
- Eprintsev AT, Falaleeva MI, Parfyonova NV. Malate dehydrogenase from the thermophilic bacterium Vulcanithermus medioatlanticus. Biochemistry-Moscow. 2005;70(9):1027–1030.10.1007/s10541-005-0220-2
- Eprintsev AT, Falaleeva MI, Klimova MA, et al. Isolation and properties of malate dehydrogenase from meso- and thermophilic bacteria. Appl Biochem Microbiol. 2006;42(3):274–278.
- Eprintsev AT, Klimova MA, Shikhalieva KD, et al. Features of structural organization and expression regulation of malate dehydrogenase isoforms from Rhodobacter sphaeroides strain 2R. Biochemistry-Moscow. 2009;74(7):793–799.10.1134/S000629790907013X
- Eprintsev AT, Falaleeva MI, Parfenova IV, et al. Physicochemical, catalytic, and regulatory properties of malate dehydrogenase from Rhodovulum steppense bacteria, strain A-20s. Biology Bulletin. 2014;41(6):486–492.10.1134/S1062359014050033
- Kompantseva EI, Komova AV, Kostrikina NA. Rhodovulum steppense sp. nov., an obligately haloalkaliphilic purple nonsulfur bacterium widespread in saline soda lakes of Central Asia. Int J Syst Evol Microbiol. 2010;60(5):1210–1214.10.1099/ijs.0.014639-0
- Dixon M, Webb EC. Enzymes. 2nd ed. New York: Academic Press; 1964.
- Leach AA, O’Shea PC. The determination of protein molecular weights of up to 225,000 by gel-filtration on a single column of Sephadex G-200 at 25° and 40°. J Chromatogr. 1965;17:245–251.10.1016/S0021-9673(00)99864-9
- Shevchenko A, Wilm M, Vorm O, et al. Mass spectrometric sequencing of proteins from silver-stained polyacrylamide gels. Anal Chem. 1996;68(5):850–858.10.1021/ac950914 h
- Davis BJ. Disc electrophoresis. Method and application to human serum proteins. Ann NY Acad Sci. 1964;121:404–427.
- Worsfold M, Marshall MJ, Ellis EB. Enzyme detection using phenazine methosulfate and tetrazolium salts – interference by oxygen. Anal Biochem. 1977;79(1–2):152–156.10.1016/0003-2697(77)90389-X
- Heeb MJ, Gabriel O. Enzyme localization in gels. Methods Enzymol. 1984;104:416–439.10.1016/S0076-6879(84)04109-4
- Kornberg HL, Krebs HA. Synthesis of cell constituents from C2-units by a modified tricarboxylic acid cycle. Nature. 1957;179(4568):988–991.10.1038/179988a0
- Aebi H. Catalase in vitro. Methods Enzymol. 1984;105:121–126.10.1016/S0076-6879(84)05016-3
- Beauchamp C, Fridovich I. Superoxide dismutase: improved assays and an assay applicable to acrylamide gels. Analytical Biochemistry. 1971;44(1):276–287.10.1016/0003-2697(71)90370-8
- Lowry OH, Rosebrough NJ, Farr AL, et al. Protein measurement with the Folin phenol reagent. J Biol Chem. 1951;193(1):265–275.
- Reeves HC, Volk MJ. Determination of isocitrate lyase activity in polyacrylamide gels. Anal Biochem. 1972;48(2):437–441.10.1016/0003-2697(72)90096-6
- Livak KJ, Schmittgen TD. Analysis of relative gene expression data using real-time quantitative PCR and the 2−ΔΔCT method. Methods. 2001;25(4):402–408.10.1006/meth.2001.1262
- Shore JD, Chakrabarti SK. Subunit dissociation of mitochondrial malate dehydrogenase. Biochemistry. 1976;15(4):875–879.10.1021/bi00649a023
- Bleile DM, Schulz RA, Harrison JH, et al. Investigation of the subunit interactions in malate dehydrogenase. J Biol Chem. 1977;252(2):755–758.
- Sánchez SA, Hazlett TL, Brunet JE, et al. Aggregation states of mitochondrial malate dehydrogenase. Protein Sci. 1998;7(10):2184–2189.10.1002/pro.v7:10
- Rozova ON, Khmelenina VN, Bocharova KA, et al. Role of NAD⁺-dependent malate dehydrogenase in the metabolism of Methylomicrobium alcaliphilum 20Z and Methylosinus trichosporium OB3b. Microorganisms. 2015;3(1):47–59.10.3390/microorganisms3010047
- Bjørk A, Dalhus B, Mantzilas D, et al. Large improvement in the thermal stability of a tetrameric malate dehydrogenase by single point mutations at the Dimer–Dimer interface. J Mol Biol. 2004;341(5):1215–1226.10.1016/j.jmb.2004.06.079
- Tayeh MA, Madigan MT. Malate dehydrogenases in phototrophic purple bacteria. Biochem J. 1988;252(2):595–600.10.1042/bj2520595
- Hunter GR, Hellman U, Cazzulo JJ, et al. Tetrameric and dimeric malate dehydrogenase isoenzymes in Trypanosoma cruzi epimastigotes. Mol Biochem Parasitol. 2000;105(2):203–214.10.1016/S0166-6851(99)00176-0
- Madern D, Ebel C, Mevarech M, et al. Insights into the molecular relationships between malate and lactate dehydrogenases: structural and biochemical properties of monomeric and dimeric intermediates of a mutant of tetrameric L-[LDH-like] malate dehydrogenase from the halophilic archaeon Haloarcula marismortui. Biochemistry. 2000;39(5):1001–1010.10.1021/bi9910023
- Popov VN, Volvenkin SV, Kosmatykh TA, et al. Induction of a peroxisomal malate dehydrogenase isoform in liver of starved rats. Biochemistry-Moscow. 2001;66(5):496–501.10.1023/A:1010298516534
- Tripathi AK, Desai PV, Pradhan A, et al. An α-proteobacterial type malate dehydrogenase may complement LDH function in Plasmodium falciparum. Eur J Biochem. 2004;271(17):3488–3502.10.1111/ejb.2004.271.issue-17
- Fujii T, Oikawa T, Muraoka I, et al. Crystallization and preliminary X-ray diffraction studies of tetrameric malate dehydrogenase from the novel Antarctic psychrophile Flavobacterium frigidimaris KUC-1. Acta Crystallogr Sect F Struct Biol Cryst Commun. 2007;63(11):983–986.10.1107/S1744309107051524
- Muccio C, Guida V, Di Petrillo A, et al. A novel malate dehydrogenase from Ceratonia siliqua L. Seeds with potential biotechnological applications. Protein J. 2012;31(8):667–673.10.1007/s10930-012-9446-1
- Takahashi-Íñiguez T, Aburto-Rodríguez N, Vilchis-González AL, et al. Function, kinetic properties, crystallization, and regulation of microbial malate dehydrogenase. J Zhejiang Univ – Sci B. 2016;17(4):247–261.
- Oh TJ, Kim IG, Park SY, et al. NAD-dependent malate dehydrogenase protects against oxidative damage in Escherichia coli K-12 through the action of oxaloacetate. Environ Toxicol Pharmacol. 2002;11(1):9–14.10.1016/S1382-6689(01)00093-X