ABSTRACT
Deep-sea Shewanella violacea 5′-nucleotidase (SVNTase) activity exhibited higher NaCl tolerance than that of a shallow-sea Shewanella amazonensis homologue (SANTase), the sequence identity between them being 70.4%. Here, SVNTase exhibited higher activity than SANTase with various inorganic salts, similar to the difference in their NaCl tolerance. In contrast, SVNTase activity decreased with various organic solvents, while SANTase activity was retained with the same concentrations of the solvents. Therefore, SVNTase is more robust than SANTase with inorganic salts, but more vulnerable with organic solvents. As to protein stability, SANTase was more stable against organic solvents and heat than SVNTase, which correlated with the differences in their enzymatic activities. We also found that SANTase retained higher activity for three weeks than SVNTase did in the presence of glycerol. These findings will facilitate further application of these enzymes as appropriate biological catalysts under various harsh conditions.
Abbreviations: NTase: 5′-nucleotidase; SANTase: Shewanella amazonensis 5′-nucleotidase; SVNTase: Shewanella violacea 5′-nucleotidase; CD: circular dichroism
ABSTRACT
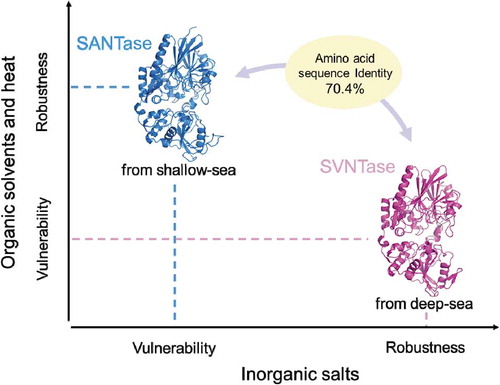
Differences in biochemical properties of SVNTase and SANTase.
Shewanella species are widely distributed in aquatic environments, from deep-sea to shallow-sea [Citation1]. They are also known to utilize a wide range of inorganic and organic compounds as nutrients, which makes these widely distributed species important players in biogeochemical cycles [Citation2]. Such diversity, in turn, has evoked our interest in using Shewanella enzymes as useful biological catalysts.
Enzymes from deep-sea Shewanella species are well known to be stable proteins. For example, monomeric dihydrofolate reductases and cytochromes c5 from deep-sea Shewanella species showed higher stability, compared with those of homologues from shallow-sea Shewanella species and other atmospheric bacteria [Citation3–Citation7]. Under high-pressure conditions, multimeric 3-isopropylmalate dehydrogenases and RNA polymerases from deep-sea Shewanella species exhibited higher activities than their homologues from shallow-sea Shewanella species and Escherichia coli [Citation8–Citation10].
5ʹ-Nucleotidases (NTases) degrade various nucleotides, such as 5ʹ-ATP, 5ʹ-AMP, 5ʹ-GTP, 5ʹ-GMP, and 5ʹ-IMP [Citation11,Citation12], some of which are umami compounds used for food seasoning. Thus, NTases with proper characteristics even under harsh conditions will be useful for food industry fields. Previously, we showed that deep-sea psychrophilic and piezophilic Shewanella violacea, and shallow-sea mesophilic and piezo-sensitive Shewanella amazonensis had homologous membrane-bound NTases, abbreviated here as SVNTase and SANTase, respectively [Citation12]. These enzymes exhibit 70.4% sequence identity (Figure S1), and thus comparative study will promote protein characterization in the aspects of stability, activity, and structure, because they are from obviously different environments. Our previous study showed that SVNTase and SANTase have the same activity properties in the aspects of the optimal pH and substrate specificity, while SVNTase activity is significantly more NaCl-tolerant than that of SANTase [Citation12].
In this study, we measured the ATPase activities of SVNTase and SANTase under various harsh conditions in order to examine their robustness and vulnerability. Their activities were compared in the presence of inorganic salts (other than NaCl) and organic solvents. The effects of these chemicals on their structures were also examined by circular dichroism (CD) spectra analyses. The findings obtained are discussed as to the activity, stability, and structure of the enzymes, which will be useful for their further application as biological catalysts.
Materials and methods
Enzyme preparation
Since our preliminary RNA-seq analysis of S. violacea DSS12 (JCM 10179) showed that SVNTase gene expression was more upregulated at pH 7.0 than at pH 5.0, the S. violacea cells for the enzyme preparation were grown at pH 7.0, as previously reported [Citation12]. The SANTase enzyme was prepared from S. amazonensis SB2B cells, also as described previously [Citation12]. The purity levels of SVNTase and SANTase, as checked by sodium dodecyl sulfate acrylamide gel electrophoresis, followed by quantification of stained band intensity with Quantity One Software installed in ChemiDoc (Bio-Rad, Japan), and resulting values were found to be 85 and 95%, respectively. These purity levels did not affect the thermal stability analysis by CD measurement in this study because data could be fitted in a single cooperation manner (see below). Protein concentrations during the enzyme preparation were determined by the method of Lowry et al. (1951) with bovine serum albumin as the standard [Citation13].
ATPase activity assay
ATPase activity was routinely measured according to the modified method of Fiske and Subbarow (1925) [Citation14]. The effects of inorganic salts (KCl, MgCl2, MnCl2, LiCl, CaCl2, CoCl2, CsCl, NiCl2, CdCl2, CuCl2, and ZnCl2) and organic solvents (glycerol, dimethyl sulfoxide, N,N-dimethyl formamide, methanol, ethanol, 2-propanol, acetonitrile, and acetone) on the enzymatic activity were examined. Enzymatic activity after long-term storage was also assayed by pre-incubating an enzyme stock solution in a block heater set at 30 and 4°C for 21 days, with or without 10% (v/v) glycerol [Citation15]. All of the reaction mixtures (0.6 mL), comprising 1.0 μg purified enzyme, 20 mM Tris-HCl (pH 8.0), 10 mM MgCl2, and 0.6 μg bovine serum albumin, were incubated with 4 mM disodium ATP for 10 min at 30°C, and the reaction was terminated by the addition of trichloroacetic acid (0.33 M, final concentration). ATPase activity was calculated by means of a colorimetric assay, at an absorbance of 740 nm using a UV-2600 spectrophotometer (Shimadzu, Japan), which estimated the amount of Pi liberated from ATP. One unit of ATPase activity was defined as the amount of enzyme that liberated 1 μmol of Pi per min.
CD measurement and thermal stability analysis
Far-UV CD spectra (200–250 nm) of the purified SVNTase and SANTase (2 μM in 10 mM Tris-HCl, pH 8.0) in the presence of various inorganic salts and organic solvents were measured to examine the α-helix content using a JASCO J-820 spectrometer with a cuvette of 1 mm path-length at 25°C. The helix content was calculated from the CD data by means of the server BeStSel [Citation16,Citation17]. The server fitted the CD experimental curve by linearly combining fixed basis components to obtain the α-helix content as a percentage.
Thermal denaturation experiments were carried out by obtaining CD spectra in a pressure-proof cell compartment (Jasco, Japan) attached to a JASCO J-820 CD spectrometer, as described previously [Citation18]. The purified SVNTase and SANTase (20 μM) in solutions of 20 mM potassium phosphate (pH 8.0) were analyzed. The temperature-dependent CD ellipticity change at 222 nm, reflecting the existence of the α-helix structure, was monitored in a cuvette of 1 mm path-length. CD values were recorded from 25 to 110°C at intervals of 0.5°C at a heating rate of 1.0 °C/min.
The raw data were statistically analyzed by nonlinear least-squares fitting, as described previously [Citation19]. The data points were corrected for the slopes of the baselines for the native and denatured forms, and were normalized to calculate the fraction of protein denatured. The fraction of protein denatured was plotted as a function of temperature, and the resulting thermal denaturation curves were used to determine the temperature at the midpoint of the transition (Tm). The calculation was performed by curve fitting of the resulting CD values versus the temperature by van’t Hoff analysis. The fitting curves obtained in the temperature range from –20 to +20°C of Tm were used to calculate thermodynamic parameters in order to reflect protein denaturation precisely.
Structure simulation
Since the amino acid sequences of SVNTase and SANTase were more than 50% identical to that of Escherichia coli NTase (ECNTase), their three-dimensional model structures could be successfully simulated using the crystal structure of substrate-bound ECNTase (PDB ID: 1HPU) as a template with the SWISS-MODEL workplace [Citation12,Citation20]. The simulated structures obtained were used for examination of differences in activity and stability between SVNTase and SANTase.
Results and discussion
Effects of inorganic salts on ATPase activity
The ATPase activities of SVNTase and SANTase were first measured in the presence of various inorganic salts. In the presence of basally added 10 mM MgCl2, the activity of SVNTase increased to approximately 120% with 500–2500 mM KCl compared with that with 0 mM KCl, which had been defined as the control (). In contrast, the activity of SANTase decreased to approximately 60% in the same concentration range of KCl compared to that with 0 mM. Similar to the case of the KCl effect, 50–500 mM MgCl2 and 2–10 mM MnCl2 increased the SVNTase activity, but decreased that of SANTase. Although the extents of the activity increase and decrease differed among these three inorganic salts, they reinforced the activity of SVNTase and inhibited that of SANTase. It should be noted that SVNTase activity increased two-fold with 500 mM MgCl2 (), this extent being similar to that observed for a salt-tolerant alginate lyase from Shewanella species YH1 with 300 mM MgCl2 [Citation15].
Figure 1. Effects of inorganic salts on ATPase activity.
Magenta and cyan bars represent mean relative values of ATPase activity obtained in three independent measurements (n = 3) for SVNTase and SANTase, respectively. Error bars indicate the standard deviation. The values for the controls (indicated by horizontal dashed lines) were obtained in the presence of 10 mM MgCl2 without other salts, and are regarded as 100% activity, where the specific activities of SVNTase and SANTase are 196.2 ± 20.4 and 184.8 ± 17.6 U/mg protein, respectively.
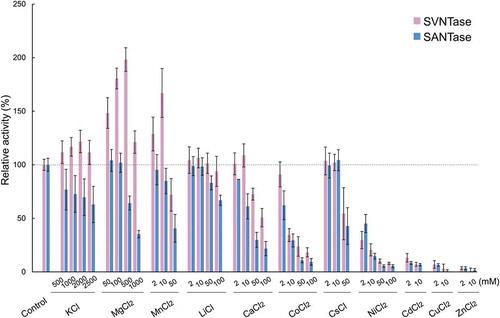
LiCl, CaCl2, and CoCl2 at 2–100, 2–10, and 2 mM, respectively, did not affect the SVNTase activity, but 50–100 mM LiCl, 10–100 mM CaCl2, and 2–100 mM CoCl2 significantly decreased the SANTase activity compared with the control (). These results together suggest that LiCl, CaCl2, and CoCl2 differently affect the activities of SVNTase and SANTase, as observed for the effects of KCl, MgCl2, and MnCl2 on both enzymes. Therefore, SVNTase is more robust than SANTase with inorganic salts such as KCl, MgCl2, MnCl2, LiCl, CaCl2, and CoCl2, as well as NaCl, and thus SVNTase appears to be more useful than SANTase as a biocatalyst with these inorganic salts.
In contrast, although the SVNTase and SANTase activities remained approximately 100% with 10 mM CsCl, they similarly decreased with 50 mM of the same salt (). In addition, 50 mM NiCl2, 2 mM CdCl2, 2 mM CuCl2, and 2 mM ZnCl2 had similarly critical effects on both activities, these four inorganic salts reducing them to less than approximately 10% of the control levels. Therefore, activities of SVNTase and SANTase were similarly affected by CsCl, NiCl2, CdCl2, CuCl2, and ZnCl2, although the extents of the effects differed among the salts.
Effects of inorganic salts on the α-helix structure
In order to correlate the effects of inorganic salts on the ATPase activities of SVNTase and SANTase with the α-helix structure, we measured CD spectra in the presence of several salts (Figure S2), and calculated the α-helix contents by means of the server BeStSel [Citation16,Citation17]. The control values measured in the absence of any inorganic salt showed that SVNTase and SANTase have a helix content of approximately 25% (), consistent with the values predicted from their amino acid sequences (Figure S1). In the presence of 2000 mM KCl, the helix contents of SVNTase and SANTase were significantly different, that of the latter being decreased compared with that of the control (). Therefore, the decrease in the SANTase activity with 2000 mM KCl, () correlated with the decrease in the helix content. A similar relationship between the helix content and activity of SVNTase and SANTase was observed with 2000 mM NaCl () [Citation12].
Figure 2. Effects of inorganic salts on the α-helix structure.
Magenta and cyan bars represent mean α-helix content values obtained in three independent measurements (n = 3) for SVNTase and SANTase, respectively. Error bars indicate the standard deviation. Asterisks represent significant difference (p < 0.05). The values for the controls were obtained in the absence of an inorganic salt.
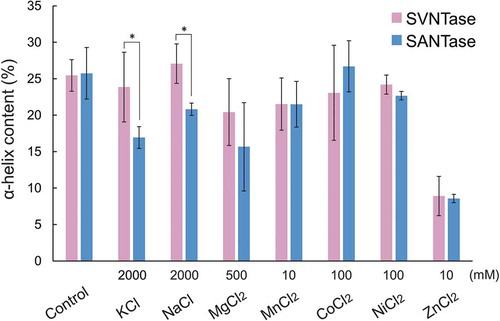
With 500 mM MgCl2, the helix contents in SVNTase and SANTase decreased to approximately 20 and 15%, respectively, which were not significantly different (), while the SVNTase and SANTase activities increased to approximately 200% and decreased to approximately 60%, respectively, which were obviously significantly different (). As both enzymes require Mg2+ for their catalysis [Citation12] and only 4 mM disodium ATP was added in the present activity assay, both enzymes appeared to be surrounded by an excess amount of free Mg2+ in the reaction mixtures with 500 mM MgCl2. Therefore, our present results indicate that excess free Mg2+ may similarly affect the helix structures of both enzymes, but only enhance the SVNTase activity through an undefined protein structure stabilization mechanism, which often occurs for halophilic or halo-tolerant enzymes [Citation21,Citation22].
Similar results for SVNTase and SANTase were obtained with 10 mM MnCl2; virtually the same helix contents, approximately 20% (), and a prominent activity difference in the presence of basally added 10 mM MgCl2 (). Two Mn2+ ions are incorporated into the catalytic site in ECNTase [Citation23], which exhibits ATPase activity with Mn2+, but not with Mg2+ [Citation24]. In contrast, because SVNTase and SANTase exhibited ATPase activity with 10 mM MnCl2 or 10 mM MgCl2 [Citation12], both Mn2+ and Mg2+ could be incorporated into the catalytic sites of their simulated structures as divalent metal cations (). From the present experimental results and relevant consideration, the existence of free Mg2+ or Mn2+ that were not bound to the catalytic sites of both enzymes during the present activity assay enhanced SVNTase activity but not that of SANTase, presumably similar to the case with 500 mM MgCl2.
Figure 3. Catalytic sites in simulated structures of SVNTase and SANTase.
The catalytic sites are enlarged from the superimposed whole structures of SVNTase (magenta) and SANTase (cyan) shown as a ribbon model. Two divalent metal cations (grey) and an ATP analog, phosphomethylphosphonic acid adenosyl ester (black), are shown as sphere and stick models, respectively. Amino acid side chains in the catalytic sites of SVNTase (magenta) and SANTase (cyan) are also shown as stick models.
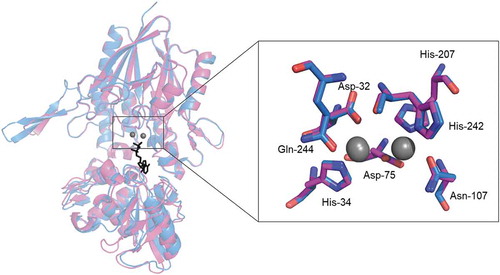
Even with 100 mM CoCl2 and NiCl2, the helix contents of the two enzymes were not significantly affected (), while the same concentrations of these salts resulted in reduction of the ATPase activity to approximately 10% of the control levels (). These results indicated that these salts have deleterious effects on the enzyme catalysis, but not on the helix structure. One possible explanation is that Co2+ and Ni2+, generally binding with His residues [Citation25], may bind with specific His-34, His-207, and His-242 residues conserved in the catalytic sites of the two enzymes (), resulting in the occupancy by these cations instead of Mg2+ essential for the catalysis.
The presence of 10 mM ZnCl2 resulted in reduction of the helix content in both enzymes to approximately 10% (), and both ATPase activities became less than 10% of the control levels with the same concentration of this salt (). Zn2+ disrupted two-thirds of the helix structures of both enzymes (), which may lead to the decreases in their activities. If Zn2+, which has been shown to occupy the catalytic site of ECNTase [Citation26], was in the catalytic sites of SVNTase and SANTase instead of Mg2+, the decreases in the activities observed () were consistent with our previous results; Zn2+ did not contribute to the activities of SVNTase and SANTase as a divalent metal cation [Citation12].
Effects of organic solvents on ATPase activity
In order to examine the robustness and vulnerability of SVNTase and SANTase, their ATPase activities were further measured in the presence of various organic solvents. In the presence of 10% (v/v) glycerol, the activities of both enzymes were more than 80% of the control levels, and similarly decreased to less than 10% with 30% (v/v) glycerol ().
Figure 4. Effects of organic solvents on ATPase activity.
Magenta and cyan bars represent mean relative values of ATPase activity obtained in three independent measurements (n = 3) for SVNTase and SANTase, respectively. Error bars indicate the standard deviation. The values for the controls (indicated by horizontal dashed lines) were obtained in the presence of 10 mM MgCl2 without organic solvents, and are regarded as 100% activity, where the specific activities of SVNTase and SANTase are 196.2 ± 20.4 and 184.8 ± 17.6 U/mg protein.
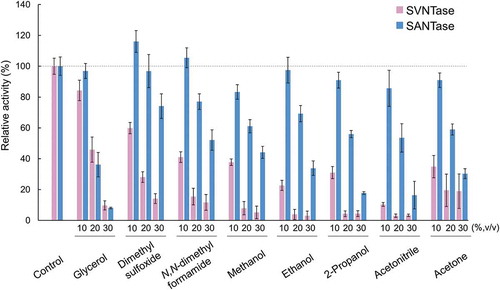
In contrast, with 10% (v/v) of all the other organic solvents tested, dimethyl sulfoxide, N,N-dimethyl formamide, methanol, ethanol, 2-propanol, acetonitrile, and acetone, the SVNTase activity drastically decreased, but the SANTase activity was retained or decreased only slightly (). With 20–30% (v/v) of these organic solvents, the SVNTase activity was significantly lower than that of SANTase. These results indicated that the SVNTase activity was very vulnerable to organic solvents compared with that of SANTase, which was the reverse of their activities in the presence of inorganic salts such as KCl, MgCl2, MnCl2, LiCl, CaCl2, and CoCl2, as well as NaCl.
Effects of organic solvents on the α-helix structure
Similar to the case of the experiments with inorganic salts, in order to correlate the effects of organic solvents on the ATPase activities of SVNTase and SANTase with those on the α-helix structure, we measured CD spectra in the presence of some of the organic solvents examined (Figure S3) to calculate the α-helix contents by the means of the server BeStSel [Citation16,Citation17]. The control values were obtained under conditions exactly the same as those used in . In the presence of 30% (v/v) methanol, ethanol, 2-propanol, and acetonitrile, the helix contents of SVNTase were significantly reduced compared with the control levels, while those of SANTase were more or less the same as that of the control levels (). Therefore, the higher activity of SANTase than that of SVNTase in the presence of the organic solvents observed above () correlated well with the robustness of the helix structure of the SANTase compared with that of SVNTase.
Figure 5. Effects of organic solvents on the α-helix structure.
Magenta and cyan bars represent mean α-helix content values obtained in three independent measurements (n = 3) for SVNTase and SANTase, respectively. Error bars indicate the standard deviation. Asterisks represent significant differences (p < 0.05). The values for the controls were obtained in the absence of an organic solvent.
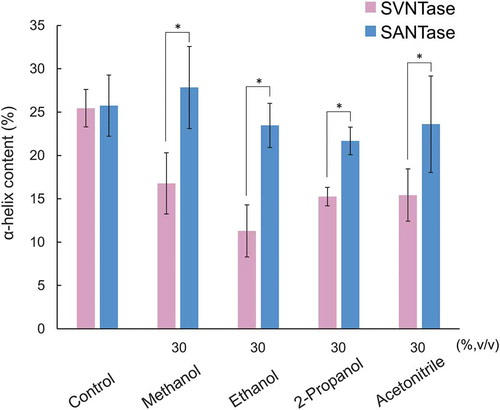
Thermal stability as measured by CD spectra
The differences in the stability robustness and vulnerability of the α-helix structure between SVNTase and SANTase against heat were further examined by means of CD spectra at 222 nm (). Three independent experiments revealed that the denaturation temperatures for SANTase and SVNTase were 88.2 ± 0.6 and 77.1 ± 0.6°C, respectively, indicating that SANTase is more stable against heat than SVNTase, which correlates with the temperature dependency of their activities [Citation12], and with the optimal growth temperatures of the source bacteria [Citation27,Citation28]. Therefore, in comparison with SVNTase, SANTase maintains its native structure not only in the presence of organic solvents, but also under higher temperature conditions.
Structural insight into the robustness of SANTase against organic solvents and heat as compared with SVNTase
On the molecular surfaces of SVNTase and SANTase in the simulated structures (), amino acid residues were different in terms of hydrophobicity and hydrophilicity: Thr-52, Leu-97, Ala-215, Ala-232, Ala-236, Ala-254, Val-269, Leu-336, Glu-348, Leu-351, Ala-366, Glu-381, Glu-404, Ala-478, Thr-502, Met-505, Leu-525, and Ala-530 in SVNTase and corresponding Ala-52, Lys-97, Gln-215, Lys-232, Gln-236, Asn-254, Gln-269, Lys-336, Ala-348, Asp-351, Thr-366, Met-381, Ala-404, Asp-478, Ala-502, Gln-505, Lys-525, and Asn-530 in SANTase (see also Figure S1). Of these eighteen residues, thirteen in SVNTase, Leu-97, Ala-215, Ala-232, Ala-236, Ala-254, Val-269, Leu-336, Leu-351, Ala-366, Ala-478, Met-505, Leu-525, and Ala-530 are hydrophobic, while the corresponding ones in SANTase are hydrophilic. In contrast, only five residues, Thr-52, Glu-348, Glu-381, Glu-404 and Thr-502 in SVNTase are hydrophilic, while the corresponding ones in SANTase are hydrophobic. This comparison indicates that the molecular surface of SVNTase is more hydrophobic than that of SANTase. Therefore, in the presence of organic solvents, the hydrophobic clustering force on the molecular surface is stronger in SVNTase than in SANTase, which may result in the vulnerability of SVNTase and the robustness of SANTase as to organic solvents. In addition, the higher number of surface hydrophobic residues in SVNTase may cause its instability during heat denaturation compared with SANTase, as discussed previously for other proteins [Citation29].
Figure 7. Surface residues that differ between SVNTase and SANTase in terms of hydrophobicity and hydrophilicity.
Main chain structures (ribbon model) of SVNTase (magenta) and SANTase (cyan) are shown. Eighteen hydrophobic and hydrophilic side chains on the molecular surfaces are shown as sphere and stick models, respectively.
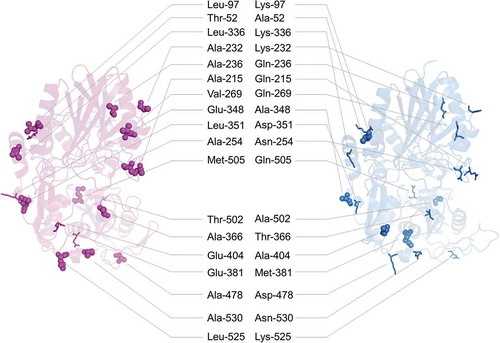
SVNTase and SANTase have nine and fourteen potential salt bridges on their molecular surfaces, respectively [Citation12]. The higher number of potential salt bridges in SANTase than that in SVNTase may also be responsible for the higher stability against organic solvents and heat of SANTase. Therefore, SANTase is more robust against organic solvents and heat than SVNTase. These findings regarding salt bridges are consistent with the results for cold shock proteins, Rhodococcus opacus ene-reductases, and Stenotrophomonas maltophilia lipase [Citation30–Citation32].
Long-term storage
To assess the enzymatic activities of SVNTase and SANTase after long-term storage, the enzyme stock solutions were incubated at 30 and 4°C for 21 days, with or without 10% (v/v) glycerol, and then the ATPase activities were measured at 30°C by routine methods. After storage at 30°C up to 21 days without glycerol, the ATPase activities of SVNTase and SANTase gradually decreased to approximately 10 and 30%, respectively, compared with fresh samples of them ()). At the same temperature, in the presence of 10% (v/v) glycerol, the SVNTase and SANTase activities decreased to approximately 30 and 50%, respectively. These results indicate that SANTase is more suitable as to long-term storage compared with SVNTase, and the activity reduction can be mitigated by the addition of glycerol during storage. In addition, 70% SANTase activity was retained after storage at 4°C for 21 days with glycerol ()). Overall, SANTase is advantageous for long-term storage compared with SVNTase, which should be considered in further application studies.
Figure 8. Long-term storage of SVNTase and SANTase at 30°C (a) and at 4°C (b).
Magenta and cyan bars represent mean relative values of ATPase activity obtained in three independent measurements (n = 3) for SVNTase and SANTase, respectively. Error bars indicate the standard deviation. The values for day 0 were obtained in the presence of 10 mM MgCl2 without glycerol, and are regarded as 100% activity, where the specific activities of SVNTase and SANTase are 196.2 ± 20.4 and 184.8 ± 17.6 U/mg protein, respectively.
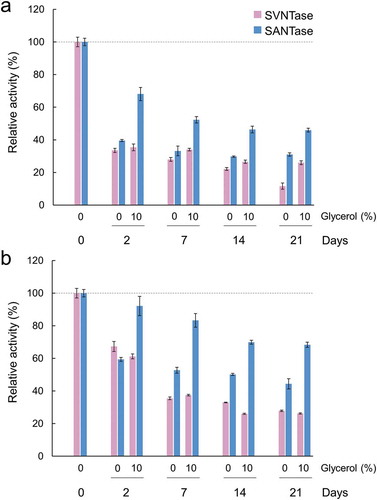
Conclusion
The aim of this study was to find biochemical differences between SVNTase and SANTase. As expected from our previous activity measurements regarding NaCl tolerance [Citation12], SVNTase exhibited higher activity than SANTase in the presence of other inorganic salts. Therefore, SVNTase will be useful with inorganic salts, compared with SANTase, as a biocatalyst. An unanticipated observation in the present study was that SANTase exhibited higher activity in the presence of various organic solvents than SVNTase did, which is consistent with the robustness of the α-helix structure of SANTase, not only against the organic solvents but also heat. The robustness of SANTase may result in the advantage as to long-term storage compared with SVNTase. These findings together facilitate further application of these enzymes as appropriate biological catalysts under various harsh conditions.
Author contributions
K.F. conducted most of the experiments, analyzed the results with the aid of S.F., L.L., S.W., H.Y., and Y.S., who conceived the idea for the project, and wrote the paper with K.F. All the authors reviewed the results and approved the final version of the manuscript.
190128_NTase_Sup_Figs.docx
Download MS Word (438.9 KB)Acknowledgments
We would like to thank Haruka Yata and Taka-aki Kuribayashi for their technical support.
Disclosure statement
No potential conflict of interest was reported by the authors.
Supplementary material
Supplemental data for this article can be accessed here.
Additional information
Funding
References
- Hau HH, Gralnick JA. Ecology and biotechnology of the genus Shewanella. Annu Rev Microbiol. 2007;61:237–258.
- Falkowski PG, Fenchel T, Delong EF. The microbial engines that drive Earth’s biogeochemical cycles. Science. 2008;320:1034–1039.
- Murakami C, Ohmae E, Tate S, et al. Cloning and characterization of dihydrofolate reductases from deep-sea bacteria. J Biochem. 2010;147:591–599.
- Murakami C, Ohmae E, Tate S, et al. Comparative study on dihydrofolate reductases from Shewanella species living in deep-sea and ambient atmospheric-pressure environments. Extremophiles. 2011;15:165–175.
- Masanari M, Wakai S, Ishida M, et al. Correlation between the optimal growth pressures of four Shewanella species and the stabilities of their cytochromes c5. Extremophiles. 2014;18:617–627.
- Masanari M, Fujii S, Kawahara K, et al. Comparative study on stabilization mechanism of monomeric cytochrome c5 from deep-sea piezophilic Shewanella violacea. Biosci Biotechnol Biochem. 2016;80:2365–2370.
- Fujii S, Masanari-Fujii M, Kobayashi S, et al. Commonly stabilized cytochromes c from deep-sea Shewanella and Pseudomonas. Biosci Biotechnol Biochem. 2018;82:792–799.
- Kawano H, Nakasone K, Matsumoto M, et al. Differential pressure resistance in the activity of RNA polymerase isolated from Shewanella violacea and Escherichia coli. Extremophiles. 2004;8:367–375.
- Kato C, Sato T, Abe F, et al. Discoveries of deep-sea piezophiles, and their pressure adapted enzymes. Proc 4th Int Conf High Pressure Biosci Biotechnol. 2007;1:114–121.
- Kasahara R, Sato T, Tamegai H, et al. Piezo-adapted 3-isopropylmalate dehydrogenase of the obligate piezophile Shewanella benthica DB21MT-2 isolated from the 11,000-m depth of the Mariana trench. Biosci Biotechnol Biochem. 2009;73:2541–2543.
- Sakai Y, Toda K, Mitani Y, et al. Properties of the membrane-bound 5ʹ-nucleotidase and utilization of extracellular ATP in Vibrio parahaemolyticus. J Gen Microbiol. 1987;133:2751–2757.
- Kuribayashi TA, Fujii S, Masanari M, et al. Difference in NaCl tolerance of membrane-bound 5ʹ-nucleotidases purified from deep-sea and brackish water Shewanella species. Extremophiles. 2017;21:357–368.
- Lowry OH, Rosebrough NJ, Farr AL, et al. Protein measurement with the folin phenol reagent. J Biol Chem. 1951;193:265–275.
- Fiske CH, Subbarow Y. The colorimetric determination of phosphorus. J Biol Chem. 1925;66:375–400.
- Yagi H, Isobe N, Itabashi N, et al. Characterization of a long-lived alginate lyase derived from Shewanella species YH1. Mar Drugs. 2017;16:E4.
- Micsonai A, Wien F, Kernya L, et al. Accurate secondary structure prediction and fold recognition for circular dichroism spectroscopy. Proc Natl Acad Sci USA. 2015;112:E3095–E3103.
- Micsonai A, Wien F, Bulyáki É, et al. BeStSel: a web server for accurate protein secondary structure prediction and fold recognition from the circular dichroism spectra. Nucleic Acids Res. 2018;46:W315–W322.
- Fujii S, Masanari M, Inoue H, et al. High thermal stability and unique trimer formation of cytochrome c’ from thermophilic Hydrogenophilus thermoluteolus. Biosci Biotechnol Biochem. 2013;77:1677–1681.
- Uchiyama S, Ohshima A, Nakamura S, et al. Complete thermal-unfolding profiles of oxidized and reduced cytochromes c. J Am Chem Soc. 2004;126:14684–14685.
- Biasini M, Bienert S, Waterhouse A, et al. SWISS-MODEL: modelling protein tertiary and quaternary structure using evolutionary information. Nucleic Acids Res. 2014;42:W252–W258.
- Lanyi JK. Salt-dependent properties of proteins from extremely halophilic bacteria. Bacteriol Rev. 1974;38:272–290.
- Wakai S, Abe A, Fujii S, et al. Pyrophosphate hydrolysis in the extremely halophilic archaeon Haloarcula japonica is catalyzed by a single enzyme with a broad ionic strength range. Extremophiles. 2017;21:471–477.
- Knöfel T, Sträter N. Mechanism of hydrolysis of phosphate esters by the dimetal center of 5ʹ-nucleotidase based on crystal structures. J Mol Biol. 2001;309:239–254.
- Neu HC. The 5′-nucleotidase of Escherichia coli. I. purification and properties. J Biol Chem. 1967;242:3896–3904.
- Pathak RK, Dessingou J, Rao CP. Multiple sensor array of Mn2+, Fe2+, Co2+, Ni2+, Cu2+, and Zn2+ complexes of a triazole linked imino-phenol based calix[4]arene conjugate for the selective recognition of Asp, Glu, Cys, and His. Anal Chem. 2012;84:8294–8300.
- Knöfel T, Sträter N. X-ray structure of the Escherichia coli periplasmic 5ʹ-nucleotidase containing a dimetal catalytic site. Nat Struct Biol. 1999;6:448–453.
- Nogi Y, Kato C, Horikoshi K. Taxonomic studies of deep-sea barophilic Shewanella strains and description of Shewanella violacea sp. nov. Arch Microbiol. 1998;170:331–338.
- Venkateswaran K, Dollhopf ME, Aller R, et al. Shewanella amazonensis sp. nov., a novel metal-reducing facultative anaerobe from amazonian shelf muds. Int J Syst Bacteriol. 1998;48:965–972.
- Lee CF, Makhatadze GI, Wong KB. Effects of charge-to-alanine substitutions on the stability of ribosomal protein L30e from Thermococcus celer. Biochemistry. 2005;44:16817–16825.
- Perl D, Mueller U, Heinemann U, et al. Two exposed amino acid residues confer thermostability on a cold shock protein. Nat Struct Biol. 2000;7:380–383.
- Riedel A, Mehnert M, Paul CE, et al. Functional characterization and stability improvement of a ‘thermophilic-like’ ene-reductase from Rhodococcus opacus 1CP. Front Microbiol. 2015;6:1073.
- Wu JP, Li M, Zhou Y, et al. Introducing a salt bridge into the lipase of Stenotrophomonas maltophilia results in a very large increase in thermal stability. Biotechnol Lett. 2015;37:403–407.