ABSTRACT
Members of the cytosolic sulfotransferase (SULT) SULT2A subfamily are known to be critically involved in the homeostasis of steroids and bile acids. SULT2A8, a 7α-hydroxyl bile acid-preferring mouse SULT, has been identified as the major enzyme responsible for the mouse-specific 7-O-sulfation of bile acids. Interestingly, SULT2A8 lacks a conservative catalytic His residue at position 99th. The catalytic mechanism underlying the SULT2A8-mediated 7-O-sulfation of bile acids thus remained unclear. In this study, we performed a mutational analysis in order to gain insight into this yet-unresolved issue. Results obtained revealed two amino acid residues, His48 and Leu99, that are unique to the mouse SULT2A8, but not other SULTs, are essential for its 7-O-sulfating activity toward bile acids. These findings suggested that substitutions of two amino acids, which might have occurred during the evolution of the mouse SULT2A8 gene, endowed mouse SULT2A8 the capacity to catalyze the 7-O-sulfation of bile acids.
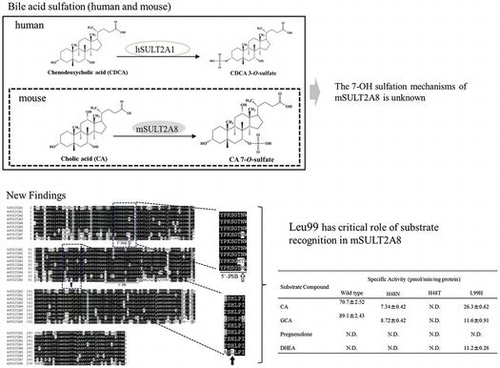
Mouse SULT2A8 can catalyze sulfation of 7α-hydroxyl bile acids, however the mechanism has been unknown. His48 has critical role of 7α-hydroxyl sulfation.
Cytosolic sulfotransferases (SULTs) are a group of enzymes that catalyze the transfer of a sulfonate group from the sulfate donor, 3′-phosphoadenosine 5′-phosphosulfate (PAPS), to various compounds containing hydroxyl or amino group [Citation1]. The SULTs have long been known to be involved in the homeostasis of key endogenous compounds such as steroid/thyroid hormones, catecholamines, and bile acids, as well as the biotransformation and elimination of xenobiotics and drugs, in mammals [Citation2–Citation4]. All SULTs constitute a gene superfamily and are categorized into distinct subfamilies based on the amino acid sequence identity [Citation5–Citation8]. Members of the SULT2A subfamily are known to catalyze the sulfation of steroids and bile acids[Citation2,9].
Bile acids are known to be synthesized from cholesterol in the liver and secreted into the duodenum, thereby facilitating the digestion and absorption of lipids [Citation10]. Studies have revealed that bile acids, by interacting with farnesoid X receptor (FXR), are also involved in the regulation of the expression of genes involved in the cholesterol, glucose, and bile acids homeostasis [Citation11–Citation13]. Importantly, bile acids have been linked to certain pathological effects, including liver toxicity and carcinogenicity [Citation14,15]. Sulfation of bile acids has been known to play an important role in the detoxification and excretion of bile acids [Citation16]. Since sulfation may inactivate the ligand activity, increase the water-solubility, and facilitate the excretion of bile acids, it plays a critical role in regulating the homeostasis of bile acids [Citation16]. Sulfation of bile acids occurs at the position of 3α-hydroxyl group bile acids in humans [Citation17]. Unlike humans, however, only bile acid-7-O-sulfates have been detected in mouse [Citation18]. It is, therefore, possible that the physiological relevance of the sulfation of bile acids may differ between humans and mouse.
In humans, SULT2A1 constitutes the sole member of the SULT2A subfamily and catalyzes the sulfation of a wide range of steroids, including dehydroepiandosterone (DHEA), androsterone, testosterone, estradiol, as well as bile acids [Citation2,9]. Previous studies have shown that human SULT2A1 catalyzes exclusively the sulfation of the 3α-hydroxyl group of bile acids [Citation17]. In contrast, mouse SULT2A subfamily comprises eight members (mSULT2A1, mSULT2A2, mSULT2A3, mSULT2A4, mSULT2A5, mSULT2A6, mSULT2A7, and mSULT2A8) [Citation5,19,20]. The fact that there are eight SULT2A members may imply the occurrence of the duplication of SULT2A genes in the mouse genome. Mouse SULT2A1 has been well characterized and shown to be capable of catalyzing the sulfation of a range of steroids [Citation2,9]. However, the functional properties of most other mouse SULT2A enzymes have remained poorly understood. A recent study has demonstrated that mouse SULT2A8 is uniquely capable of catalyzing specifically the sulfation of 7-O-hydroxyl group of bile acids [Citation21]. Generally speaking, the majority of SULT enzymes possess a broad range of substrate specificity in order to detoxify and eliminate numerous xenobiotics as well as endogenous compounds [Citation2,4,9]. A better understanding of the mechanism underlying the sulfation of 7-O-hydroxyl group of bile acids may not only explain the strict substrate specificity of mouse SULT2A8, but also shed light on its physiological relevance.
In this study, we performed an amino acid sequence alignment analysis and showed that mouse SULT2A8 contains two unique amino acid residues, His48 and Leu99, which may play critical roles in catalysis. Enzymatic characterization of mouse SULT2A8 mutants, in comparison with the wild-type enzyme, confirmed the critical role of these two amino acid residues in the sulfation of 7α-hydroxyl group of bile acids.
Materials and methods
Materials
Pregnenolone, dehydroepiandrosterone (DHEA), cholic acid (CA), deoxycholic acid (DCA), chenodeoxycholic acid (CDCA), taurocholic acid sodium salt hydrate (TCA), sodium taurochenodeoxy cholate (TCDCA), and glycocholic acid hydrate (GCA) were products of Sigma Chemical Company. Ursodeoxycholic acid (UDCA) was from Phoenix Pharmaceuticals Inc. An I.M.A.G.E. cDNA clone ID 1499725 (GenBank accession number AI119604) encoding mSULT2A8 was obtained from Genome Systems, Inc. pBluescript II SK (+), XL1-Blue MRF′ and BL21 Escherichia coli host strains were from Stratagene. pGEX-2TK prokaryotic GST fusion vector and glutathione Sepharose 4B were from GE Healthcare Biosciences. All other chemicals were of the highest grade commercially available.
Construction of SULT phylogenetic dendrogram
Amino acid sequences of mouse SULTs were collected from the GenBank database at the National Center for Biotechnology Information (NCBI) website. Amino acid sequences were aligned using the ClustalW program (http://www.genome.jp/tools/clustalw/) and the phylogenetic dendrogram was constructed based on the unweighted pair group method with arithmetic mean (UPGMA) method using MEGA6.
Molecular cloning of mouse SULT2A8
The I.M.A.G.E. cDNA clone1499725, identified by searching the expressed sequence tag (EST) database, was used as a template for the PCR amplification of mouse SULT2A8 cDNA. Using sense (5′-CGCGGATCCATGACAGATGAATTTCTGTGG-3′) and antisense (5′-CGCGGATCCTTATTCCCATGAAAAGAGCTC-3′) oligonucleotide primers (), a PCR in a 20 μL reaction mixture was carried out under the action of Pfu Turbo DNA polymerase (Stratagene), with the plasmid harboring clone 1499725 cDNA as the template. Amplification conditions were 30 cycles of 0.5 min at 94 °C, 0.5 min at 50 °C and 3 min at 72 °C. Purified PCR product was subcloned into the Bam HI site of pBluescript II SK(+) and transformed into E. coli XL1-Blue MRF’. To verify its authenticity, the mouse SULT2A8 cDNA insert was subjected to nucleotide sequencing [Citation22]. Upon verification, the cDNA was released by Bam HI digestion and subcloned into the Bam HI site of the pGEX-2TK prokaryotic expression vector.
Table 1. Oligonucleotide primers used for the cloning of mSULT2A8 and the construction of mutant mSULT2A8.
Generation of mouse SULT2A8 mutants, H48N, H48T, L99H
Mutagenic primers encoding single amino acid changes (His48Asn, His48Thr, or Leu99His) were synthesized and used in the PCR-amplification of mutated mouse SULT2A8 cDNAs. Each reaction mixture contained 250 ng of pGEX-2TK harboring wild-type mouse SULT2A8 cDNA, 0.4 μM primers (cf. ), 200 μM of each of the four deoxynucleotide triphosphates (dNTPs), and 1.25 units of Pfu Turbo DNA polymerase. Amplification conditions were 12 cycles of 0.5 min at 95 °C, 0.5 min at 55 °C and 6 min at 68 °C. The resulting PCR products were treated with Dpn I for 1 h at 37 °C, followed by transformation into E. coli XL1-Blue MRF’. The authenticity of the mutant SULT2A8 cDNA sequences was verified by nucleotide sequencing.
Bacterial expression and purification of wild-type and mutated mouse SULT2A8 enzymesp
GEX-2TK harboring wild-type or mutated SULT2A8 cDNA was transformed into competent E. coli BL21 cells. Transformed BL21 cells were grown to OD600 nm = 0.3 in 200 mL LB medium supplemented with 100 μg/mL ampicillin and induced with 0.1 mM IPTG. After a 4-h induction at 24 °C, the cells were collected by centrifugation and homogenized in 15 mL ice-cold lysis buffer (50 mM Tris–HCl, pH 8.0, 150 mM NaCl, and 1 mM EDTA) using an Ohtake French Press. The crude homogenate was subjected to centrifugation at 20,400 × g for 15 min at 4 °C. The supernatant collected was fractionated using 0.5 mL of glutathione Sepharose 4B, and the bound mouse SULT2A8-GST fusion protein was treated with 0.5 mL of a thrombin digestion buffer (50 mM Tris–HCl, pH 8.0, 150 mM NaCl, and 2.5 mM CaCl2) containing 5 U/mL bovine thrombin. Following a 2-h digestion at 4 °C, the preparation was subjected to centrifugation, and the supernatant containing purified mouse SULT2A8 was collected and used in the enzymatic assay.
Enzymatic assay
The sulfating activity of wild-type and mutant SULT2A8 enzymes was assayed using [35S]-PAPS as the sulfonate donor. The standard assay mixture, with a final volume of 25 μL, contained 50 mM Bis-tris propane-HCl (pH 6.27), 0.5 μM [35S]-PAPS (45 Ci/mmol), and 10 μM substrate (). The reaction was started by the addition of the enzyme, allowed to proceed for 30 min at 37 °C, and terminated by heating at 100 °C for 3 min. The precipitates formed were eliminated by centrifugation, and the supernatant was subjected to the analysis of [35S]-sulfated product using a previously developed thin-layer chromatography separation procedure [Citation23], with n-butanol/isopropanol/formic acid/water (3:1:1:1; by volume) as the solvent system. Afterwards, the TLC plate was air-dried and analyzed using a fluoro image analyzer (FLA-3000G).
Miscellaneous method
To prepare the solutions for use in the enzymatic assay, all substrate compounds were dissolved in dimethyl sulfoxide. [35S]-PAPS (45 Ci/mmol) was synthesized from ATP and [35S]-sulfate using recombinant human PAPS synthetase, as previously described [Citation24]. Sodium dodecyl sulfate–polyacrylamide gel electrophoresis (SDS–PAGE) was performed on a 10% polyacrylamide gel using the method of Laemmli [Citation25]. Protein determination was performed based on Lowry’s method, with bovine serum albumin as the standard [Citation26].
Results and discussion
The mouse genome has been shown to contain eight SULT2A genes (cf. Figures , and ) and all these eight genes are present closely in the same chromosome [Citation19]. Therefore, the SULT2A gene appears to have been duplicated several times in the mouse chromosome, leading to the complicated regulation of the sulfation of steroid hormones and bile acids in mouse. The enzymatic characteristics and differential physiological roles of individual mouse SULT2A enzymes have not been fully understood. A recent study has demonstrated that the mouse SULT2A8 is uniquely capable of catalyzing specifically the 7α-hydroxyl bile acids [Citation21]. In order to gain insight into the catalytic mechanism of SULT2A8, we performed a sequence alignment analysis of human and mouse SULT2A enzymes and carried out a mutational analysis of mouse SULT2A8. We report here that two unique amino acid residues, His48 and Leu99, are critical to the bile acid 7-O-sulfating activity of mouse SULT2A8.
Figure 2. Phylogenic tree analysis of mouse SULTs on the basis of their amino acid sequences. Notes: The dendrogram shows the degree of amino acid sequence homology among mouse SULTs. Protein sequences were extracted from the GeneBank database and accession number of individual SULTs are as follows: mSULT1A1(NP_598431), mSULT1B1 (NP_063931), mSULT1C1 (NP_061221), mSULT1C2 (NP_081211), mSULT1D1 (NP_058051), mSULT1E1 (NP_075624), mSULT2A1 (NP_001104766), mSULT2A2 (AAA40145), mSULT2A3 (NP_001095056), mSULT2A4(NP_001095004), mSULT2A5 (NP_001171909), mSULT2A6 (NP_001074794), mSULT2A7 (modified from LOC194586), mSULT2A8 (NP_780459), mSULT2B1a1(XP_006541060), mSULT2B1a2(XP_006541061), mSULT2B1b (NP_059493), mSULT3A1(NP_065590), mSULT3A2 (NP_001094922), mSULT4A1 (NP_038901), mSULT5A1 (NP_065589), mSULT6B1 (NP_001157097). Construction of dendrogram was performed based on the methods as described in “Materials and Methods”.
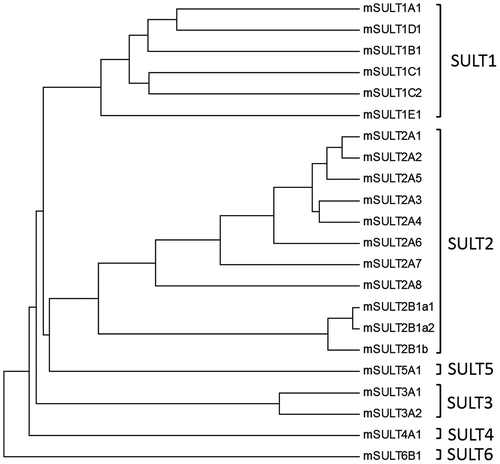
Figure 3. Amino acid sequence alignment analysis of human and mouse SULT2A subfamily members. Notes: Identical residues conserved among at least two of the nine SULT enzymes are drawn in Black, and similar residues are in gray. 5′-PSB loop and 3′-PB motif located, respectively, in the N-terminal and middle regions are underlined. All SULTs, except mSULT2A8, contain His99 residue in their amino acid sequences as indicated by solid arrow. His48 residue of mouse SULT2A8, as indicated by boxed arrow, is also unique as compared with other SULTs.
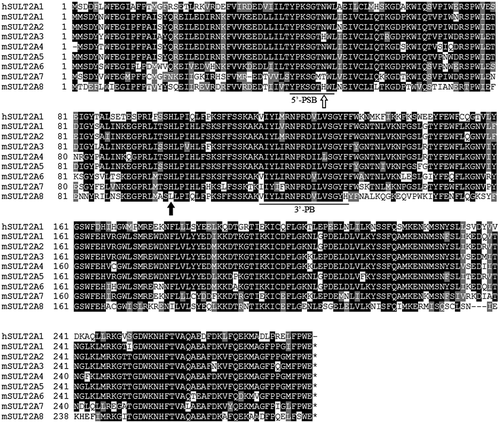
Molecular cloning of mouse SULT2A8 and sequence alignment analysis
The open reading frame of mouse SULT2A8 was PCR amplified using the I.M.A.G.E. cDNA clone ID 1499725 (GenBank accession number AI119604) as the template in conjunction with gene-specific primers (). Sequence data obtained for the amplified product completely matched that previously reported for the mouse SULT2A8 (GenBank accession number NM_175250) [Citation21]. The open reading frame of mouse SULT2A8 encompasses 849 nucleotides and encodes a 282 amino acid polypeptide. Two signature sequences, a 5′-phosphosulfate binding loop (5′-PSB loop) in the N-terminal region and a 3′-phosphate binding motif (3′-PB motif) in the middle, were found in mouse SULT2A8 sequence [Citation27]. Sequence analysis indicated that the deduced amino acid sequence of mouse SULT2A8 displays 58, 59, 58, 59, 57, 60, 54, and 59% amino acid sequence identity to mouse SULT2A1, SULT2A2, SULT2A3, SULT2A4, SULT2A5, SULT2A6, SULT2A7 and human SULT2A1, respectively. It is noted that a catalytic histidine (His) residue, normally positioned at around 100th amino acid residue, conserved among all other SULTs is absent in mouse SULT2A8 and is substituted by a Leu residue located at position 99th (). This conserved His residue found in other SULTs has been shown to be essential in the sulfation reaction [Citation27]. Another interesting feature is that mouse SULT2A8 carries a His residue at the 49th amino acid residue position in the 5′-PSB loop (). Since the 5′-PSB loop is located in the active site and interacts with the sulfate group of PAPS, this His residue may play an essential role in the catalytic action of mouse SULT2A8. It is possible that substitution of these two amino acid residues, Asn to His at 48th residue and His to Leu at 99th residue, might have occurred during the duplication of mouse SULT2A gene, resulting in the unique substrate specificity of SULT2A8.
Expression and purification of wild-type and mutant mouse SULT2A8
Recombinant wild-type and mutant mouse SULT2A8 enzymes were generated, expressed, and purified as described in the Materials and Methods section. Purified recombinant wild-type and mutant SULT2A8 enzymes all migrated at ~33 kDa positions upon SDS-polyacrylamide gel electrophoresis (figure not shown). These results were in good agreement with the predicted molecular weight (32,295) of wild-type mouse SULT2A8.
Enzymatic characterization of wild-type mouse SULT2A8
Mammalian SULT2A subfamily members are known to be capable of catalyzing the sulfation of hydroxysteroids including bile acids [Citation2,9]. A previous study showed that mouse SULT2A8 displayed 7α-hydroxyl bile acid-preferring activity and is likely responsible for the production of bile acid-7-O-sulfates [Citation21]. We first performed enzymatic assays to verify the substrate specificity of wild-type mouse SULT2A8 using a panel of bile acids as substrates. Among the substrates tested, mouse SULT2A8 displayed sulfating activities toward 7α-hydroxyl cholic acids (CA, GCA, CDCA, TCA, TCDCA, UDCA) that carry a hydroxyl group at the 7-position (). In contrast, mouse SULT2A8 showed no sulfating activity toward DCA, a secondary bile acid generated from CDCA via the 7α-dehydration under the action of intestinal bacteria [Citation28], and other typical substrates, e.g. pregnenolone and DHEA, for SULT2A enzymes. Kinetic analysis showed that SULT2A8 exhibited the highest substrate affinity and catalytic activity toward CA among other 7α-hydroxyl bile acids (). These data may be useful in understanding the mechanism of the production of a main bile acid form, cholic acid-7-O-sulfate, in mouse [Citation18]. To further investigate the enzymatic characteristics of the bile acid-7-O-sulfation by SULT2A8, a pH-dependence experiment was carried out using 10 μM CA as the substrate. Results obtained showed that SULT2A8 displayed a pH optimum at 6.27 with CA as the substrate (date not shown). Previous studies have demonstrated that human SULT2A1 displayed a pH optimum at 8.5 with LCA as the substrate [Citation17]. It therefore appears that the ionization status of the active site, including the catalytic His residue (with a side chain pKa ≈ 6.0), of SULT2A8 may underscore the difference with human SULT2A1.
Table 2. Specific activity of mSULT2A8 with bile acids and steroids a.
Table 3. Kinetic parameters of the sulfation of cholic acids by mSULT2A8 a.
Catalytic activity of mSULT2A8 mutant enzymes
As shown above, the primary structure of SULT2A8 contains two unique amino acid residues, His48 and Leu99 (). To obtain clues to the special locations of these two amino acid residues in mouse SULT2A8, the crystal structure of human SULT2A1, in complex with LCA and PAP (PDBid:3F3Y), was examined. In the active site of human SULT2A1, Thr47, Asn48, and His99 form a pore structure accessible to the acceptor hydroxyl group. Based on this information, it can be speculated that His48 of mouse SULT2A8 may be involved in the transfer of the sulfonate group from PAPS as does His99 of human SULT2A1 [Citation27], and Leu99 of mouse SULT2A8 may play a role in the substrate recognition. To verify the contributions of these two residues to the bile acid-7-O-sulfation, a mutational analysis was performed using three mouse SULT2A8 mutants, H48N, H48T, L99H. As shown in , H48N, H48T, and L99H mutants displayed lower or no sulfating activity toward CA and GCA, compared with the wild type SULT2A8. These three mutant enzymes also showed much lower affinity (higher K m values) and catalytic efficiency (lower V max/K m values) with CA than the wild-type enzyme (). Remarkably, while the L99H mutant was less capable of catalyzing the sulfation of CA, the mutant appeared to be capable of catalyzing the sulfation of DHEA. These results suggested that Leu99 residue of mouse SULT2A8 may be critical to the strict substrate specificity toward 7α-hydroxyl bile acids.
Table 4. Specific activity of mutant mSULT2A8 with cholic acids and steroidsa.
Table 5. Kinetic parameters of the sulfation of cholic acid by wild-type and mutant mSULT2A8a.
In summary, the current study confirmed that mouse SULT2A8, which carries unique amino acid residues His48 and Leu99, catalyzed strictly the sulfation of 7α-hydroxyl group of bile acids in mouse. Mutational analysis revealed that Leu99 and His48 residues indeed play an important role in the recognition of 7α-hydroxyl groups of bile acid substrates. The catalytically essential His residue located around the 100th amino acid residue is generally conserved among all reported SULTs, but not SULT2A8 [Citation27]. The substituted Leu99 in mouse SULT2A8 appeared to be involved in the specific substrate recognition of mouse SULT2A8 for 7α-hydroxyl bile acids. Moreover, His48 present in the 5′-PSB loop of mouse SULT2A8 appeared to act as a catalytic residue as do those of other SULTs. Further investigation is warranted in order to clarify further the catalytic mechanism of mouse SULT2A8-mediated 7-O-sulfation of bile acids. It is worthwhile mentioning that intestinal bacteria, particularly the Clostridium strains, are capable of hydrolyzing bile acid-3-O-sulfate but not 7-O-sulfate [Citation29]. Since 7α-hydroxyl group is subjected to the dehydration by intestinal bacteria, 7α-O-sulfation of bile acids by mouse SULT2A8 may represent a key reaction for preventing the production of toxic secondary bile acids.
Author contribution
T. S., K. K., and Y. S. conceived and designed the research. T. S. performed experiments and T. S., K. K., and Y. S. analyzed data. M.-C. L., and M. S. provided conceptual advice. T. S., K. K., M.-C. L., and Y. S. wrote and edited the manuscript and all authors read and approved the final manuscript.
Funding
This work was supported by JSPS KAKENHI [grant numbers 23580138 (M.S.), 21580114 (Y.S.), 15H04502 (Y.S.), 17H05028 (K.K.).
Disclosure statement
No potential conflict of interest was reported by the authors.
Acknowledgment
The authors are grateful to Masataka Teramoto and Yoshimitsu Kakuta for valuable discussions, and Makiko Sonoda for technical assistance.
References
- Lipmann F. Biological sulfate activation and transfer. Science. 1958;128:575–580.10.1126/science.128.3324.575
- Falany CN. Enzymology of human cytosolic sulfotransferases. FASEB J. 1997;11:206–216.10.1096/fasebj.11.4.9068609
- Hemmerich S, Verdugo D, Rath VL. Strategies for drug discovery by targeting sulfation pathways. Drug Discov Today. 2004;9:967–975.10.1016/S1359-6446(04)03261-1
- Gamage N, Barnett A, Hempel N, et al. Human sulfotransferases and their role in chemical metabolism. Toxicol Sci. 2006;90:5–22.10.1093/toxsci/kfj061
- Blanchard RL, Freimuth RR, Buck J, et al. A proposed nomenclature system for the cytosolic sulfotransferase (SULT) superfamily. Pharmacogenetics. 2004;14:199–211.10.1097/00008571-200403000-00009
- Yamazoe Y, Nagata K, Ozawa S, et al. Structural similarity and diversity of sulfotransferases. Chem Biol Interact. 1994;92:107–117.10.1016/0009-2797(94)90057-4
- Weinshilboum RM, Otterness DM, Aksoy IA, et al. Sulfation and sulfotransferases 1: Sulfotransferase molecular biology: cDNAs and genes. FASEB J. 1997;11:3–14.10.1096/fasebj.11.1.9034160
- Freimuth RR, Wiepert M, Chute CG, et al. Human cytosolic sulfotransferase database mining: identification of seven novel genes and pseudogenes. Pharmacogenomics J. 2004;4:54–65.10.1038/sj.tpj.6500223
- Otterness DM, Wieben ED, Wood TC, et al. Human liver dehydroepiandrosterone sulfotransferase: molecular cloning and expression of cDNA. Mol Pharmacol. 1992;41:865–872.
- Borgström B, Barrowman JA, Lindström M. Roles of bile acids in intestinal lipid digestion and absorption. In: Danielsson H, Sjövall J, editors. Sterols and Bile Acids. Vol. 12. Amsterdam: Elsevier; 1985. p. 405–425.10.1016/S0167-7306(08)60690-0
- Kalaany NY, Mangelsdorf DJ. LXRS AND FXR: the yin and yang of cholesterol and fat metabolism. Annu Rev Physiol. 2006;68:159–191.10.1146/annurev.physiol.68.033104.152158
- Claudel T, Staels B, Kuipers F. The farnesoid X receptor: a molecular link between bile acid and lipid and glucose metabolism. Arterioscler Thromb Vasc Biol. 2005;25:2020–2030.10.1161/01.ATV.0000178994.21828.a7
- Staudinger JL, Lichti K. Cell signaling and nuclear receptors: new opportunities for molecular pharmaceuticals in liver disease. Mol Pharm. 2008;5:17–34.10.1021/mp700098c
- Kim ND, Moon JO, Slitt AL, et al. Early Growth response factor-1 is critical for cholestatic liver injury. Toxicol Sci. 2006;90:586–595.10.1093/toxsci/kfj111
- Fukase K, Ohtsuka H, Onogawa T, et al. Bile acids repress E-cadherin through the induction of Snail and increase cancer invasiveness in human hepatobiliary carcinoma. Cancer Sci. 2008;99:1785–1792.10.1111/cas.2008.99.issue-9
- Palmer RH. The formation of bile acid sulfates: a new pathway of bile acid metabolism in humans. Proc Natl Acad Sci USA. 1967;58:1047–1050.10.1073/pnas.58.3.1047
- Radominska A, Comer KA, Zimniak P, et al. Human liver steroid sulphotransferase sulphates bile acids. Biochem J. 1990;272:597–604.10.1042/bj2720597
- Eyssen HJ, Parmentier GG, Mertens JA. Sulfate bile acids in germ-free and conventional mice. Eur J Biochem. 1976;66:507–514.10.1111/ejb.1976.66.issue-3
- Kocarek TA, Duanmu Z, Fang HL, et al. Age- and sex-dependent expression of multiple murine hepatic hydroxysteroid sulfotransferase (SULT2A) genes. Biochem Pharmacol. 2008;76:1036–1046.10.1016/j.bcp.2008.07.032
- Suiko M, Kurogi K, Hashiguchi T, et al. Updated perspectives on the cytosolic sulfotransferases (SULTs) and SULT-mediated sulfation. Biosci Biotechnol Biochem. 2017;81:63–72.10.1080/09168451.2016.1222266
- Feng L, Yuen YL, Xu J, et al. Identification and characterization of a novel PPARalpha-regulated and 7alpha-hydroxyl bile acid-preferring cytosolic sulfotransferase mL-STL (Sult2a8). J Lipid Res. 2017;58:1114–1131.10.1194/jlr.M074302
- Sanger F, Nicklen S, Coulson AR. DNA sequencing with chain-terminating inhibitors. Proc Natl Acad Sci USA. 1977;74:5463–5467.10.1073/pnas.74.12.5463
- Liu MC, Lipmann F. Decrease of tyrosine-O-sulfate-containing proteins found in rat fibroblasts infected with Rous sarcoma virus or Fujinami sarcoma virus. Proc Natl Acad Sci USA. 1984;81:3695–3698.10.1073/pnas.81.12.3695
- Yanagisawa K, Sakakibara Y, Suiko M, et al. cDNA cloning, expression, and characterization of the human bifunctional ATP sulfurylase/adenosine 5′-phosphosulfate kinase enzyme. Biosci Biotechnol Biochem. 1998;62:1037–1040.10.1271/bbb.62.1037
- Laemmli UK. Cleavage of structural proteins during the assembly of the head of bacteriophage T4. Nature. 1970;227:680–685.10.1038/227680a0
- Lowry OH, Rosebrough NJ, Farr AL, et al. Protein measurement with the Folin phenol reagent. J Biol Chem. 1951;193:265–275.
- Negishi M, Pedersen LG, Petrotchenko E, et al. Structure and function of sulfotransferases. Arch Biochem Biophys. 2001;390:149–157.10.1006/abbi.2001.2368
- Hofmann AF. detoxification of lithocholic acid, a toxic bile acid: relevance to drug hepatotoxicity. Drug Metab Rev. 2004;36:703–722.10.1081/DMR-200033475
- Robben J, Parmentier G, Eyssen H. Isolation of a rat intestinal Clostridium strain producing 5 alpha- and 5 beta-bile salt 3 alpha-sulfatase activity. Appl Environ Microbiol. 1986;51:32–38.