Abstract
Tetrathionate hydrolase (4THase), a key enzyme of the S4-intermediate (S4I) pathway, was partially purified from marine acidophilic bacterium, Acidithiobacillus thiooxidans strain SH, and the gene encoding this enzyme (SH-tth) was identified. SH-Tth is a homodimer with a molecular mass of 97 ± 3 kDa, and contains a subunit 52 kDa in size. Enzyme activity was stimulated in the presence of 1 M NaCl, and showed the maximum at pH 3.0. Although 4THases from A. thiooxidans and the closely related Acidithiobacillus caldus strain have been reported to be periplasmic enzymes, SH-Tth seems to be localized on the outer membrane of the cell, and acts as a peripheral protein. Furthermore, both 4THase activity and SH-Tth proteins were detected in sulfur-grown cells of strain SH. These results suggested that SH-Tth is involved in elemental sulfur-oxidation, which is distinct from sulfur-oxidation in other sulfur-oxidizing strains such as A. thiooxidans and A. caldus.
SH-Tth from the marine bacterium exhibited halophilic features distinct from those of the limnetic Af-Tth.
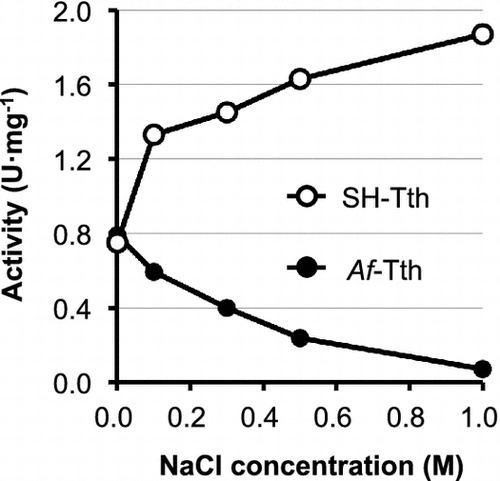
The biological oxidation of reduced inorganic sulfur compounds (RISCs) is one of the most important processes for both the global sulfur cycle [Citation1–3] and industrial biomining [Citation4,5]. Many studies on biological oxidation of RISCs have been published [Citation1,2,6,7]. At least two major pathways for RISCs oxidation have been suggested in various RISCs-oxidizing microorganisms [Citation1,2]. As the sulfur-oxidizing (Sox) system is the most common pathway for RISCs oxidation, this system has been extensively investigated in various RISCs oxidizers, including the neutrophilic chemolithotrophic bacterium Paracoccus pantotrophus [Citation8], the phototrophic purple and green bacteria, Allochromatium vinosum [Citation9], and Chlorobaculum tepidum [Citation10].
In addition, the S4 intermediate (S4I) pathway has also been reported in neutrophilic/acidophilic sulfur-oxidizing microorganisms [Citation11–13]. Tetrathionate (S4O62−), which is composed of four sulfur atoms and six oxygen atoms, is a key intermediate in this pathway. It has been suggested that tetrathionate is generated from the oxidation of two thiosulfate (S2O32−) molecules; this reaction can be catalyzed by thiosulfate oxidoreductases such as thiosulfate dehydrogenase (Tsd) [Citation14] and thiosulfate:quinone oxidoreductase (TQO) [Citation12,15]. Furthermore, tetrathionate can be utilized as the sole growth substrate for acidophilic sulfur-oxidizers, and is metabolized by tetrathionate hydrolase (4THase) [Citation16]. Indeed, 4THases are abundantly expressed in acidophilic chemolithotrophic sulfur-oxidizing bacteria such as Acidithiobacillus caldus [Citation17] and Acidithiobacillus ferrooxidans [Citation18] when grown on tetrathionate. This enzyme is one of the unique enzymes in the S4I pathway, and catalyzes the hydrolysis of tetrathionate to produce elemental sulfur, thiosulfate, and sulfate [Citation18,19]. Disruption and knockout experiments of the gene encoding 4THase in A. caldus [Citation20] and A. ferrooxidans [Citation21], respectively, have been conducted. It has been shown that 4THase-deficient mutants in both Acidithiobacillus species are unable to utilize tetrathionate as the sole growth substrate [Citation20,21], suggesting that tetrathionate needs to be metabolized by 4THase, and that 4THase is a key enzyme in the S4I pathway in these acidophiles.
We have isolated an autotrophic and acidophilic sulfur-oxidizing bacterium, Acidithiobacillus thiooxidans strain SH, from the marine environment [Citation22]. This strain is distinct from the limnetic A. thiooxidans type strain, and exhibits optimal growth in the presence of 0.34 M (2%) NaCl. Previous studies have shown that sulfur- and sulfite-oxidation activities can be stimulated with NaCl in resting cells [Citation23]. Similarly, our results also indicated that strain SH is able to grow on tetrathionate medium; 4THase activity was detected in both resting cells and cell-free extracts. To date, 4THases from A. ferrooxidans (Af-Tth) [Citation18], A. caldus (Ac-TetH) [Citation17,24], and the thermoacidophilic archaeon Acidianus ambivalens (Ad-TTH) [Citation25] have been isolated, and both biochemical characterizations and molecular biological (genetic) investigations have been carried out. Whole genome sequencing and transcriptional analyses were also performed to study sulfur oxidation in A. thiooxidans. The gene homologues associated with both RISCs oxidation pathways (Sox system and S4I pathway) were detected in the genome of A. thiooxidans type strain [Citation26], suggesting that this bacterium utilizes both pathways for RISCs oxidation. However, little is known with respect to RISCs oxidation from marine acidophilic sulfur-oxidizers. Since the ocean covers more than 70% of the surface on earth, the activities of marine sulfur-oxidizers are affected by the global sulfur cycle. In addition, halophilic acidophilic sulfur-oxidizing bacteria may be useful for bio-remediation in salt-containing environments contaminated with heavy metals, and can also be utilized for biomining in high salt environments.
Here, we characterized the key S4I pathway enzyme, 4THase, in strain SH of the marine acidophilic A. thiooxidans. Biochemical and molecular biological investigations of 4THase isolated from the bacterium were performed, and its properties were compared with those of other limnetic Acidithiobacillus species. This enzyme displayed halophilic properties distinct from those of limnetic 4THases. Furthermore, the SH strain seemed to be able to utilize both the S4I pathway and the Sox system for elemental sulfur oxidation, which is different from the closely related limnetic A. thiooxidans type strain.
Materials and methods
Bacterial strains, plasmids, and growth media
A. thiooxidans strain SH was aerobically grown on basal salts medium (in grams per liter: Na2SO4, 3.2; (NH4)2SO4, 3.0; MgSO4 7H2O, 0.5; KCl, 0.1; KH2PO4, 0.05; Ca(NO3)2, 0.01 adjusted to pH 4.0 with sulfuric acid) containing 0.34 M NaCl and energy substrates (5 mM K2S4O6, 10 mM K2S2O3 or 0.1% elemental sulfur) and the following trace elements (in milligram per liter: MnSO4 7H2O, 4.0; H3BO3, 3.0; Na2MoO4 2H2O, 2.0; FeSO4 7H2O, 2.0; KI, 1.0; CuSO4 5H2O, 0.4). The culture was maintained under aerobic condition at 30 °C. Cloning vector pUC18 and host cells Escherichia coli DH5α (Invitrogen, Carlsbad, CA, USA) were used for DNA manipulation. Cells were grown in Luria-Bertani (LB) medium supplemented with ampicillin (50 μg·ml−1).
Partial purification of 4THase from strain SH
Following a 10 day cultivation period in basal salt medium containing 5 mM potassium tetrathionate and 0.34 M NaCl, A. thiooxidans strain SH cells were harvested by centrifugation at 8000×g for 10 min. Cells were washed three times, suspended in 50 mM potassium phosphate buffer (KPB) (pH 7.0), and were disrupted by three passages through a French pressure cell press at 1500 kg cm−2. The solution was centrifuged at 10,000×g for 10 min to remove cellular debris and undisrupted cells. The supernatant was designated as the cell-free extract. Insoluble (total membrane) and soluble (cytosolic/periplasmic) fractions were separated from the cell-free extract by ultra-centrifugation at 60,000×g for 60 min. Ammonium sulfate was added to the soluble fraction to 30% saturation. This solution was centrifuged at 10,000×g for 10 min, and the supernatant was transferred to hydrophobic interaction columns (TOYOPEARL Butyl-650M, Tosoh, Tokyo, Japan) equilibrated with 50 mM KPB containing (NH4)2SO4 at 30% saturation. The 4THase enzyme was eluted with a linear gradient of 30–0% (NH4)2SO4 in the same buffer. The purity of active fractions in each purification step was assessed by sodium dodecyl sulfate-polyacrylamide gel electrophoresis (SDS-PAGE). Protein concentrations were determined by the Bio-Rad Protein Assay system (Bio-Rad, Hercules, CA, USA) with bovine serum albumin as the standard.
Fractionation of cell-free extracts
Membrane extraction treatment was carried out using cell-free extracts. After total membrane fraction was obtained via ultra-centrifugation, the precipitate (total membrane fraction) was homogeneously suspended in 50 mM KPB (pH 7.0) at 4 °C. The suspension was ultra-centrifuged at 60,000×g for 60 min to separate the soluble (membrane extracts) fraction from the insoluble (total membrane) fraction. The precipitate was suspended in the same buffer, and was ultra-centrifuged again under the same conditions. The supernatant was added to the first soluble fraction, and this mixture was designated as the membrane extract. The precipitate was suspended in the same buffer, and was designated as the total washed membrane fraction.
Isolation and cloning of the 4THase gene from strain SH
Following SDS-PAGE, the Coomassie Brilliant Blue (CBB)-stained protein band was excised from the gel, and was subjected to in-gel trypsin digestion. The digested protein samples were prepared using an In-Gel Tryptic Digestion Kit (Pierce, USA), and were analyzed by HPLC-Chip/QTOF (G6520 and G4240, Agilent Tech., USA). The internal amino acid sequences obtained from the treatment were analyzed with the MASCOT software (Matrix Science Inc., MA, USA). Two primers corresponding to the internal amino acid sequences were designed; SH52int-F (5′-GATGATATGTTTGCNTAYGC-3′, N: A/T/G/C, Y: C/T, R: A/G) was designed from DDMFAYA, and SH52int-R (5′-TTNGGNACRTGCCAGGTCCA-3′) was a complementary sequence of WTWHVPN. These primers were used to amplify the desired DNA fragments (0.8 kbp) from genomic DNA of strain SH. A plasmid clone carrying the gene encoding 4THase was screened from the genomic library by colony hybridization using the amplified DNA fragment as the probe. Nucleotide sequences of the 4THase gene were determined using the BigDye Terminator Cycle Sequencing Kit ver. 3.1 and the Model 3130 capillary DNA sequencer (Applied Biosystems, Foster City, CA, USA). In order to construct an expression vector for the 4THase gene, two primers were designed. The forward primer (SH-tth-NdeI-F: 5′-CAGCCCATATGCAAACCAATCAGGTGCC-3′) with a restriction enzyme site for NdeI (underlined) was designed from the expected N-terminal amino acid sequence of mature 4THase without the putative signal peptide sequence. The reverse primer (5′-ATTACGAATTCCTTTAAGCCTTGTGGGC-3′) with a restriction enzyme site for EcoRI (underlined) was a complementary sequence corresponding to the C-terminal region of the deduced amino acid sequence of the cloned gene. PCR was performed using the two primers, and the positive clone plasmid was used as the template. The amplified DNA fragments were purified using the DNA extraction kit. Purified DNA fragments were digested with NdeI and EcoRI, and the digested fragments were inserted into the corresponding cloning sites in the pET21a expression vector (Novagen, Madison, WI, USA). The constructed vector was designated as pETSH-tth.
Recombinant protein expression and refolding
The pETSH-tth expression plasmid was introduced into E. coli BL21 Star™(DE3) to obtain recombinant SH-Tth. E. coli BL21 Star™(DE3) harboring pETSH-tth was cultured in a modified Terrific broth (TB) medium supplemented with 50 μg·ml−1 ampicillin at 37 °C to an OD660 nm of 1.0. The expression of SH-tth in pETSH-tth was induced by the addition of 1 mM isopropyl-β-D-thiogalactopyranoside (IPTG), followed by incubation at 20 °C for 36 h. The recombinant protein was synthesized in inclusion bodies in its inactive form. The inclusion bodies were collected, and were washed three times with 100 mM potassium phosphate buffer (KPB) (pH 7.0) containing 4% (v/v) Triton X-100. They were then washed again with sterilized, distilled water to remove the detergent. The protocol for SH-Tth refolding to induce enzyme activation was the same as that used for Af-Tth, and has previously been described in detail [Citation27]. Soluble and active SH-Tth was obtained following the refolding procedure.
Western blot analysis
Cell extracts containing 50 μg of total cell protein or fractionated cell proteins by ultra-centrifugation were separated by 12.5% SDS-PAGE. In order to compare total proteins and specific protein, SDS-PAGE was performed in duplicate. One SDS-polyacrylamide gel was stained by CBB to detect total proteins in each lane. For Western blot analysis, proteins were transferred from the counterpart gel to polyvinylidene difluoride (PVDF) membranes (Millipore, Bedford, MA, USA). Polyclonal rabbit antibodies against SH-Tth polypeptides were diluted 500-fold, and were used to detect SH-Tth in the samples. Protein A-peroxidase conjugate (Bio-Rad) was used to visualize specific protein bands, together with 4-chloro-1-naphtol and hydrogen peroxide.
Measurement of enzyme activity
SH-Tth activity was measured as follows. The reaction mixture (1 mL) contained 1.5 mM K2S4O6, 40 mM K2SO4, 0.4 M NaCl, and 50 mM β-alanine nitrate buffer (pH 3.0); the reaction was initiated by the addition of 50 μL enzyme solution at 30 °C. During the reaction, 110 μL reaction mixture was removed and placed into small thin-wall tubes (0.2 mL PCR tube) at 60 min intervals, and was immediately heated at 98 °C for 5 min to terminate the reaction. Samples were then placed on ice. Since elemental sulfur was also generated during the process, samples were centrifuged at 8000×g for 3 min to remove the sulfur particles. The remaining tetrathionate concentrations were measured in 0.1 mL of the supernatant by cyanolysis. One unit (U) of activity was defined as the amount of enzyme required for hydrolysis of 1 μmol tetrathionate per min.
Results
Partial purification of 4THase from A. thiooxidans strain SH
4THase activity was detected in strain SH cell-free extracts from tetrathionate-grown cells (0.035 ± 0.006 U mg−1). After cell-free extracts were obtained, the protein solution was ultra-centrifuged (60,000×g) to separate the cytosolic/periplasmic (soluble) fraction from the total membrane (precipitate) fraction. 4THase activity was detected in both fractions (soluble fraction; 0.011 ± 0.004 U mg−1, total membrane fraction; 0.067 ± 0.018 U mg−1). While higher specific 4THase activity was detected in the membrane fraction, for convenience, we chose the soluble fraction for enzyme purification. The soluble fraction was applied to hydrophobic chromatography columns following ammonium sulfate precipitation treatment (30% saturation). The purification profiles are shown in Table S1. In order to assess enzyme purity in each step, active fractions were subjected to SDS-PAGE. SDS-PAGE analysis of the active fraction in the TOYOPEARL Butyl-650M (hydrophobic interaction chromatography) step yielded three major protein bands with molecular masses of 52, 35, and 16 kDa (Figure , lane 3). The internal amino acid sequence of the protein was analyzed by the in-gel trypsin digestion method using HPLC-Chip/QTOF. Amino acid sequences for four peptide fragments in the 52 kDa protein were identified (Table S2). All four peptides were shown to be top hits against 4THase from the NCBI database using BLASTP. In addition, MASCOT software analysis demonstrated that 4THase sequences from Acidithiobacillus ferrivorans and A. thiooxidans exhibited the greatest similarity to those of the isolated peptides.
Figure 1. SDS-PAGE of 4THase from Acidithiobacillus thiooxidans strain SH. The active fractions of each purification step were subjected to SDS-PAGE. Lane 1, soluble fraction after ultra-centrifugation; lane 2, supernatant of 30% saturated ammonium sulfate; lane 3, TOYOPEARL Butyl-650M hydrophobic column chromatography; lane M, molecular mass marker. The molecular sizes in kDa are indicated on the left. The 52 kDa protein band expected for SH-Tth is indicated by the arrow.
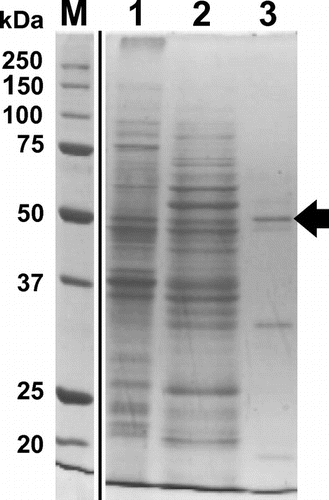
Isolation of the gene encoding 4THase from strain SH
In order to obtain the gene encoding 4THase in strain SH, two oligonucleotide primers were designed based on the internal peptide sequences of the 52 kDa protein. PCR was carried out with A. thiooxidans SH genomic DNA, which yielded fragments with lengths of approximately 800 bp. The DNA sequence of the PCR products showed high similarity to 4THase genes from another Acidithiobacillus species such as A. thiooxidans ATCC19377 and A. caldus strain KU (ATCC51756). Using the 800 bp DNA as a probe, the PstI-EcoRI fragment (1489 bp) was isolated from the SH genome clone plasmid library by colony hybridization (Figure S1). Because PstI recognition sequence (CTGCAG) was present in the isolated gene, about 30 bp from start codon seemed to be truncated in the gene (Figures and S1). However, the N-terminus region of the encoded protein was considered to be the signal peptide, and therefor the isolated gene fragment encoded the full length mature protein. The deduced amino acid sequence of the gene without the putative signal peptide (1443 bp) consisted of 480 amino acids, and showed the highest similarity to putative 4THases from A. thiooxidans ATCC19377 (93% identical), TetH [Citation17] from A. caldus (76% identical), and Tth [Citation18] from A. ferrooxidans (57% identical). Therefore, this gene was designated as SH-tth. All four peptides of the trypsin-digested 52 kDa protein were observed in the deduced amino acid sequence of SH-Tth (Figure S1).
Figure 2. Alignment of deduced amino acid sequence of 4THases. Putative signal peptides are indicated in bold. Asterisks indicate conserved amino acid residues between the four 4THases, and colons and periods indicate strongly and weakly similar residues between the sequences, respectively. SH-Tth: 4THase from A. thiooxidans strain SH; At-Tth: 4THase from A. thiooxidans type strain; Ac-TetH: 4THase from A. caldus; Af-Tth: 4THase from A. ferrooxidans.
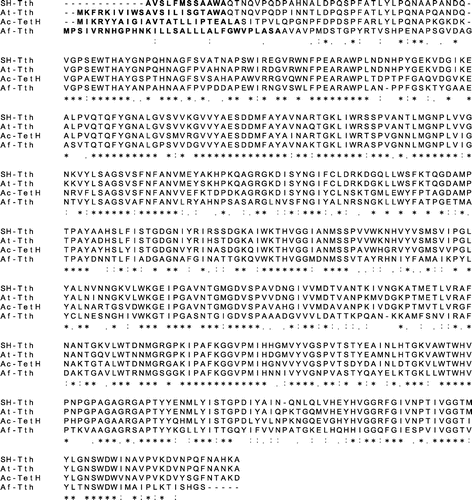
Recombinant expression of SH-tth and refolding
As mentioned in the Materials and Methods section, the expression vector, pETSH-tth, was constructed and introduced into E. coli BL21 Star™(DE3). The recombinant gene product (SH-Tth) was synthesized in inclusion bodies in its inactive form, similar to recombinant Af-Tth (Tth from A. ferrooxidans). We have already optimized a condition to obtain recombinant Af-Tth in its active form following acidic refolding treatment [Citation27]. SH-Tth was solubilized, and exhibited 4THase activity (1.45 U mg−1) following the same refolding treatment. SDS-PAGE analysis did not reveal the presence of other protein bands aside from that of SH-Tth (52 kDa) in the soluble fraction (Figure S2).
Characterization of recombinant SH-Tth
The subunit molecular mass of SH-Tth was estimated to be 52 kDa by SDS-PAGE and the deduced amino acid sequence. In order to determine the native molecular mass of SH-Tth, the refolded and purified enzyme was subjected to gel-permeation column chromatography. Two protein peaks were observed, which corresponded to 97 ± 3 kDa and 56 ± 8 kDa (Figure S3(A) and (B)); similar levels of 4THase activity were detected in each peak fraction. These results suggested that SH-Tth coexists as both monomers and homodimers in the soluble enzyme solution.
The optimal temperature for SH-Tth activity was found to be 55 °C; activity drastically decreased at temperatures above 65 °C (Figure (A)). In addition, SH-Tth activity was detected at a pH range of 1.0–5.5, and reached maximum value at pH 3.0 (Figure (B)). SH-Tth activity was increased by 2.5 times in the presence of 1 M NaCl (Figure ). KCl (2 M) also increased SH-Tth activity by 2.5-fold. Furthermore, in the presence of 1 M Na2SO4 and (NH4)2SO4, a 2.5 and 4-fold enhancements in enzyme activity were observed in comparison with the absence of Na2SO4 and (NH4)2SO4, respectively. However, enzyme activity declined by 20 and 40% in the presence of 1 M NaNO3 and 1 M KNO3, respectively. On the other hand, more than 90% of Af-Tth activity from limnetic A. ferrooxidans was inhibited in the presence of 1 M NaCl (Figure ).
Figure 3. Effect of temperature (A) and pH (B) on recombinant SH-Tth. (A) SH-Tth in the reaction mixture without tetrathionate was incubated for 3 min at each temperature prior to assay of the activity. The reaction was initiated by the addition of tetrathionate solution. (B) Buffers used were as follows: pH 1.0–3.5, 50 mM β-alanine nitrate buffer (closed circle); pH 2.5–4.5, 50 mM formate buffer (open triangle); pH 3.5–5.5, 50 mM citrate buffer (closed triangle); pH 5.5–7.0, 50 mM potassium phosphate buffer (open circle).
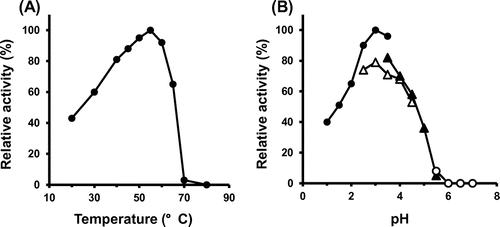
Localization of SH-Tth
4THases from limnetic A. thiooxidans ON107 and the closely related species A. caldus KU (type strain) have been reported as periplasmic enzymes [Citation24,28]. Ac-TetH was confirmed to be localized in the periplasm by differential fractionation, where enzymatic activities of glucose-6-phosphate dehydrogenase, acid phosphatase, and succinate dehydrogenase were detected in the cytoplasmic, periplasmic, and membrane fractions [Citation24]. Cell-free extracts from strain SH cells grown on tetrathionate were fractionated by ultra-centrifugation at 60,000×g for 60 min to yield both the precipitate (total membrane) and the supernatant (cytoplasm/periplasm). As mentioned above, SH-Tth activity was detected in both fractions. The membrane fraction was re-suspended in 50 mM KPB (pH 7.0), and the protein solution was ultra-centrifuged again. Interestingly, SH-Tth activity (0.018 ± 0.007 U·mg−1) was detected in the supernatant, suggesting that SH-Tth can be solubilized without any detergents. While most of the enzyme activity was observed in the soluble fraction, SH-Tth activity was still detected in the precipitate (0.051 ± 0.012 U·mg−1).
In order to confirm the existence of SH-Tth in each fraction, Western blots were performed using rabbit antisera against the recombinant SH-Tth. Binding of polyclonal antibodies to SH-Tth was clearly observed in all fractions, and again, SH-Tth activity was detected in both fractions (Figure ). These results suggested that SH-Tth was a membrane protein, but taking into account the solubility of this enzyme, SH-Tth was hypothesized to be a membrane associated (peripheral membrane) protein.
Figure 5. Detection of SH-Tth protein by Western blot. Cell-free extract was separated by ultra-centrifugation at 60,000×g for 60 min. Each fraction was loaded onto SDS-PAGE. Lane 1, supernatant (cytosolic/periplasmic fraction); lane 2, precipitate (total membrane fraction). Total membrane fraction (lane 2) was re-suspended and ultra-centrifuged again. Lane 3, supernatant following the second ultra-centrifugation step (membrane extracted proteins); Lane 4, precipitate following the second ultra-centrifugation step (washed total membrane fraction). Panel (A): SDS-PAGE gel stained by CBB. Panel (B): Western blot using polyclonal antibodies against recombinant SH-Tth. The procedures are described in Materials and methods section. Positive SH-Tth signals are indicated by the arrow. Lane M, molecular mass marker. Molecular masses are indicated on the left side of each panel.
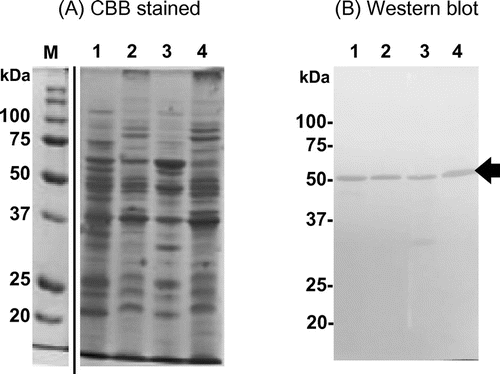
Synthesis of SH-Tth in cells grown on different substrates
A. thiooxidans strain SH can use elemental sulfur, thiosulfate, and tetrathionate as the sole energy source for growth. SH cells at the beginning of the stationary phase were harvested from cultures with different growth substrates. Total cell proteins (50 μg each) were separated by SDS-PAGE, followed by Western blot analysis with anti-SH-Tth polyclonal antibodies. A clear protein band was observed in tetrathionate-grown cells (Figure , lane TT); a faint band was detected in thiosulfate-grown cells (Figure , lane TS); a faint band was also detected in sulfur-grown cells (Figure , lane ES). Signal intensities of SH-Tth protein bands were comparable to the corresponding 4THase activity in cells grown on each substrate (Figure ). Whereas SH-Tth was synthesized in cells grown on all three substrates, 4THase activity could not be detected in sulfur-grown cells in the closely related A. caldus type strain [Citation17,24]. These results suggested that SH-Tth was not only involved in tetrathionate metabolism, but also in thiosulfate and elemental sulfur metabolism.
Figure 6. SH-Tth synthesis in cells grown on different growth substrates. Lane ES, total cell proteins from elemental sulfur-grown cells; lane TS, total cell proteins from thiosulfate-grown cells; lane TT, total cell proteins from tetrathionate-grown cells; lane M, molecular mass marker. Panel (A): SDS-PAGE gel stained by CBB. Panel (B): Western blot using polyclonal antibodies against recombinant SH-Tth. The procedures are described in Materials and methods section. Positive SH-Tth signals are indicated by the arrow. The specific activities (mU mg−1 protein) in the cell-free extract are indicated on the bottom of each lane. Molecular masses are indicated on the left side of each panel.
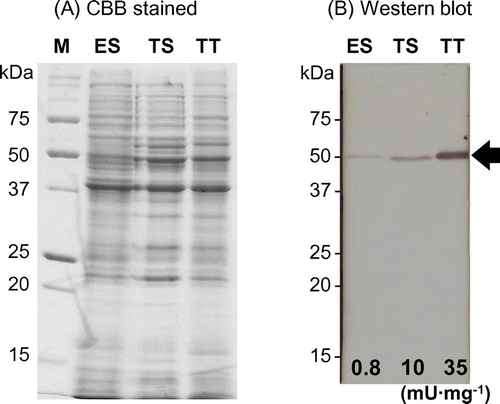
Discussion
We successfully obtained recombinant SH-Tth from E. coli cells in its active form via the acidic refolding treatment. The biochemical properties of SH-Tth from the marine bacterium exhibited halophilic features distinct from those of the limnetic Af-Tth (Figure ). The primary structure of SH-Tth, aside from the putative signal peptide, showed 57% identity (272/480 aa) to that of Af-Tth. The 43% differences in the residues between the two 4THases may be associated with enzyme sensitivity or activation in the presence of NaCl. Interestingly, only a 7% difference was observed in the primary structures between SH-Tth and At-Tth from A. thiooxidans type strain (ATCC19377). As it is not uncommon for a few substitutions in amino acid residues of an enzyme to substantially enhance its activity or stability, the 7% difference between the two 4THases may play a crucial role in enzyme activity during NaCl exposure. Further studies on 4THase isolated from limnetic A. thiooxidans should be conducted to investigate the effect of NaCl on 4THase activity.
As assessed by gel permeation chromatography, the recombinant SH-Tth was estimated to be a homodimer with a molecular mass of 97 ± 3 kDa. Its enzyme composition was found to be the same as that of other 4THases [Citation18,24,25,28]. However, the soluble recombinant SH-Tth obtained following the refolding treatment was easily dissociated into its monomer form (Figure S3(A) and (B)). This was similar to soluble Ad-TTH from Ad. ambivalens, which was also reported to display both the homodimer and monomer forms after being liberated from the S-layer or released from the pseudo-periplasm [Citation25].
SH-Tth was hypothesized to be loosely associated with the membrane. Af-Tth from the iron- and sulfur-oxidizing bacterium, A. ferrooxidans, has been suggested to be an outer membrane protein [Citation29], and can be liberated from the membrane without any detergents [Citation18]. Similar to SH-Tth, acidic refolding treatment was also able to solubilize and restore enzymatic activity of the recombinant Af-Tth. Although only a single peak corresponding to 100 kDa was observed in recombinant Af-Tth at pH 4.0 by gel-permeation chromatography, two peaks that corresponded to the homodimer and monomer forms were observed at neutral pH (unpublished data). This suggested that SH-Tth forms homodimeric structures when it is associated with the membrane. However, once the enzyme is liberated from the membrane, the subunits could dissociate from each other to yield monomers.
It has been reported that Ac-TetH from A. caldus is localized in the periplasmic space [Citation24]. In contrast, SH-Tth and Af-Tth were found to be localized on the outer membrane [Citation29]. Since it is interesting that even closely related species in Acidithiobacilli demonstrate diversity in terms of enzyme localization, immunocytochemical studies need to be performed to offer direct evidence that can determine the localization of various 4THases.
Western blot analysis revealed that synthesis of SH-Tth in cells is regulated by growth substrates. Differential synthesis (or expression levels) of 4THases (or genes) grown in different substrates have been reported in A. ferrooxidans [Citation18], A. caldus [Citation17], and Ad. ambivalens [Citation25]. We observed high synthesis of Af-Tth in tetrathionate-grown cells, but not in iron-grown cells. Transcriptional and protein analysis also demonstrated that synthesis of Ac-TetH was carried out in tetrathionate-grown cells, but not in sulfur-grown cells. Quantitative RT-PCR analysis showed that expression of Ac-tetH in tetrathionate-grown cells is enhanced by more than 200-fold as compared to that in sulfur-grown cells. Furthermore, in Ad. ambivalens, higher Ad-tth1 gene expression was detected in total RNA from tetrathionate-grown cells as compared to that from sulfur-grown cells via Northern blot. The putative two-component transcriptional regulator genes were located in regions adjacent to the Af-tth and Ac-tetH genes in A. ferrooxidans and A. caldus, respectively. Recently, stoichiometric modeling of RISC oxidation has been proposed in A. thiooxidans DSM17318 [Citation30]. According to the model, thiosulfate generated from elemental sulfur was mainly oxidized via the Sox system, and the flux associated with TTH was zero in sulfur-grown cells. In contrast, TTH plays a central role in tetrathionate-grown cells with no elemental sulfur production. Two sets of sox gene clusters have been detected in the genome of A. thiooxidans strains, ATCC19377 [Citation26], A01 [Citation31], and A. caldus type strain [Citation32]. In sulfur-grown cells, these three strains utilized Sox enzymes for elemental sulfur oxidation, and 4THase activity was repressed at the transcriptional level. However, both SH-Tth synthesis (detected by Western blot) (Figure , lane ES) and 4THase activity were detected in sulfur-grown SH cells. Even though strain SH belongs to A. thiooxidans species, the S4I pathway involving 4THases may be associated with elemental sulfur oxidation. We have previously reported on a novel tetrathionate producing thiosulfate dehydrogenase from strain SH [Citation15]; this protein or its homologue was not found in any other A. thiooxidans strains whose genomic sequences have been determined. This enzyme may play a role in supplying substrates for SH-Tth, and may generate reduced quinone to be utilized as a source of reducing power.
Our results provided the first characterization of 4THase from marine bacteria. SH-Tth displayed halophilic characteristics distinct from those of limnetic 4THases despite their similar primary structures. These results showcased the various properties of 4THases from different species. Furthermore, the detection of SH-Tth at both the protein and activity levels in sulfur-grown cells implied that strain SH utilizes a different sulfur-oxidation pathway from that of other closely related A. thiooxidans strains under the same growth condition. Detection and identification of the genes associated with RISC oxidation via SH whole genome sequencing, and comparison of these genes with other Acidithiobacillus species, will be helpful for revealing the sulfur-oxidation system in this marine bacterium. Whole genome sequencing and draft genome analyses of strain SH are currently in progress.
Authors contributions
TK and KK designed this research. MO, YK, YH, TT and HK performed the experiments. TK wrote the paper. TK and KK supervised all the experiments and data analysis.
Disclosure statement
No potential conflict of interest was reported by the authors.
Funding
This work was supported by the Japan Society for the Promotion of Science (JSPS) [KAKEN grant number 17K08169]; the Yakumo Foundation for Environmental Science.
Supplemental data
Supplemental data for this article can be accessed [https://doi.org/10.1080/09168451.2017.1415128].
Fig_S1-S3B_BBB_leg_Revise1.pdf
Download PDF (594.3 KB)Table_S1-2_SH-Tth__BBB.pdf
Download PDF (54.7 KB)Acknowledgement
We would like to thank Editage (www.editage.jp) for English language editing.
References
- Kelly DP, Shergill JK, Lu WP, Wood AP. Oxidative metabolism of inorganic sulfur compounds by bacteria. Antonie van Leeuwenhoek. 1997;71:95–107.10.1023/A:1000135707181
- Ghosh W, Dam B. Biochemistry and molecular biology of lithotrophic sulfur oxidation by taxonomically and ecologically diverse bacteria and archaea. FEMS Microbiol Rev. 2009;33:999–1043.10.1111/j.1574-6976.2009.00187.x
- Stewart FJ. Dissimilatory sulfur cycling in oxygen minimum zones: an emerging metagenomics perspective. Biochem Soc Trans. 2011;39:1859–1863.10.1042/BST20110708
- Rawlings DE. Characteristics and adaptability of iron- and sulfur-oxidizing microorganisms used for the recovery of metals from minerals and their concentrates. Microb Cell Fact. 2005;4: 13.10.1186/1475-2859-4-13
- Rawlings DE, Johnson DB. The microbiology of biomining: development and optimization of mineral-oxidizing microbial consortia. Microbiology. 2007;153:315–324.10.1099/mic.0.2006/001206-0
- Friedrich CG, Bardischewsky F, Rother D, Quentmeier A, Fischer J. Prokaryotic sulfur oxidation. Curr Opin Microbiol. 2005;8:253–259.10.1016/j.mib.2005.04.005
- Dopson M, Johnson DB. Biodiversity, metabolism and applications of acidophilic sulfur-metabolizing microorganisms. Environ Microbiol. 2012;14:2620–2631.10.1111/emi.2012.14.issue-10
- Friedrich CG, Rother D, Bardischewsky F, Quentmeier A, Fischer J. Oxidation of reduced inorganic sulfur compounds by bacteria: emergence of a common mechanism? Appl Environ Microbiol. 2001;67:2873–2882.10.1128/AEM.67.7.2873-2882.2001
- Hensen D, Sperling D, Truper HG, Brune DC, Dahl C. Thiosulphate oxidation in the phototrophic sulphur bacterium Allochromatium vinosum. Mol Microbiol. 2006;62:794–810.10.1111/mmi.2006.62.issue-3
- Azai C, Tsukatani Y, Harada J, Oh-oka H. Sulfur oxidation in mutants of the photosynthetic green sulfur bacterium Chlorobium tepidum devoid of cytochrome c-554 and SoxB. Photosynth Res. 2009;100:57–65.10.1007/s11120-009-9426-2
- Hallberg KB, Dopson M, Lindström EB. Reduced sulfur compound oxidation by Thiobacillus caldus. J Bacteriol. 1996;178:6–11.10.1128/jb.178.1.6-11.1996
- Müller FH, Bandeiras TM, Urich T, Teixeira M, Gomes CM, Kletzin A. Coupling of the pathway of sulphur oxidation to dioxygen reduction: characterization of a novel membrane-bound thiosulphate:quinone oxidoreductase. Mol Microbiol. 2004;53:1147–1160.10.1111/j.1365-2958.2004.04193.x
- Dam B, Mandal S, Ghosh W, Das Gupta SK, Roy P. The S4-intermediate pathway for the oxidation of thiosulfate by the chemolithoautotroph Tetrathiobacter kashmirensis and inhibition of tetrathionate oxidation by sulfite. Res Microbiol. 2007;158:330–338.10.1016/j.resmic.2006.12.013
- Kikumoto M, Nogami S, Kanao T, Takada J, Kamimura K. Tetrathionate-forming thiosulfate dehydrogenase from the acidophilic, chemolithoautotrophic bacterium Acidithiobacillus ferrooxidans. Appl Environ Microbiol. 2013;79:113–120.10.1128/AEM.02251-12
- Sharmin S, Yoshino E, Kanao T, Kamimura K. Characterization of a novel thiosulfate dehydrogenase from a marine acidophilic sulfur-oxidizing bacterium, Acidithiobacillus thiooxidans strain SH. Biosci Biotechnol Biochem. 2016;80:273–278.10.1080/09168451.2015.1088377
- Beard S, Paradela A, Albar JP, Jerez CA. Growth of Acidithiobacillus ferrooxidans ATCC 23270 in thiosulfate under oxygen-limiting conditions generates extracellular sulfur globules by means of a secreted tetrathionate hydrolase. Front Microbiol. 2011;2: 79.
- Rzhepishevska OI, Valdés J, Marcinkeviciene L, Gallardo CA, Meskys R, Bonnefoy V, Holmes DS, Dopson M. Regulation of a novel Acidithiobacillus caldus gene cluster involved in metabolism of reduced inorganic sulfur compounds. Appl Environ Microbiol. 2007;73:7367–7372.10.1128/AEM.01497-07
- Kanao T, Kamimura K, Sugio T. Identification of a gene encoding a tetrathionate hydrolase in Acidithiobacillus ferrooxidans. J Biotechnol. 2007;132:16–22.10.1016/j.jbiotec.2007.08.030
- de Jong GAH, Hazeu W, Bos P, Kuenen JG. Polythionate degradation by tetrathionate hydrolase of Thiobacillus ferrooxidans. Microbiology. 1997;143:499–504.10.1099/00221287-143-2-499
- van Zyl LJ, van Munster JM, Rawlings DE. Construction of arsB and tetH mutants of the sulfur-oxidizing bacterium Acidithiobacillus caldus by marker exchange. Appl Environ Microbiol. 2008;74:5686–5694.10.1128/AEM.01235-08
- Yu Y, Liu X, Wang H, Li X, Lin J. Construction and characterization of tetH overexpression and knockout strains of Acidithiobacillus ferrooxidans. J Bacteriol. 2014;196:2255–2264.10.1128/JB.01472-13
- Kamimura K, Higashino E, Moriya S, Sugio T. Marine acidophilic sulfur-oxidizing bacterium requiring salts for the oxidation of reduced inorganic sulfur compounds. Extremophiles. 2003;7:95–99.
- Kamimura K, Higashino E, Kanao T, Sugio T. Effects of inhibitors and NaCl on the oxidation of reduced inorganic sulfur compounds by a marine acidophilic, sulfur-oxidizing bacterium, Acidithiobacillus thiooxidans strain SH. Extremophiles. 2005;9:45–51.
- Bugaytsova Z, Lindström EB. Localization, purification and properties of a tetrathionate hydrolase from Acidithiobacillus caldus. Eur J Biochem. 2004;271:272–280.10.1046/j.1432-1033.2003.03926.x
- Protze J, Müller F, Lauber K, Nass B, Mentele R, Lottspeich F, et al. An extracellular tetrathionate hydrolase from the thermoacidophilic archaeon Acidianus ambivalens with an activity optimum at pH 1. Front Microbiol. 2011;2: 68.
- Valdés J, Ossandon F, Quatrini R, Dopson M, Holmes DS. Draft genome sequence of the extremely acidophilic biomining bacterium Acidithiobacillus thiooxidans ATCC 19377 provides insights into the evolution of the Acidithiobacillus genus. J Bacteriol. 2011;193:7003–7004.10.1128/JB.06281-11
- Kanao T, Matsumoto C, Shiraga K, Yoshida K, Takada J, Kamimura K. Recombinant tetrathionate hydrolase from Acidithiobacillus ferrooxidans requires exposure to acidic conditions for proper folding. FEMS Microbiol Lett. 2010;309:43–47.
- Tano T, Kitaguchi H, Harda M, Nagasawa T, Sugio T. Purification and some properties of a tetrathionate decomposing enzyme from Thiobacillus thiooxidans. Biosci Biotech Biochem. 1996;60:224–227.10.1271/bbb.60.224
- Buonfiglio V, Polidoro M, Soyer F, Valenti P, Shively J. A novel gene encoding a sulfur-regulated outer membrane protein in Thiobacillus ferrooxidans. J Biotechnol. 1999;72:85–93.10.1016/S0168-1656(99)00097-8
- Bobadilla Fazzini RA, Cortes MP, Padilla L, Maturana D, Budinich M, Maass A, Parada P. Stoichiometric modeling of oxidation of reduced inorganic sulfur compounds (Riscs) in Acidithiobacillus thiooxidans. Biotechnol Bioeng. 2013;110:2242–2251.10.1002/bit.v110.8
- Yin H, Zhang X, Liang Y, Xiao Y, Niu J, Liu X. Draft genome sequence of the extremophile Acidithiobacillus thiooxidans A01 isolated from the wastewater of a coal dump. Genome Announc. 2014;2:e00222–14
- Valdés J, Quatrini R, Hallberg K, Dopson M, Valenzuela PD, Holmes DS. Draft Genome Sequence of the Extremely Acidophilic Bacterium Acidithiobacillus caldus ATCC 51756 Reveals Metabolic Versatility in the Genus Acidithiobacillus. J Bacteriol. 2009;191:5877–5878.10.1128/JB.00843-09