ABSTRACT
We previously reported an orexigenic action of oral zinc administration in male Sprague-Dawley (SD) rats during an early stage of feeding with a zinc-deficient diet, without decreased zinc concentrations in tissues. The overall conclusion was that orally but not intraperitoneally administered zinc stimulates food intake in short-term zinc-deficient-diet fed rats. We here investigate the mechanism of the orexigenic action of zinc using GC-MS/MS-targeted metabolomic analysis in the rat hypothalamus. Four-week-old, male SD/Slc rats were used, and after 2 days of feeding with a zinc-deficient diet, 3 mg of ZnSO4 in 5 mL saline solution were administered to each rat either orally or intraperitoneally. Three hours after administration, the rats were sacrificed and the hypothalamus were excised and analyzed. We found that the oral administration group showed increased concentrations of 3-aminopropanoic acid (β-alanine), hypotaurine, dopamine, and biotin. In light of metabolomic analysis of these results, we indicate directions for further research.
Graphical Abstract

Plot of the results of an OPLS-DA for Zn-PO (oral) and Zn-IP (intraperitoneal) groups, and S-plot by OPLS-DA revealed several metabolites were increased in the hypothalamus by Zn-PO.
Zinc is required by humans and animals for many physiological functions, such as growth, immune function, and reproduction. Zinc deficiency induces several physiological problems, including anorexia, growth retardation, dermatitis, taste disorder, and hypogonadism. Within 3 to 5 days after feeding a zinc-deficient diet, food intake is suppressed [Citation1,Citation2]. This suppression of food intake is the first sign of zinc deficiency and thereafter, other symptoms associated with zinc deficiency occur [Citation2], suggesting that zinc plays an important role in food intake regulation [Citation1]. We have reported an orexigenic action of oral zinc administration in male Sprague-Dawley (SD) rats during an early stage of feeding with a zinc-deficient diet (the 2nd to 3rd days of feeding) in the absence of decreased zinc concentrations in tissues (hypothalamus and liver). As a result, we found that orally (PO) but not intraperitoneally (IP) administered zinc stimulates food intake in short-term zinc-deficient-diet–fed rats [Citation2] (Supplemental Figure 1), although we found no difference in the plasma or liver zinc concentrations between the Zn-PO and Zn-IP groups (Supplemental Figure 2) [Citation3]. The mRNA expressions of hypothalamic peptides such as orexin and neuropeptide Y (NPY) increased after oral administration of zinc, which also produced increased food intake [Citation4]. Pretreatment with an antagonist of the NPY Y1 receptor or the orexin OX1 receptor blocked the orexigenic activity of zinc administration. The stimulation of food intake by oral administration of zinc was also abolished by vagotomy. Taken together, these previous results indicate that zinc stimulates food intake through the afferent vagus nerve followed by activation of the hypothalamic peptide associated with regulation of food intake [Citation2]. These studies suggested that the gastrointestinal zinc signal is indispensable for food appetite induction in the experimentally anorexigenic rat. However, the mechanisms of zinc sensing by the epithelial membrane of the gastrointestinal tract and of appetite control by the hypothalamic brain area remain to be fully clarified. We therefore investigated the mechanism of the orexigenic action of oral zinc administration using gas-liquid chromatography–tandem mass spectrometry (GC-MS/MS) to target metabolomic analysis in the SD rat hypothalamus.
Materials and methods
Animals and tissue sampling
Four-week-old, male Sprague-Dawley rats (SD/Slc) were used. Each rat was individually housed under regulated conditions (room temperature 22 ± 2°C, humidity 50 ± 5%, on a 12-h-light/12-h-dark cycle, lights on 08:00–20:00) and drinking water with free access. A commercial pelleted diet (F-2, Funabashi Farms Corporation, Chiba, Japan; protein, 21%; fat, 5%; carbohydrate, 58%) were fed given acclimation period. The experimental design for the present study was approved by the Animal Research-Animal Care Committee of Tohoku University (2017-AgA-020).
After 2 days of feeding with the zinc-deficient diet (0.7 ppm Zn, ), 3 mg of ZnSO4 in 5 mL of saline solution (5 mL/kg body weight) were administered to each rat orally (“per oralis”) (Zn-PO);n = 4 or intraperitoneally (Zn-IP);n = 3, and vehicle saline solution was administered orally (Saline-PO);n = 4. The experimental diets were prepared as previously described by Ohinata et al. [Citation2]. Three hours after administration, rats were sacrificed by decapitation and blood samples were collected. Thereafter, the brain samples were obtained in the same manner as previously described [Citation5], and also confirmed by the rat brain atlas [Citation6]; i.e., about 10 mg of the hypothalamus, hippocampus, and amygdala were excised into Eppendorf tubes and frozen immediately in liquid nitrogen. These tissues were weighed with included zirconia beads in anticipation of a tissue homogenization step and stocked in a deep freezer at −80°C pending GC-MS/MS analysis.
Table 1. Composition of the zinc-deficient diet.
The blood sample was centrifuged at 3000 rpm for 10 min at 4°C and the upper-layer serum was kept in a deep freezer at −80°C pending zinc analysis. Serum zinc concentration was measured by an atomic absorption spectrophotometer (AAS-6800, Shimadzu Corporation, Kyoto, Japan).
Metabolite extraction and derivatization
Metabolites were extracted using a modification of the Bligh-Dyer method [Citation7]. Four hundred microliters of cold phosphate-buffered saline were added to each tube of frozen tissue, and the tissue samples were homogenized at 2200 rpm for 30 s using a bead-beater type of homogenizer (μT-12, Taitec Co., Saitama, Japan). Immediately, 500 μL of cold methanol and 250 μL of cold chloroform were added to each homogenized sample followed by vortexing for 2 min. Five μL of internal standard solution (2-isopropylmalic acid, 1 mg/mL) were added to the mixture, and the mixture let stand at room temperature (RT) for 5 min. Next, 250 μL of Milli QTM water and 250 μL of chloroform were added to each sample followed by vortexing for 2 min. The mixtures were then centrifuged at 14,000 rpm for 6 min at RT. Five hundred microliters of supernatant were transferred to new tubes and dried in an Eppendorf concentrator at RT for 3 h. The fully dried samples were then combined with 80 μL of methoxyamine hydrochloride in pyridine (20 mg/mL), vortexed for 30 s, and sonicated for 30 s until the solids were fully dispersed. The samples were shaken at 30°C for 90 min at 200 rpm (BioShakerTM, Taitec Co., Saitama, Japan). After shaking, 80 μL of N-methyl-N-trimethylsilyltrifluoroacetamide were added to the samples followed by further shaking at 37°C for 30 min at 200 rpm using the BioShaker. Finally, the derivatized samples were centrifuged at 14,000 rpm for 5 min at RT and 100 μL of supernatant were transferred to GC-MS vials using micro tubes.
Metabolomic analysis
The analysis of metabolites was performed by gas chromatography-tandem mass spectrometry (GC-MS/MS) using a multiple-reaction monitoring mode. The GC-MS/MS analysis was performed on a GCMS-TQ8040 system (Shimadzu Corporation, Kyoto, Japan) equipped with a DB-5 capillary column (30 m × 0.25 mm i.d., film thickness 1 μm; Agilent, Santa Clara, CA, USA). Each 1.0-μL aliquot of the derivatized sample solution was automatically injected in splitless mode into the gas-liquid chromatography (GC) column using an auto-injector (AOC-20i, Shimadzu Corporation, Kyoto, Japan). During the GCMS-TQ8040 analysis, the injector temperature was kept at 280°C, and helium was used as a carrier gas at a constant flow rate of 39.0 cm/s. The GC column temperature was programmed to remain at 100°C for 4 min and then to rise from 100°C to 320°C at a rate of 10°C/min, then remain at 320°C for a further 11 min. The total GC run time was 37 min. The mass spectrometry transfer line and ion-source temperatures were 280°C and 200°C, respectively. The ionization voltage was 70 eV. Argon was used for collision-induced dissociation. Metabolite detection was performed using the Smart Metabolites Database Ver. 2 (Shimadzu Corporation, Kyoto, Japan) by the method of a previous study with some modifications [Citation8]. The 2-isopropylmalic acid contained in the extraction solution was also used to evaluate the stability of our GC-MS/MS analysis system. Peak identification was performed automatically and then confirmed manually based on the specific precursor and product ions and the retention time.
Multivariate statistical analysis
The integral metabolomic datasets were imported into SIMCA version 13.0 (Umetrics, Umea, Sweden), and all variables were scaled to Pareto (par) for orthogonal partial least squares discriminant analysis (OPLS-DA). The use of chemometrics tools, e.g., principal component analysis (PCA), partial least-squares to latent structures (PLS), and orthogonal PLS (OPLS), is of great importance as these include efficient, validated, and robust methods for modeling information-rich chemical and biological data. Further, the S-plot is proposed as a tool for visualization and interpretation of multivariate classification models, e.g., OPLS discriminate analysis (OPLS-DA), having two or more classes. The S-plot visualizes both the covariance and correlation between the metabolites and the modeled class designation. So, significant metabolites were selected from the S-plot, in which both intensity and reliability were visualized by statistical significance, such that 0.8 < p (corr) <1.0 or −1.0 < p (corr) < −0.8 [Citation9]. Selected data are represented as means ± standard error and were compared using Student’s t-test by IBM SPSS Statistics 22 (Armonk, NY, USA). The selected differential metabolites were used for KEGG pathway analysis (https://www.genome.jp/kegg-bin/show_pathway?153251030022929/map01100.args).
Results
Our GC-MS/MS-based metabolite analysis system detected 356 metabolites in the sampled tissues from hypothalamus, 335 from hippocampus, and 356 from amygdala. shows the OPLS-DA scores of metabolic profiles in the selected brain areas plotted to determine the quality of sampling. The metabolic profiles of the three brain regions classified into three distinct clusters, which means the metabolisms in these mid-brain areas were distinct. The amygdala and hypothalamus are the food-intake controling brain sites, however, there was no distinct difference between Zn-IP and Zn-PO groups in the analyzed metabolites of the amygdala. Therefore, only the hypothalamic analysis was continued in detail to clarify the orexigenic action of oral zinc administration, only the hypothalamic analysis was continued in detail. The profile pattern of the hypothalamic metabolites is shown in . The metabolites plotted above the x-axis in the Zn-PO group, but below in the other two groups (Zn-IP and Saline-PO), although we observed a distribution difference between the latter two groups. From the plots, various metabolites could be identified as responsible for the separation between the Zn-IP and Zn-PO groups, and these were therefore viewed as potential biomarkers. Potential markers of interest were extracted from S-plots () constructed following OPLS-DA, and markers were chosen based on their contribution to the variation and correlation within the data. Therefore, the S-plot helps in identifying statistically and (potentially) biochemically significant metabolites. shows the comparative S-plot between Zn-IP and Zn-PO groups in the hypothalamus, and reveals significant increases in the Zn-PO group were observed in 3-amino-propanoic acid (β-alanine), dopamine (−4TMS), hypotaurine, sorbose, cytidine, octopamine, and biotin and so on compared with Zn-IP group by the amount (x-axis) order; and also significant but small amount increases in the Zn-IP group were observed in dopamine (−3TMS), ureidopropionic acid, succinylacetone, and deoxy-glucose compared with Zn-PO group, judging from OPLS-DA and/or Student’s t-test. These extracted metabolites were also listed the upper side in that shows the significantly changed metabolites in the Zn-IP and Zn-PO groups extracted by S-plot and/or Student’s t-test analyses.
Figure 1. Shown is a plot of the results of an orthogonal partial least squares discriminant analysis (OPLS-DA) for three brain regions.
The x-axis shows intra-group variance and the y-axis shows inter-group variance. Each point represents the results of analysis of data from one rat. t[Citation1] = scores for predictive component 1, t[Citation2] = scores for predictive component 2. R2X[Citation1] = 0.0938; R2X[Citation2] = 0.0757. The ellipse shows the 95% confidence interval using Hotelling’s T2 statistics.
![Figure 1. Shown is a plot of the results of an orthogonal partial least squares discriminant analysis (OPLS-DA) for three brain regions.The x-axis shows intra-group variance and the y-axis shows inter-group variance. Each point represents the results of analysis of data from one rat. t[Citation1] = scores for predictive component 1, t[Citation2] = scores for predictive component 2. R2X[Citation1] = 0.0938; R2X[Citation2] = 0.0757. The ellipse shows the 95% confidence interval using Hotelling’s T2 statistics.](/cms/asset/cec4a15d-8b95-4096-b227-699ba842de9a/tbbb_a_1516543_f0001_oc.jpg)
Figure 2. An OPLS-DA plot for hypothalamus for the three experimental groups.
Each point represents the results of analysis of data from one rat; PO, oral administration; IP, intraperitoneal administration. t[Citation1] = scores for predictive component 1, t[Citation2] = scores for predictive component 2. R2X[Citation1] = 0.2100; R2X[Citation2] = 0.0672. The ellipse shows the 95% confidence interval using Hotelling’s T2 statistics.
![Figure 2. An OPLS-DA plot for hypothalamus for the three experimental groups.Each point represents the results of analysis of data from one rat; PO, oral administration; IP, intraperitoneal administration. t[Citation1] = scores for predictive component 1, t[Citation2] = scores for predictive component 2. R2X[Citation1] = 0.2100; R2X[Citation2] = 0.0672. The ellipse shows the 95% confidence interval using Hotelling’s T2 statistics.](/cms/asset/7804ec5a-3ccc-494b-be36-2d6c7cdf3bb6/tbbb_a_1516543_f0002_b.gif)
Figure 3. S-plot between the Zn-IP group and the Zn-PO group for hypothalamus; boxes, significant results; TMS, trimethylsilyl.
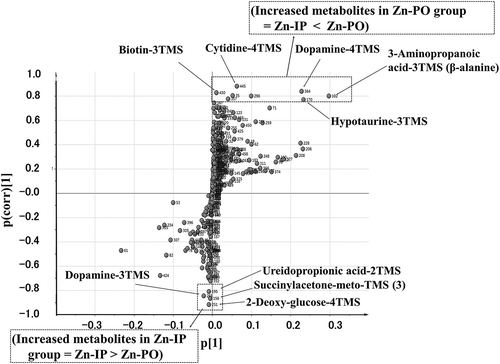
Table 2. Metabolites significantly changed according to S-plot analysis, Zn-IP group vs. Zn-PO group.
Selected metabolites from the Zn-PO group were then mapped to KEGG pathways (). Dopamine and hypotaurine were included in the dopamine-metabolism pathway, pertaining to one of the neurotransmitters, and biotin was included in the AMPK signaling pathway via acetyl-CoA carboxylase, which regulates food appetite.
Figure 4. KEGG pathway analysis. Significant metabolites of the Zn-PO group are mapped to KEGG metabolic pathways. https://www.genome.jp/kegg-bin/show_pathway?153251030022929/map01100.args.
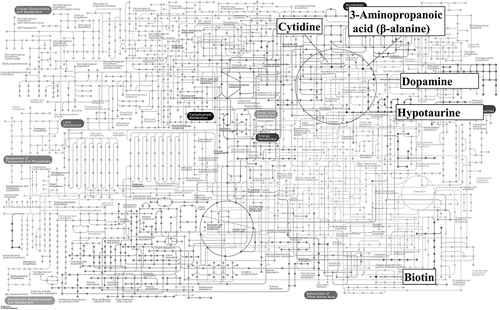
Finally, we summarize metabolites significantly highlighted by statistical comparison between the two groups in . In the hypothalamus, 3-amino-propanoic acid, hypotaurine, dopamine, and biotin increased significantly in the Zn-PO group compared with the Zn-IP group.
Discussion
In our previous research [Citation2], we concluded that orally but not intraperitoneally administered zinc stimulates food intake in short-term zinc-deficient rats. The mRNA expressions of hypothalamic peptides, such as orexin and NPY, were increased after an oral administration of zinc that produced increased food intake. The afferent vagus nerve signals the peripheral energy status to the central nervous system, and that signal reaches the hypothalamic area responsible for regulating food intake [Citation10,Citation11]. The orexigenic activity of zinc was blocked by vagotomy. Taken together, the results indicated that zinc stimulates food intake in short-term zinc-deficient rats via the afferent vagus nerve followed by activation of the hypothalamic peptide associated with regulation of food intake. However, the detailed mechanisms of hypothalamic food-intake regulation remain to be clarified, so the present GC-MS/MS- targeted metabolomic analysis of the rat hypothalamus was undertaken. Metabolic profiles of the three regions of the brain studied here (hypothalamus, hippocampus, and amygdala) classified to separate clusters, as shown in . The hypothalamic metabolites revealed a clear difference between the Zn-PO and Zn-IP groups relevant to food intake regulation as shown in . We analyzed the difference further by S-plot distribution. shows the comparative S-plot distribution of the hypothalamic metabolites between Zn-IP and Zn-PO groups. In the Zn-PO group, significantly increased metabolites were β-alanine (3-amino- propanoic acid)-3TMS, hypotaurine-3TMS, dopamine-4TMS, and biotin-3TMS. In the Zn-IP group, dopamine-3TMS was significantly increased. TMS stands for trimethylsilyl, a volatility-enhancing chemical group present for technical reasons.
In seeking explanations for the dopamine results, we note that high-dose zinc administration has been shown to inhibit the binding of dopamine to D1 and D2 receptors, which acts as antagonistic to dopamine activation, and are important interactions for dopamine’s food intake-inhibiting effect (= food intake acceleration by zinc). This theory suggests that zinc could be playing a regulatory role in food intake by an action on neurotransmitter receptors [Citation12]. Applying this theory to our results, we speculate that orally administered zinc partly binds to D1 and D2 receptors in the intestinal epithelium, and the resulting free dopamine would lead to an observation of increased dopamine in the Zn-PO group, although this idea must be investigated further in detail [Citation13]. The other speculated possibility is that the dopamine transporter in the central nervous system (e.g., hypothalamus) may be involved in this food intake regulation mechanism. The dopamine transporter (also dopamine active transporter, DAT, SLC6A3) is a membrane-spanning protein that pumps the neurotransmitter dopamine out of the synaptic cleft and deposits it into surrounding cells, thus terminating the signal of the neurotransmitter. Actually, the human dopamine transporter (hDAT) contains a high affinity extracellular zinc binding site which, upon zinc binding, serving as a DAT inhibitor by inhibiting dopamine reuptake[Citation14–Citation16]. These facts suggest that elevated Zn circulation via intestinal absorption through ZIP4 in the present experiment may inhibit DAT activity, consequently increase an extracellular remained dopamine concentration, though further elucidation must be undertaken at this point.
At this moment, the data in hand do not allow us to speculate on the reasons for the observed elevation in β-alanine or hypotaurine levels, although β-alanine is related to dopamine metabolism.
The increase in the biotin level observed in the Zn-PO group can be illuminated by considering the AMPK (AMP-activated protein kinase) signaling pathway, because biotin acts as a co-enzyme for acetyl-CoA carboxylase (ACC) and AMPK inactivates ACC by phosphorylation of its serine residue [Citation17]. However, at this moment we cannot explain the increased biotin level in the Zn-PO group in this experimental system, because ACC, a biotin-requiring enzyme, accelerates malonyl-CoA formation, which suppresses food intake [Citation18] through the inhibition of carnitine palmitoyltransferase 1 activity. Considering these facts, observation of an increased biotin level in the hypothalamus may be controversial.
However, it is well known that inactivation of ACC by AMPK requires ACC phosphorylation, and a recent study found that ACC-bound biotin does not change its form inside the protein molecule [Citation19] during changes in ACC phosphorylation status. This leads us to speculate that ACC inactivation by AMPK might reduce biotin binding to ACC because of low activity and thus low turnover, which could lead to an increase in free biotin in the hypothalamus. Concerning this point, a recent paper [Citation20] reported the important finding that the AMPK pathway is up-regulated in a fish, Synechogobius hasta, by zinc exposure in the aquatic environment. That paper used hepatic transcriptome analysis to conclude that AMPK is activated by zinc exposure.
Ghrelin is one of the peptide hormones secreted from the gastric mucosa, and acts as an orexigenic signal by stimulating NPY secretion by the hypothalamus [Citation21–Citation23]. This signaling may be characterized as an AMPK-pathway mechanism because it is known that ghrelin activates AMPK activity. Considering dietary zinc administration, an important paper appeared in 2008, which showed that dietary supplementation with zinc oxide stimulates ghrelin secretion from the stomach of young pigs [Citation24]. That paper concluded that ZnO directly stimulates ghrelin production by gastric mucosal cells, a mechanism consistent with a previous report by our group that NPY-mRNA expression in the hypothalamus increased in response to oral zinc administration [Citation2]. However, such a mechanism requires a dietary zinc receptor located in the gastric or small-intestinal mucosa, the identity of which remains unknown. This must be clarified in future experiments.
During the immediate postoperative period, a distinct difference between enteral and parenteral nutrition has been reported. For example, in rats, postoperative enteral nutrition augmented intestinal protein synthesis and immune defenses and thus led to lower stress than did parenteral nutrition [Citation25]. Lower stress and increased protein synthesis in the intestine may decrease whole-body protein breakdown and enhance muscle protein synthesis in postoperative enteral nutrition. In humans, oral zinc supplementation improved perioperative appetite in patients undergoing open-heart surgery or coronary-artery bypass [Citation26]. In other research, a group of gastrectomy patients who had received zinc supplementation, showed fewer post-operative inflammatory complications [Citation27].
It is known that zinc signaling falls into two types, early and late [Citation28]. In the case of early zinc signaling, an extracellular stimulus directly induces elevated zinc levels within several minutes by releasing zinc from the endoplasmic reticulum or from metallothionein. In this experiment, however, ZIP4 expression in the small intestinal mucosa must be increased because 2 days after the Zn-deficient diet feeding to the rat, which means 1 oral administration of Zn solution could be absorbed efficiently as reported previously [Citation29]. Therefore, absorbed Zn might be increased in the small intestinal cells and move to the blood circulation, so this free Zn2+ signaling may contribute to this mechanism, possibly through blood circulation or gastrointestinal vagus nerve signal transduction to the hypothalamus.
We conjecture that in the present experiments, oral zinc ingestion causes early zinc signaling in the gastrointestinal tract [Citation30], because an abrupt increase in food intake was observed after an oral zinc administration in our preceding paper as shown in Supplemental Figure 1, despite unchanged zinc concentrations in tissues (plasma and liver) between Zn-IP and Zn-PO groups (Supplemental Figure 2).
We found that our oral zinc-administration group showed increased concentrations of 3-aminopropanoic acid (β-alanine), hypotaurine, dopamine, and biotin. This study is the first to reveal changes in the metabolite profile in the hypothalamus relevant to food-intake regulation in the rat. We indicate directions for further research in light of metabolomic analysis of these results.
Authors’ contributions
MN, FK, FK, SH, TG, HS, and MK designed the experiments. MN and KS performed the experiments, and MN, KS, HS, and MK analyzed the data. MN, KS, and MK wrote the manuscript. All authors discussed the results, read, and approved the final version of the manuscript.
0821_Supplemental_fig_HS_MK.pdf
Download PDF (56.8 KB)Acknowledgments
The authors thank Minami Ooi, Naohiro Sodeyama, and Hisahiro Nakai for technical support.
Disclosure statement
No potential conflict of interest was reported by the authors.
Supplementary material
Supplemental data for this article accessed here.
Additional information
Funding
References
- Suzuki H, Asakawa A, Li JB, et al Zinc as an appetite stimulator - the possible role of zinc in the progression of diseases such as cachexia and sarcopenia. Recent Pat Food Nutr Agric. 2011;3:226‒231.
- Ohinata K, Takemoto M, Kawanago M, et al Orally administered zinc increases food intake via vagal stimulation in rats. J. Nutr. 2009;139:611‒616.
- Komai M, Goto T, Ohinata K, et al. Clarification of the mechanism involved in orexigenic action by oral zinc ingestion. Yakugaku Zasshi. 2018;138:1011‒1016. (in Japanese.)
- Selvais PL, Labuche C, Nguyen XN, et al Cyclic feeding behaviour and changes in hypothalamic Galanin and neuropeptide Y gene expression induced by zinc deficiency in the rat. J Neuroendocrinol. 1997;9:55‒62.
- Glowinski J, Iversen LL. Regional studies of catecholamines in the rat brain-1. J Neurochem. 1966;13:656–657.
- Paxinos G, Watson C. The rat brain in stereotaxic coordinates. USA: Academic Press; 1998.
- Bligh EG, Dyer WJ. A rapid method of total lipid extraction and purification. Can J Biochem Physiol. 1959;37:911‒917.
- Nishiumi S, Kobayashi T, Kawana S, et al Investigations in the possibility of early detection of colorectal cancer by gas chromatography/triple-quadrupole mass spectrometry. Oncotarget. 2017;2:1‒12.
- Wiklund S. Johansson E, Sjostrom L, et al. Visualization of GC/TOF-MS-Based Metabolomics Data for Identification of Biochemically Interesting Compounds Using OPLS Class Models. Anal Chem. 2008;80:115‒122.
- Morton GJ, Cummings DE, Baskin DG, et al Central nervous system control of food intake and body weight. Nature. 2006;443:289–295.
- Schwartz MW, Woods SC, Porte DJ, et al Central nervous system control of food intake. Nature. 2000;404:661‒671.
- Baltaci AK, Mogulkoc R. Leptin and zinc relation: in regulation of food intake and immunity. Indian J Endocrinol Metab. 2012;16(Suppl 3):S611–S616.
- Schetz JA, Sibley DR. Zinc allosterically modulates antagonist binding to cloned D1 and D2 dopamine receptors. J Neurochem. 1997;68:1990‒1997.
- Li Y, Mayer FP, Hasenhuetl PS, et al Occupancy of the zinc-binding site by transition metals decreases the substrate affinity of the human dopamine transporter by an allosteric mechanism. J Biol Chem. 2017;292:4235–4243.
- Li Y, Hasenhuetl PS, Schicker K, et al Dual action of Zn2+ on the transport cycle of the dopamine transporter. J Biol Chem. 2015;290:31069–31076.
- Sensi SL, Paoletti P, Bush AI, et al. Zinc in the physiology and pathology of the CNS. Nat Rev. 2009;10:780–791.
- Gao S, Kinzig KP, Aja S, et al. Leptin activates hypothalamic acetyl-CoA carboxylase to inhibit food intake. Proc Natl Acad Sci U S A. 2007;104:17358‒17363.
- Sone H, Kamiyama S, Higuchi M, et al Biotin augments acetyl CoA carboxylase 2 gene expression in the hypothalamus, leading to the suppression of food intake in mice. Biochem Biophys Res Commun. 2016;476:134‒139.
- Wei J, Zhang Y, Yu TY, et al A unified molecular mechanism for the regulation of acetyl-CoA carboxylase by phosphorylation. Cell Discov. 2016;2:16044.
- Wu K, Huang C, Shi X, et al Role and mechanism of the AMPK pathway in waterborne Zn exposure influencing the hepatic energy metabolism of Synechogobius hasta. Sci Rep. 2016;6:38716.
- Inui A, Asakawa A, Bowers CY. Ghrelin, appetite, and gastric motility: the emerging role of the stomach as an endocrine organ. FASEB J. 2004;3:439‒456.
- Minokoshi Y. Food intake regulation by hypothalamic AMPK. (In Japanese) Nihon Yakurigaku Zasshi. 2011;137:172‒176.
- Andrews ZB, Liu ZW, Walllingford N, et al UCP2 mediates ghrelin’s action on NPY/AgRP neurons by lowering free radicals. Nature. 2008;454:846‒851.
- Yin J, Li X, Li D, et al Dietary supplementation with zinc oxide stimulates ghrelin secretion from the stomach of young pigs. J Nutri Biochem. 2009;20:783‒790.
- Hiramatsu T. Beneficial effects of postoperative enteral nutrition on protein metabolism, immunocompetence and catabolic hormones. Nihon Shokaki Geka Gakkai Zasshi. 1990;23:1207‒1219.
- Nishiuchi M, Ohtani M, Konno A, et al Zinc supplementation for perioperative poor appetite of the patients who underwent open heart surgery or coronary artery bypass graft. Nihon Jōmyaku Keichō Eiyō Gakkai Zasshi. 2016;31:1136‒1140.
- Shimura M, Tsuchiya T. Changes of serum zinc levels in patients undergoing gastrointestinal surgery. Geka to Taisha, Eiyō. 2015;49:43‒51.
- Fukada T, Yamasaki S, Nishida K, et al Zinc homeostasis and signaling in health and diseases Zinc signaling. J Biol Inorg Chem. 2011;16:1123–1134.
- Hashimoto A, Kambe T, Komai M, et al. Properties of Zip4 accumulation during zinc deficiency and its usefulness to evaluate zinc status: a study of the effects of zinc deficiency during lactation. Am J Physiol Regul Integr Comp Physiol. 2016;310:R459–R468.
- Yamada T. Central nervous system regulates inter-organ communication involved in energy metabolism. Tonyobyo. The Journal of the Japan Diabetic Society. 2012;55:309–312.