ABSTRACT
High temperature (HT) during the grain developing stage causes deleterious effects on rice quality resulting in mature grains with a chalky appearance. Phospholipase D (PLD) plays an important role in plants, including responses to environmental stresses. OsPLDα1, α3 and β2-knockdown (KD) plants showed decreased production of chalky grains at HT. HT ripening increased H2O2 accumulated in the developing grains. However, the increase was canceled by the knockdown of OsPLDβ2. Expression levels of OsCATA which is one of three rice catalase genes, in developing grains of OsPLDβ2-KD plants at 10 DAF were increased compared with that in vector-controls in HT growth conditions. Overexpression of OsCATA markedly suppressed the production of chalky grains in HT growth conditions. These results suggested that OsPLDβ2 functions as a negative regulator of the induction of OsCATA and is involved in the production of chalky grains in HT growth conditions.
Graphical Abstract

High temperature during the grain developing stage causes production of chalky grains in rice. OsPLDα1, α3 and β2-knockdown plants showed decreased the production
High temperature (HT) during the grain-filling stage causes destructive effects of crop products. HT impairs rice grains filling by inhibiting the deposition of storage starch, resulting in mature grains with a chalky appearance [Citation1–Citation3]. It is well known that heat stress decreases the activity of starch synthesis enzymes [Citation4,Citation5] and changes the levels of gene expression associated with starch metabolism [Citation2]. In addition, several mutants whose grains showed a chalky phenotype have been reported [Citation6–Citation10]. Recently, it has been reported that RNAi-mediated suppression of α-amylase genes resulted in an improvement in chalky grain production in HT conditions [Citation11]. Thus, several α-amylases, which are starch-hydrolyzing enzymes, were suggested to be involved in HT-triggered grain chalkiness [Citation11]. Furthermore, a superoxide dismutase (SOD), which converts superoxide radicals into hydrogen peroxide (H2O2), was also suggested to be involved in HT-triggered grain chalkiness [Citation12]. The grain appearance quality of rice plants with constitutive overexpression of the SOD in HT conditions was significantly better than that of wild-type plants [Citation12]. In addition, accumulation of H2O2 in developing grains in HT growth conditions has also been reported previously [Citation13]. These lines of evidence suggested the critical role of reactive oxygen species (ROS) in the control of grain appearance.
Phospholipase D (PLD) is a representative phospholipase in plants, and it hydrolyzes phospholipids to generate phosphatidic acid (PA) and a free-head group. In Arabidopsis (Arabidopsis thaliana), 12 PLD genes have been reported, and they can be classified into six types, PLDα (3), β (2), γ (3), δ, ε, and ζ (2) [Citation14]. In rice (Oryza sativa), 17 PLD genes were classified into eight types, PLDα (5), β (2), δ (3), ε, η (2), θ, ζ (2), and ι [Citation15]. In recent years, PLD has been suggested to be involved in many plant cellular processes. In Arabidopsis, AtPLDαɩ has been reported to be involved in responses to abscisic acid (ABA) [Citation16], ethylene [Citation17], drought [Citation18], freezing [Citation19], salt stress [Citation20], and ROS generation [Citation21]. AtPLDδ has also been reported to be involved in responses to dehydration [Citation22], freezing, UV irradiation [Citation23], H2O2-siganal [Citation24,Citation25], ethylene [Citation26], nitric oxide [Citation27], and microtubule reorganization [Citation28,Citation29]. In rice, it has been reported that OsPLDα1 is involved in responses to salt tolerance [Citation30] and OsPLDα4 and α5 in herbivore-induced defense [Citation31]. OsPLDβ1 has been reported to be involved in defense responses and increased disease resistance [Citation15] and ABA signaling [Citation32]. Previously, the accumulation of phospholipids and an increase in lipase activity had been reported in developing seeds [Citation33]. Thus, PLD might have important roles in HT stress response during grain development.
In the present study, we propose another important aspect of PLD in the stress responses of developing seeds and we found the up-regulation of PLD activity in grains in HT growth conditions after flowering. We report here that the knockdown (KD) of each of PLD gene, OsPLDα1, OsPLDα3, and OsPLDβ2, resulted in improvement of chalky grain production in HT growth conditions. In addition, the accumulation of ROS in HT conditions was markedly suppressed in the grains of OsPLDβ2-KD plants compared with that in vector-control plants. Furthermore, the overexpression of a rice catalase gene, which is up-regulated in OsPLDβ2-KD plants in HT growth conditions, resulted in a reduction in chalky grains by HT. These results clearly indicated that OsPLDβ2 is crucial for controlling ROS-scavenging enzymes such as a catalase at HT. Its suppression deregulated the scavenging enzyme to clear harmful ROS, thus preventing grain chalkiness. The relationship between ROS and chalky grains production is also discussed.
Materials and methods
Plant materials and growth conditions
Rice (Oryza sativa L., cultivar “Nipponbare”) plants were used in this study. Seeds were surface-sterilized and germinated for 2 weeks on Murashige-Skoog (MS) agar medium. In the case of transgenic plants, 25 mg L−1 of hygromycin was added in the medium. The resulting plants were transferred to soil and grown in a greenhouse or plant incubators. In the greenhouse, each plant was grown in normal conditions (27°C for 12 h/25°C for 12 h, solar radiation) and 5 DAF, plants were moved to HT conditions (33°C for 12 h/29°C for 12 h, solar radiation). In plant incubators, plants were grown in normal conditions (27°C for 12 h light/22°C for 12 h dark) and 5 DAF, plants were shifted to HT conditions (33°C for 12 h light/28°C for 12 h dark). The light cycle program was fixed in all experiments: 12 h with maximum fluorescent light (40w x 15) and high-power light (1,500 μmol photons s−1 m−2 at highest) and 12 h dark. At 5, 10, 15, 20, and 30 DAF, an aliquot of developing grains was detached from the panicle, immediately frozen in liquid nitrogen, and stored at −80°C until analysis and used for determination of enzymatic activity and transcript levels.
Evaluation of rice grain appearance
The appearance of matured grains was evaluated visually in accordance with the method by Ishimaru [Citation3]. The chalky grains matured under HT in this study have at least some areas of chalkiness including both the white-cored/milky-white and the white-based/white-back type chalkiness.
DNA constructs and rice transformation
To make KD plants for eight PLD genes [Citation15] by introducing gene-specific RNAi constructs, part of each cDNA was amplified by PCR with KpnI and XbaI-SacI restriction sites at the 5ʹ and 3ʹ ends, respectively, and inserted into the corresponding sites of a pZH2Bik binary vector, in which an inverted repeat trigger RNA was transcribed using a rice ubiquitin promoter (OsmUbiP) [Citation34]. To ensure the specificity, the 3ʹ non-coding region, the sequences of which were different for each PLD and catalase gene, was used (Table S1) and inserted into the vector. The empty vector, pZH2Bik, was also used to generate vector-control plants. To make overexpression plants for the three catalase genes, a fragment of the full-length catalase cDNA was inserted between the XbaI and BglII sites of pZH2B, in which the introduced gene was driven by OsmUbiP [Citation34]. The empty vector, pZH2B, was also used to generate vector-control plants.
Agrobacterium tumefaciens strain EHA101 [Citation35] carrying the above vectors was used to transform rice cv. Nipponbare. Agrobacterium-mediated transformation was performed in accordance with the method by Toki [Citation36]) using vigorously growing callus derived from mature embryos. Transformed calli were selected on MS agar medium containing 25 mg L−1 of hygromycin. Regenerated plants were grown in a greenhouse and T0 transgenic seeds were harvested 40-day after flowering. T1 transgenic plants carrying the transgene were selected by germinating seeds on MS agar medium containing 25 mg L−1 of hygromycin for 2 weeks, and then selected plants were transferred to soil and were grown in a greenhouse (25–30°C, solar radiation).
Real-time PCR
Total RNA for first-strand cDNA synthesis was extracted using an RNeasy Plant Mini Kit (Qiagen, Hilden, Germany) in accordance with the manufacturer’s instructions. The isolated RNA (5 μg) was reverse-transcribed (SuperScript II, Invitrogen) using an oligo-dT13 primer. An aliquot of the first-strand cDNA mixture corresponding to 10 ng of total RNA was used as a template for real-time PCR analysis. Reactions were carried out using a Thermal Cycler Dice Real Time System III (Takara Bio Inc.) with SYBR premix Ex Taq (Takara Bio Inc.) in accordance with the manufacturer’s instructions. The gene-specific primers for each gene are shown in Table S2. A calibration curve for each gene was obtained by performing real-time PCR with several dilutions of the cloned cDNA fragment. The specificity of the individual PCR amplification was checked using a heat dissociation protocol from 65°C to 95°C after the final cycle of the PCR. The results obtained for the different cDNAs were standardized using the expression level of the rice polyubiquitin gene, RUBIQ1 [Citation37].
Determination of H2O2 accumulation and scavenging activity
Three developing grains harvested at 10 DAF were weighed and macerated using a Multi-beads Shocker (MB301, Yasui Kikai Co., Osaka, Japan) after being frozen in liquid nitrogen, and then suspended in 5% (w/v) aqueous trichloroacetic acid containing EGTA (10 mM). The extract was centrifuged at 10,000g at 4°C for 10 min, and the amount of H2O2 in the supernatant was determined using the luminol chemiluminescence method [Citation38]. Next, 50 μL of the supernatant added to 800 μL of 50 mM phosphate-KOH buffer, pH 7.9, immediately followed by the injection of 50 μL of luminol (5-amino-2,3-dihydro-1,4-phthalazinedione, 0.195 mg ml−1) and 100 μL of potassium hexacyanoferrate (III) (4.6 mg ml−1). H2O2 accumulation was measured by the chemiluminescence of luminol using a photon counting luminometer (ATP-300, Advantec Tokyo, Japan). Standard solution of H2O2 (1–10 μM) was used to make a calibration curve. ROS scavenging activity was determined using 10 mM H2O2 as a substrate. The grains were macerated as described above and suspended in 0.5 mL of 25 mM Tris-HCl buffer (pH 7.5) containing 10 mM EGTA, 1 mM dithiothreitol (DTT), 1 mM phenylmethyl sulfonyl fluoride (PMSF), and 0.3 M sucrose on ice. The extract was centrifuged at 10,000g at 4°C for 10 min, and the supernatant was stored as the cellular extract fraction at −20°C until analysis. Reactions performed at 37°C, for 10 min, and the amount of H2O2 in the supernatant was analyzed using the same methods as described above.
Results
Suppression of OsPLDα1, OsPLDα3, or OsPLDβ2 reduced the production of chalky grains by high temperature
Previously, we generated KD plants for each of seven PLD isoforms, OsPLDa1, a3, α4, α5, β1, β2, δ2, and ε, whose accession numbers are shown in Table S1 and S2, by RNA interference (RNAi) [Citation15]. To elucidate the function of each PLD in HT-triggered grain chalkiness, we analyzed the grain chalkiness of seven rice PLD genes-KD plants in HT growth conditions. The OsPLDα1-, α3-, and β2-KD plants reduced chalky grain production compared with the vector-control plants (). In contrast, the OsPLDα4- and α5-KD plants showed increased production of chalky grains in HT growth conditions (data not shown). More than 10 KD lines were established for each gene, and at least five independent lines were used for the analysis. Quantitative analysis of OsPLDα1, OsPLDα3, and OsPLDβ2 mRNA in the grains confirmed a clear suppression of each gene expression ()). Five independent KD-lines gave similar results (Fig. S1). No difference in the grain weight was observed between vector-control and OsPLDβ2-KD plants in normal growth conditions. But the grain weight of vector-control plants in HT growth conditions was slightly lower than that in normal growth conditions (Table S3).
Figure 1. Changes in chalky grain production of the PLD gene-KD plants in HT growth conditions.
(a) Each plant was grown in a greenhouse in normal conditions (27°C/25°C) before flowering, and then it was grown in HT conditions (33°C/29°C). Values represent the ratio of chalky grains and average of triplicate experiments of two individual plants, and the error bars indicate SD. The symbols, *, **, and *** indicate a significant difference from the control (HT) at the 0.2%, 0.03%, and 0.01% levels, respectively, as determined by using Student’s t-test. (b) Appearance of grains. (c) Accumulation of OsPLDα1, OsPLDα3, or OsPLDβ2 mRNAs in the grains of the OsPLDα1-KD, OsPLDα3-KD, OsPLDβ2-KD, or vector-control plants in HT growth conditions. Homozygote KD and vector-control plants were grown in a greenhouse, and after flowering they were grown in HT conditions. Total RNA was extracted from grains at 10 DAF. The values were standardized using the expression level of a rice polyubiqutin gene (RUBIQ1). Values represent the averages of three individual plants, and the error bars indicate SD.
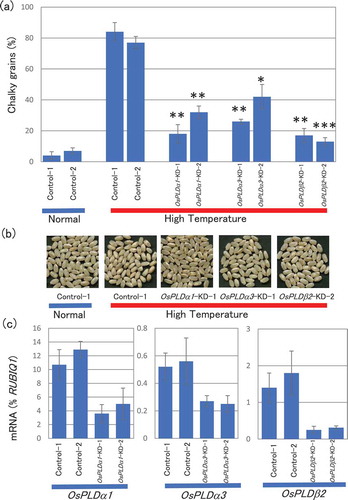
Suppression of OsPLDβ2 showed decreased production of ROS in developing grains in HT conditions
Because OsPLDβ2-KD plants showed a marked improvement in chalky grain production (), we performed further examinations using OsPLDβ2-KD plants. Because PLD has been reported to be involved in ROS generation [Citation15,Citation21,Citation39,Citation40], we examined ROS production in developing grains in HT growth conditions. In vector-control plants, HT increased H2O2 production by approximately 4-fold at 10 DAF. This increase was not observed in OsPLDβ2-KD plants. ()). These results suggested that HT-triggered ROS generation was involved in chalky grains production. Recently, Suriyasak et al [Citation13]. also reported the increased accumulation of ROS in the rice developing grains in response to HT. To examine the possibility that PLD enhanced ROS scavenging activity, we analyzed the catalase- and peroxidase-like activity in the grains in OsPLDβ2-KD and vector-control plants. Although no difference in the enzyme activity was observed between the vector-control plants in normal and HT growth conditions ()), the enzyme activity in the grains of OsPLDβ2-KD plants was slightly but significantly increased compared with that in vector-control plants ()). These results suggested that catalase- and peroxidase-like activity was activated in developing grains of OsPLDβ2-KD plants in HT growth conditions, which resulted in lower accumulation of H2O2.
Figure 2. Accumulation of H2O2 (a) and ROS-scavenging activity (b) in developing grain grown in normal or HT conditions. Each plant was grown in a greenhouse in normal conditions (27°C/25°C) before flowering and then, it was grown under normal or high temperature conditions (33°C/29°C). Each test sample was harvested at 10 DAF. (a) H2O2 was analyzed in the grains using the luminol chemiluminescence method. Values represent averages of triplicate experiments of three individual plants, and the error bars indicate SD. (b) The catalase-like activity was analyzed using H2O2 as a substrate. The values represent the average of triplicate experiments of three individual plants, and the error bars indicate SD. The symbol indicates a significant difference from the control plants at the 0.2% level.
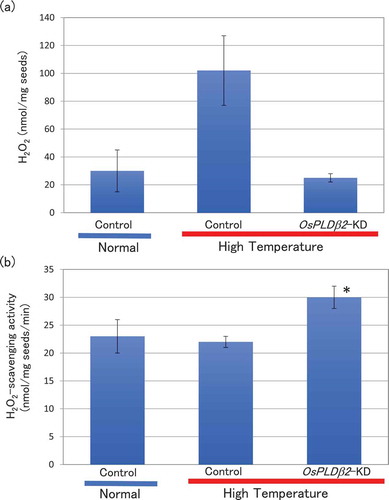
Up-regulation of a catalase gene expression by HT conditions in the OsPLDβ2-KD plants
To examine whether the up-regulation of higher ROS-scavenging activity by HT in OsPLDβ2-KD plants ()) was caused by increased expression of genes associated with ROS-scavenging, we performed expression analysis of rice catalase genes. The rice genome carries three catalase genes, OsCATA (Os02g0115700), OsCATB (Os06g0727200), and OsCATC (Os03g0131200) [Citation41,Citation42], whose accession numbers are shown in Table S1 and S2. Of these isoforms, the expression of OsCATA was significantly up-regulated in the grains of OsPLDβ2-KD plants compared with vector-control plants matured in HT conditions at 10 DAF (). These results suggested that OsPLDβ2 functions as a negative regulator of the induction of OsCATA and involved in the lower accumulation of ROS in grains in HT growth conditions.
Figure 3. Accumulation of OsCATA, OsCATB, or OsCATC mRNA in the grains of the vector-control or OsPLDβ2-KD plants in HT growth conditions. Each plant was grown in a greenhouse, and after flowering they were grown in HT conditions. Total RNA was extracted from the grains at 10 DAF. The values were standardized using the expression levels of a rice polyubiqutin gene (RUBIQ1). Values represent the average of triplicate experiments of three individual plants, and the error bars indicate SD. The symbol indicates a significant difference from the control at the 0.05% level.
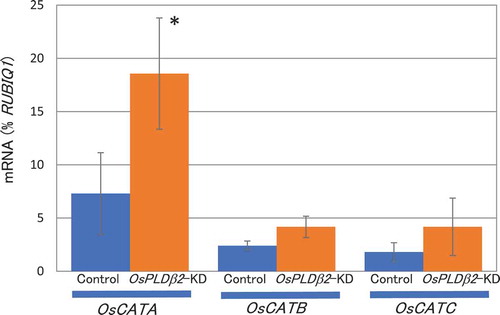
Overexpression of a catalase gene reduced chalky grains in HT conditions
To examine the function of catalase in chalky grain production, we generated the catalase gene-overexpression plants. As shown in ), the production of chalky grains of OsCATA-overexpression plants was significantly decreased compared with wild type plants under HT growth conditions. That of OsCATB-overexpression plants was only slightly decreased and no difference in the production of that was observed between OsCATC-overexpression and vector-control plants. More than 10 overexpression lines were established for each gene, and at least five independent lines were used for the analysis. Quantitative analysis of OsCATA, OsCATB, and OsCATC mRNA in the grains confirmed a clear increase of each gene expression ()). Five independent overexpression lines gave similar results (Fig. S1). These results indicated that the expression levels of OsCATA would be involved in the production and improvement of chalky grains by HT.
Figure 4. Changes in chalky grain production of the catalase gene overexpression plants in HT growth conditions. (a) Each plant was grown in a greenhouse in normal conditions (27°C/25°C) before flowering, and then it was grown in HT conditions (33°C/29°C). Values represent the ratio of chalky grains and the average of triplicate experiments, and the error bars indicate SD. The symbol indicates a significant difference from the control plants (HT) at the 0.01% level. (b) Appearance of grains. (c) Accumulation of OsCATA, OsCATB, or OsCATC mRNA in the grains of the OsCATA-ox, OsCATB-ox, OsCATC-ox, or vector-control plants in HT growth conditions. The homozygote ox and vector-control plants were grown in a greenhouse and after flowering they were grown in HT conditions. Total RNA was extracted from the grains at 10 DAF. Values were standardized to the expression levels of a rice polyubiqutin gene (RUBIQ1). Values represent the average of three individual plants, and the error bars indicate SD.
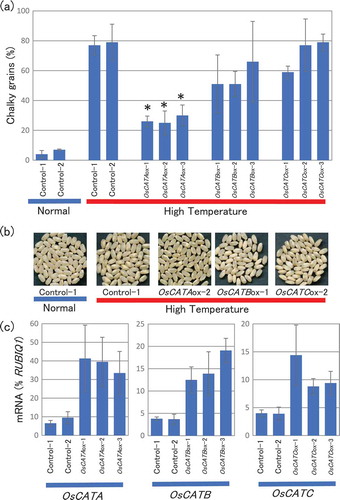
Discussion
In this study, we show that the knockdown of rice PLD genes, OsPLDα1, OsPLDα3, and OsPLDβ2, reduced chalky grain production in HT conditions (). According to the RiceXPro database (http://ricexpro.dna.affrc.go.jp/), which compiles expression data for entire rice genes covering all organs of rice plants in various developmental stages determined by extensive microarray analyses [Citation43], OsPLDα1 is highly expressed in most tissues. Although the expression of OsPLDα3 was lower compared with that of OsPLDα1, it was detected in most tissues. In addition, OsPLDβ2 was markedly highly expressed in specific tissues such as the ovary during the early period of development at 0 and 5 DAF. For these reasons, each PLD may be affected in chalky grain production in HT. In the previous study, PLD is well known to be involved in low temperature or freezing stress tolerance [Citation19,Citation23,Citation44,Citation45]. For heat stress responses in plants, an increase in PLD activity and accumulation of PA, which is a reaction product of PLD, were observed when tobacco BY-2 cells were incubated in HT (40°C) conditions [Citation46]. Previously, the accumulation of phospholipids and an increase in lipase activity had been reported in developing seeds in rice [Citation33]. For these reasons, we proposed here important roles of PLD in HT-stress responses.
To obtain insights into the underlying mechanism by which the knockdown of PLDs prevented chalky grain production in HT conditions, we analyzed the accumulation and scavenging activities of ROS using OsPLDβ2-KD plants. The results shown in suggested that the lower accumulation of ROS in the grains of OsPLDβ2-KD plants is caused by the up-regulation of the scavenging activity for ROS, indicating that the knockdown of OsPLDβ2 probably affected ROS scavenging activity. Concerning to the involvement of PLD in ROS generation, it has been suggested that PLD functions as both a positive and negative regulator of ROS generation [Citation15,Citation21,Citation39], indicating that PLD isoforms have distinct functions in ROS generation. As shown in , the knockdown of OsPLDβ2 induced the expression of OsCATA, which is one of three rice catalase genes, in the developing grains in HT conditions. Furthermore, OsCATA overexpression significantly decreased the production of chalky grains in HT conditions (). These results suggested that the reduction in chalky grain production in OsPLDβ2-KD plants might be caused by the enhanced response of OsCATA expression at HT. Thus, OsPLDβ2 functions as a positive regulator of ROS generation and chalky grain production in HT conditions. However, the potential mechanism of the regulation of OsCATA expression by OsPLDβ2 remains to be determined. The regulatory mechanism of OsCATA expression remains unclear although it is assumed to be involved in tolerance against environmental stress [Citation42]. It has been suggested that OsCATA expression shows the photoperiod-dependent manner and is not induced in response to ABA, drought, high salinity, and H2O2 treatments in leaves [Citation42]. According to the RiceXPro database, both OsCATA and OsPLDβ2 are highly expressed in the ovary after heading stage. The suppression of OsPLDβ2 might contribute to the up-regulation of OsCATA expression in the ovary in HT.
The results obtained in this paper indicated that the ROS H2O2 is a positive factor triggering chalky grain production at HT. Indeed, a similar observation was reported where the overexpression of a rice SOD gene, MSD1, could also improve chalky grains production [Citation12]. Shiraya and colleagues proposed that H2O2 formed by MSD1 induces the expression of ROS scavengers, molecular chaperones, and a quality control system in developing grains and suppresses chalky grain production at HT [Citation12]. However, the underlying mechanism of how H2O2 leads to the suppression of chalky grains production remains to be clarified.
H2O2 is the most stable ROS and an important signaling molecule involved in many plant cellular processes and environmental responses, including the heat stress response [Citation47]. However, it is not clear how the production of H2O2 is involved in chalky grain production by HT. Thus far, potential ROS receptors have been proposed, such as protein kinases, protein phosphatases, transcription factors, and ion channels [Citation47]. Recently, it has become clear that ROS including the hydroxyl radical, mainly formed from H2O2, are sensed by Ca2+ channels, which directly induce physiological responses such as cytosolic Ca2+ elevation [Citation47]. In addition, it has been reported that RNAi-mediated suppression of α-amylase genes resulted in an improvement in chalky grain production in HT growth conditions [Citation11]. Furthermore, in germinating cereal seeds, the elevation of α-amylase activities and its secretion are known to be Ca2+-dependent [Citation48,Citation49]. For these reasons, the up-regulation of cytosolic Ca2+ induced by ROS might contribute to the activation of α-amylase and chalky grain production in response to HT. To elucidate the mechanism, we should perform the analysis of α-amylase activity in developing grains of OsPLDα1, OsPLDα3, and OsPLDβ2-KD plants in HT growth conditions. Further analysis of the mechanism of chalky grain production by ROS should be the subject of future studies.
The results showed that the knockdown of rice PLD genes, OsPLDα1, OsPLDα3, and OsPLDβ2, reduced chalky grain production in HT conditions. This suggested that the suppression or deficiency of PLD genes is a potential strategy for ameliorating chalky grain production in HT conditions. Once mutants deficient in PLD genes are found to improve grain quality, they can be used as genetic resources for breeding HT-tolerant rice plants. In addition, PLD genes-KO plants might be able to produce by using genome editing technology, such as TALEN or CRISPR/Cas9. We propose here a potential strategy to produce a rice plants that is tolerant of HT from global warming.
Author Contribution
TY designed research, performed the experiments, and wrote the paper. HY, MN, MK, and MH assisted DNA constructs, rice transformation, and real-time PCR experiments.
Supplemental_data_Final.pdf
Download PDF (156.1 KB)Acknowledgments
We thank Ms H. Ikeda for technical assistance.
Disclosure statement
No potential conflict of interest was reported by the authors.
Supplementary material
Supplemental data for this article can be accessed here.
Additional information
Funding
References
- Morita S, Shiratsuchi H, Takahanashi J, et al. Effect of high temperature on grain ripening in rice plants. Analysis of the effects of high night and high day temperatures applied to the panicle and other parts of the plant. Jpn J Crop Sci. 2004;73:77–83.
- Yamakawa H, Hirose T, Kuroda M, et al. Comprehensive expression profiling of rice grain filling-related genes under high temperature using DNA microarray. Plant Physiol. 2007;144:258–277.
- Ishimaru T, Horigane AK, Ida M, et al. Formation of grain chalkiness and changes in water distribu-tion in developing rice caryopses grown under high-temperature stress. J Cereal Sci. 2009;50:166–174.
- Umemoto T, Terashima K. Activity of granule-bound synthase is an important determinant of amylose content in rice endosperm. Funct Plant Biol. 2002;29:1121–1124.
- Jiang H, Dian W, Wu P. Effect of high temperature on fine structure of amylopectin in rice endosperm by reducing the activity of the atarch branching enzyme. Phytochemistry. 2003;63:53–59.
- Kubo A, Fujita N, Harada K, et al. The starch-debranching enzymes isoamylase and pullulanse are both involved in amylopectin biosynthesis in rice endosperm. Plant Physiol. 1999;121:399–409.
- Nishi A, Nakamura Y, Tanaka N, et al. Biochemical and genetic analysis of the effect of amylase-extender mutation in rice endosperm. Plant Physiol. 2001;127:459–472.
- Tanaka N, Fujita N, Nishi A, et al. The structure of starch can be manipulated by changing the expression levels of starch branching enzyme IIb in rice endosperm. Plant Biotechnol J. 2004;2:507–616.
- Kang HG, Park S, Matsuoka M, et al. White-core endosperm floury endosperm-4 in rice is generated by knockout mutations in the C4-type pyruvate orthophosphate dikinase gene (OsPPDKB). Plant J. 2005;42:901–911.
- Fujita N, Satoh R, Hayashi A, et al. Starch biosynthesis in rice endosperm requires the presence of either starch synthase I or IIIa. J Exp Bot. 2011;62:4819–4831.
- Hakata M, Kuroda M, Miyashita T, et al. Suppression of α-amylase genes improves quality of rice grain ripened under high temperature. Plant Biotechnol J. 2012;10:1110–1117.
- Shiraya T, Mori T, Maruyama T, et al. Golgi/plastid-type manganese superoxide dismutase involved in heat-stress tolerance during grain filling of rice. Plant Biotechnol J. 2015;13:1251–1263.
- Suriyasak C, Harano K, Tanamachi K, et al. Reactive oxygene species induced by heat stress during grain filling of rice (Oryza sativa L.) are involved in occurrence of grain chakiness. J Plant Physiol. 2017;216:52–57.
- Wang X. Regulatory functions of phospholipase D and phosphatidic acid in plant growth, development, and stress responses. Plant Physiol. 2005;139:566–573.
- Yamaguchi T, Kuroda M, Yamakawa H, et al. Suppression of a phospholipase D gene, OsPLDβ1, activates defense responses and increases disease resistance in rice. Plant Physiol. 2009;150:308–319.
- Zhang W, Qin C, Zhao J, et al. Phospholipase Dα1-derived phosphatidic acid interacts with ABI1 phosphatase 2C and regulates abscisic acid signaling. Proc Natl Acad Sci USA. 2004;101:9508–9513.
- Fan L, Zheng S, Wang X. Antisense suppression of phospholipase D alpha retards abscisic acid- and ethylene-promoted senescence of postharvest arabidopsis leaves. Plant Cell. 1997;9:2183–2196.
- Sang Y, Zheng S, Li W, et al. Regulation of plant water loss by manipulating the expression of phospholipase Dα. Plant J. 2001;28:135–144.
- Welti R, Li W, Li M, et al. Profiling membrane lipids in plant stress responses: role of phospholipase D in freezing-induced lipid changes in Arabidopsis. J Biol Chem. 2002;277:31994–32002.
- Yu HQ, Yong TM, Li HJ, et al. Overexpression of a phospholipase D alpha gene from Ammopiptanthus nanus enhances salt tolerance of phospholipase D alpha 1-deficient Arabidopsis mutant. Planta. 2015;242:1495–1509.
- Zhang Y, Zhu H, Zhang Q, et al. Phospholipase D alpha 1 and phosphatidic acid regulate NADPH oxidase activity and production of reactive oxygen species in ABA-mediated stomatal closure in Arabidopsis. Plant Cell. 2009;21:2357–2377.
- Katagiri T, Takahashi S, Shinozaki K. Involvement of a novel Arabidopsis phospholipase D, AtPLDδ, in dehydration-inducible accumulation of phosphatidic acid in stress signaling. Plant J. 2001;26:595–605.
- Li W, Li M, Zhang W, et al. The plasma membrane-bound phospholipase D delta enhances freezing tolerance in Arabidopsis thaliana. Nature Biotech. 2004;22:427–433.
- Zhang W, Wang C, Qin C, et al. The oleate-stimulated phospholipase D, PLD, and phosphatidic acid decrease H2O2-induced cell death in Arabidopsis. Plant Cell. 2003;15:2285–2295.
- Guo L, Devaiah SP, Narasimhan R, et al. Cytosolic glyceraldehyde-3-phosphate dehydrogenases interact with phospholipase D delta to transduce hydrogen peroxide signals in the Arabidopsis response to stress. Plant Cell. 2012;24:2200–2212.
- Jia YX, Li WQ. Characterisation of lipid changes in ethylene-promoted senescence and its retardation by suppression of phospholipase D delta in Arabidopsis leaves. Front Plant Sci. 2015;6:1045.
- Distefano AM, Scuffi D, Garcia-Mata C, et al. Phospholipase D delta is involved in nitric oxide-induced stomatal closure. Planta. 2012;236:1899–1907.
- Dhonukshe P, Laxult AM, Goedhart J, et al. Phospholipase D activation correlates with microtubule reorganization in living plant cells. Plant Cell. 2003;15:2666–2679.
- Gardiner J, Collings DA, Harper JDI, et al. The effects of the phospholipase D-antagonist 1-butanol on seedling development and microtubule organization in Arabidopsis. Plant Cell Physiol. 2003;44:687–696.
- Shen P, Wang R, Jing W, et al. Rice phospholipase D alpha is involved in salt tolerance by the mediation of H(+)-ATPase activity and transcription. J Integr Plant Biol. 2011;53:289–299.
- Qi J, Zhou G, Yang L, et al. The chloroplast-localized phospholipases D α4 and α5 regulate herbivore-induced direct and indirect defenses in rice. Plant Physiol. 2011;157:1987–1999.
- Li G, Lin F, Xue HW. Genome-wide analysis of the phospholipase D family in Oryza sativa and functional characterization of PLDβ1 in seed germination. Cell Res. 2007;17:881–894.
- Choudhury N, Juliano B. Lipds in developing and mature rice grain. Phytochemistry. 1981;19:1063–1069.
- Kuroda M, Kimizu M, Mikami C. A simple set of plasmids for the production of transgenic plants. Biosci Biotechnol Biochem. 2010;74:2348–2351.
- Hood E, Helmer R, Fraley R, et al. The hypervirulence of Agrobacterium tumefaciens A281 is encoded in a region pTiBo542 outside of T-DNA. J Bacterol. 1986;168:1291–1301.
- Toki S. Rapid and efficient Agrobacterium-mediated transformation in rice. Plant Mol Biol Rep. 1997;15:16–21.
- Wang J, Jiang J, Oard JH. Structure, expression and promoter activity of two polyubiquitin genes from rice (Oryza sativa L.). Plant Sci. 2000;156:201–211.
- Kuchitsu K, Kosaka T, Shiga T, et al. EPR evidence for generation of hydroxyl radical triggered by N-acetylchitooligosaccharide elicitor and a protein phosphatase inhibitor in suspension-cultured rice cells. Protoplasma. 1995;188:138–142.
- Sang Y, Cui D, Wang X. Phospholipase D and phosphatidic acid-mediated generation of superoxide in Arabidopsis. Plant Physiol. 2001;126:1449–1458.
- Yamaguchi T, Ueki J, Minami E, et al. Elicitor-induced activation of phospholipases plays an important role for the induction of defense responses in suspension-cultured rice cells. Plant Cell Physiol. 2005;46:579–587.
- Mhamdi A, Queval G, Chaouch S, et al. Catalase function in plants: a focus on Arabidopsis mutants as stress-mimic models. J Exp Bot. 2010;61:4197–4220.
- Joo J, Lee JY, Song SI. Rice CatA, CatB, and CatC are involved in environmental stress response, root growth, and photorespiration, respectively. J Plant Biol. 2014;57:375–382.
- Sato Y, Antonio BA, Namiki N, et al. RiceXPro: a platform for monitoring gene expression in Japonica rice grown under natural field conditions. Nucleic Acids Res. 2011;39:D1141–D1148.
- Rajashekar CB, Zhou HE, Zhang Y, et al. Suppression of phospholipase D alpha 1 induces freezing tolerance in Arabidopsis: response of cold responsive genes and osmolyte accumulation. Plant Physiol. 2006;163:916–926.
- Huo C, Zhang B, Wang H, et al. Comparative study of early cold-regulated proteins by two-dimentional differemce gel electrophoresis reveals a key role for phospholipase Dα1 in mediating cold acclimation signaling oathway in rice. Mol Cell Proteomics. 2016;15:1397–1411.
- Mishkind M, Vermeer JEM, Darwish E, et al. Heat stress activates phospholipase D and triggers PIP2 accumulation at the plasma membrane and nucleus. Plant J. 2009;60:10–21.
- Demidchik V. Mechanisms of oxidative stress in plants: from classical chemistry to cell biology. Environ Exp Bot. 2015;109:212–228.
- Bush DS, Sticher L, Huystee R, et al. The calcium requirement for stability and enzymatic activity of two isoforms of barley aleurone α-amylase. J Biol Chem. 1989;264:19392–19398.
- Mitsui H, Ueki Y, Igaue I. Biosynthesis and secretion of α-amylase by rice suspension-cultured cells: purification and characterization of α-amylase isozyme H. Plant Physiol Biochem. 1993;31:863–874.