ABSTRACT
Difructose anhydride III (DFAIII) is a prebiotic involved in the reduction of secondary bile acids (BAs). We investigated whether DFAIII modulates BA metabolism, including enterohepatic circulation, in the rats fed with a diet supplemented with cholic acid (CA), one of the 12α-hydroxylated BAs. After acclimation, the rats were fed with a control diet or a diet supplemented with DFAIII. After 2 weeks, each group was further divided into two groups and was fed diet with or without CA supplementation at 0.5 g/kg diet. BA levels were analyzed in aortic and portal plasma, liver, intestinal content, and feces. As a result, DFAIII ingestion reduced the fecal deoxycholic acid level via the partial suppression of deconjugation and 7α-dehydroxylation of BAs following CA supplementation. These results suggest that DFAIII suppresses production of deoxycholic acid in conditions of high concentrations of 12α-hydroxylated BAs in enterohepatic circulation, such as obesity or excess energy intake.
Abbreviation: BA: bile acid; BSH: bile salt hydrolase; CA: cholic acid; DCA: deoxycholic acid; DFAIII: difructose anhydride III; MCA: muricholic acid; MS: mass spectrometry; NCDs: non-communicable diseases; LC: liquid chromatography; SCFA: short-chain fatty acid; TCA: taurocholic acid; TCDCA: taurochenodeoxycholic acid; TDCA: taurodeoxycholic acid; TUDCA: tauroursodeoxychlic acid; TαMCA: tauro-α-muricholic acid; TβMCA: tauro-β-muricholic acid; TωMCA: tauro-ω-muricholic acid
Graphical Abstract
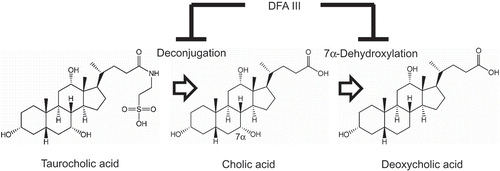
Suppressed deconjugation and 7α-dehydroxylation of 12α-hydroxylated bile acids by difructose anhydride III contribute to reduction of deoxycholic acid.
According to the World Health Organization, non-communicable diseases (NCDs) are responsible for almost 70% of deaths reported worldwide [Citation1]. The number of deaths is expected to increase worldwide from 38 million in 2012 to 52 million by 2030 [Citation2]. Unhealthy diets, including those with high portion of sugars or saturated fats (30%), drinking alcohol (30%), physical inactivity (10%), and smoking (10%) are the primary factors that raise the risk of NCDs. Dietary intervention is proposed as one of the suitable strategies to prevent NCDs [Citation3]. Epidemiologic studies have revealed that the consumption of an energy-dense diet increases obesity rate as well as the risk of diseases, including insulin resistance and metabolic syndrome [Citation4,Citation5].
One of prominent changes induced by energy-dense diet is the alteration in bile acid (BA) metabolism. BA is a steroid synthesized from cholesterol in the liver and contributes to lipid absorption [Citation6]. Primary BAs are conjugated with taurine or glycine in the liver and stored in the gallbladder. The conjugated BAs are secreted in response to diet consumption into the intestine through the bile duct to assist lipid absorption. The secreted BAs are reabsorbed through the portal vein into the liver and reused in the process called as enterohepatic circulation [Citation7]. In the large intestine, some gut microbes produce bile salt hydrolase (BSH), which deconjugates BA and liberates a taurine or glycine moiety [Citation8]. The production of BSH depends on the species of bacteria, intestinal environment, and BA concentration [Citation9]. The “free BA” becomes weakly acidic and undergoes 7α-dehydroxylation to produce secondary BAs [Citation10].
A significant increase in 12α-hydroxylated BAs, especially taurocholic acid (TCA) in the bile and deoxycholic acid (DCA) in the large intestine, is observed in the rats fed with a high-fat diet in our previous study [Citation11]. This observation suggests that the increased TCA flows into the gastrointestinal tract and undergoes deconjugation and 7α-dehydroxylation, resulting in an increase in the excretion of DCA in feces. Such an alteration in BA metabolism was observed in the rats fed with CA and was accompanied with an alteration in the gut microbiota composition [Citation12]. In the intestine, a variety of gut microbes are involved in BA metabolism [Citation13]. Ingestion of a prebiotic may modulate the gut microbiota and possibly alter BA metabolism and host health because some of the secondary BAs are risk factors associated with colon [Citation14] and liver cancer [Citation15].
Difructose anhydride III (di-D-fructofuranose-1,2′:2,3′-dianhydride, DFAIII), a non-digestible disaccharide, is mainly derived from inulin [Citation16]. DFAIII is high fermentable and enhances short-chain fatty acid (SCFA) synthesis during microbial fermentation in the cecum [Citation17]. Intestinal acidification after DFAIII supplementation [Citation18,Citation19] increases DFAIII-assimilating bacteria and decreases fecal secondary BA concentration [Citation20]. However, no information is available on BA metabolism, including enterohepatic circulation. In this study, we investigated whether DFAIII ingestion affects BA metabolism in combination with CA supplementation.
Material & methods
Animal and diets
The animal experiment was approved by the Institutional Animal Care and Use Committee of National University Corporation of Hokkaido University (14–0026). All animals were maintained according to the Hokkaido University Manual for Implementing Animal Experimentation. Male Wistar rats (n = 32; 3-week old; Japan SLC Inc, Hamamatsu, Japan) were individually housed in stainless steel cages in a controlled environment of temperature (22 ± 2°C), humidity (55 ± 5%), and light (from 8:00–20:00). Feeding schedule of the entire experimental period is presented in . Rats had free access to water and a control diet (C) based on AIN-93G [Citation21] () during the acclimation period for a week. The rats were then divided into two dietary groups, namely, C (n = 16) and D (DFAIII-supplemented diet) (n = 16). After feeding for 2 weeks, each group of rats was further divided into two groups and were fed diet with or without CA supplementation at 0.5 g/kg diet. Body weight and food intake were measured every 2 days. On day 33 of the test period, the rats were orally administered with a chromium (III) chloride hexahydrate (Wako Pure Chemical Industries, Ltd., Osaka, Japan) [Citation22] solution with ethylenediamine tetra acetate 2Na (Dojindo Laboratories, Kumamoto, Japan) for the determination of urinary chromium (Cr) excretion. Urine samples for 24 h were obtained after 24 h from oral administration for gut permeability test. Fecal samples were collected from day 34 to 35 and stored at −30°C for the evaluation of BAs. On day 35, the rats were sacrificed under anesthesia by an intraperitoneal injection of sodium pentobarbital (50 mg/kg body weight, Somnopentyl, Kyoritsuseiyaku Corporation, Tokyo, Japan). Blood plasma samples were collected using syringe from both abdominal aorta and portal vein with aprotinin (final concentration of 500 KIU/mL) and heparin (final concentration of 50 IU/mL). The blood samples were centrifuged and the supernatant collected. Cecum was collected and the weights of whole cecum and cecal contents were measured. The cecal contents were diluted four times with deionized water and homogenized with Teflon homogenizer. The pH of homogenates was measured using a semiconducting electrode (ISFET pH sensor Argus; Sentron, Roden, Netherlands). Cecal contents were kept in liquid nitrogen and stored at −80°C for BA analysis. Epididymal adipose tissue was dissected and weighed. Liver tissue weight was measured and the tissues were kept in liquid nitrogen and stored at −80°C for BA analysis.
Table 1. Diet compositions.
Figure 1. Feeding schedule.
After acclimation for 1 week, the rats were divided into two groups, including those fed with control diet (C) and DFAIII-supplemented diet (D) for 2 weeks. Rats from each group were further divided into two groups and fed the diet supplemented with CA (CB and DB) or without CA (C and D) (n = 8 each) for another 2 weeks.
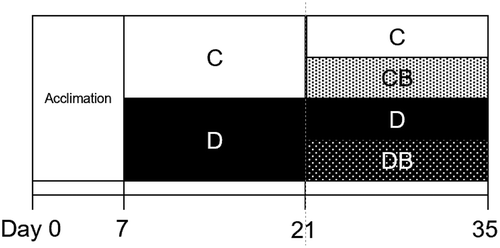
Gut permeability
The concentration of Cr in urine samples was measured using an atomic absorption spectrophotometer (Z-5310, Hitachi High-Tech., Tokyo, Japan). The proportion of urinary Cr excretion following oral administration of Cr-ethylenediaminetetraacetic acid solution was calculated as an indicator of gut permeability [Citation23].
BA analysis
BA extraction and liquid chromatography (LC) separation were performed with Dionex UltiMate 3000 UPLC system (Thermo Fisher Scientific corporation, San Jose, CA, USA) according to our previous study [Citation24]. Mass spectrometry (MS) was performed using Orbitrap mass spectrometer (Thermo Fisher Scientific) equipped with an electrospray ionization probe under negative-ion mode. Full-scan MS spectra (from m/z 200–700) were acquired with Orbitrap analyzer after accumulation to a target value of 1e6 in the linear ion trap. Resolution in Orbitrap system was set to r = 17,500. Standard mass spectrometric conditions for all experiments were as follows: spray voltage, 3.8 kV; sheath gas flow rate, 35 L/h; aux gas flow rate, 10 L/h; heated capillary temperature, 250°C; aux gas heater temperature, 450°C; s-lens RF level, 100%. Individual BA concentration was measured using nordeoxycholic acid (23-nor-5β-cholanic acid-3α,12α-diol) as an internal standard. BAs were measured as follows: 5β-cholanic acid-3α,7α,12α-triol (CA); 5β-cholanic acid-3α,6β,7α-triol; 5β-cholanic acid-3α,6β,7β-triol (β-muricholic acid, βMCA); 5β-cholanic acid-3α,6α,7β-triol (ω-muricholic acid, ωMCA); 5β-cholanic acid-3α,6α-diol; 5β-cholanic acid-3α,7β-diol; 5β-cholanic acid-3α,7α-diol; 5β-cholanic acid-3α,12α-diol (DCA); 5β-cholanic acid-3α-ol; 5β-cholanic acid-3α,6α,7α-triol; 5β-cholanic acid-3α,7β,12α-triol; 5β-cholanic acid-3α,12α-diol-7-one; 5β-cholanic acid-3α-ol-7-one (7-oxo-LCA); 5β-cholanic acid-3α-ol-12-one; 5β-cholanic acid-12α-ol-3-one (3o12α); 5β-cholanic-3α,7α,12α-triol-N-(2-sulphoethyl)-amide (TCA); 5β-cholanic-3α,6β,7α-triol-N-(2-sulphoethyl)-amide (tauro-α-muricholic acid, TαMCA); 5β-cholanic-3α,6β,7β-triol-N-(2-sulphoethyl)-amide (tauro-β-muricholic acid, TβMCA); 5β-cholanic-3α,6α,7β-triol-N-(2-sulphoethyl)-amide (tauro-ω-muricholic acid, TωMCA); 5β-cholanic-3α,6α-diol-N-(2-sulphoethyl)-amide; 5β-cholanic-3α,7β-diol-N-(2-sulphoethyl)-amide (tauroursodeoxycholic acid, TUDCA); 5β-cholanic-3α,7α-diol-N-(2-sulphoethyl)-amide (taurochenodeoxychlic acid, TCDCA); 5β-cholanic-3α,12α-diol-N-(2-sulphoethyl)-amide (taurodeoxycholic acid, TDCA); 5β-cholanic-3α-ol-N-(2-sulphoethyl)-amide; 5β-cholanic-3α,7α,12α-triol-N-(carboxymethyl)-amide; 5β-cholanic-3α,6α-diol-N-(carboxymethyl)-amide; 5β-cholanic-3α,7β-diol-N-(carboxymethyl)-amide; 5β-cholanic-3α,7α-diol-N-(carboxymethyl)-amide; 5β-cholanic-3α,12α-diol-N-(carboxymethyl)-amide; and 5β-cholanic-3α-ol-N-(carboxymethyl)-amide.
Fecal DFAIII excretion
Fecal DFAIII excretion in the DFAIII-fed rats was measured based on the method as previously described [Citation20]. Briefly, homogenized fecal samples were added to deionized water and sonicated. After centrifugation, the supernatant was collected and degreased with chloroform. The amount of DFAIII was analyzed using high-performance LC (Hitachi High-Tech Science Co., Tokyo) with a TSKgel Amide-80 column (4.6 × 250 mm).
Statistical analysis
All data are presented as the mean ± standard error of means (SEM). Two-way analysis of variance (ANOVA) test was used to represent significant interaction between two factors, BA and DFAIII. Student’s t-test was used for comparison of DFAIII excretion between two groups of rats fed diet with or without CA supplementation. All statistical analysis was performed using JMP software version 13.0 (SAS Institute Inc., Cary, NC, USA).
Results
Reduction of fecal DFAIII concentration
To investigate whether the ingested DFAIII was assimilated by the intestinal microbes, the fecal DFAIII excretion was determined as shown in . Fecal excretion of DFAIII was the highest on day 8 and gradually decreased thereafter. On day 21, the rats were divided into two groups and fed with or without CA-supplemented diet to evaluate the effect of CA supplementation on fecal DFAIII excretion. As a result, the fecal DFAIII was detected at trace levels under CA supplementation condition. A significant difference was observed between the groups on day 27, but only a trace level was detected.
Figure 2. Changes in fecal DFAIII excretion in the rats fed with DFAIII with or without CA supplementation.
The proportion of fecal DFAIII following DFAIII supplementation was calculated in D (open circle, n = 8) and DB (closed circle, n = 8) groups. Asterisk represents significant differences in the values between groups at the same time point (P < 0.05).
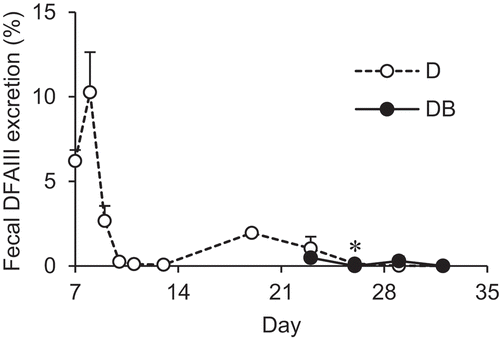
Alteration of BA metabolism in rats fed with the DFAIII diet
Thirty molecular species of BAs were analyzed using LC-MS at the end of the experimental period, as shown in . TCA was the most abundant BA in the liver, intestinal contents, and portal plasma. CA supplementation increased the concentration of TCA at these sites. Two-way ANOVA results revealed the synergetic effect of CA and DFAIII supplementation on TCA concentration in the portal plasma. A similar tendency was observed in the ileal contents. CA supplementation increased the concentration of TDCA in the liver; a significant interaction was found between CA and DFAIII. Similar changes were observed in the cecal and fecal DCA concentrations, as shown in the liver TDCA. A synergetic effect of CA and DFAIII supplementation was observed on both CA and TCA concentrations in the cecal contents and feces. The trend in fecal 3o12α excretion resembled that observed for DCA. On the other hand, the concentration of ωMCA decreased after CA and DFAIII supplementation. Similar tendency was also observed in the feces.
Figure 3. Distribution of BAs in the rats fed with four different diets at the end of the experimental period.
BA concentrations were measured in the aorta plasma, portal plasma, liver, ileal contents, cecal contents, and feces of the rats fed with four different diets. Values are the mean ± SEM (n = 7 or 8 rats per group). Two-way ANOVA was used to determine significant differences (P < 0.05).
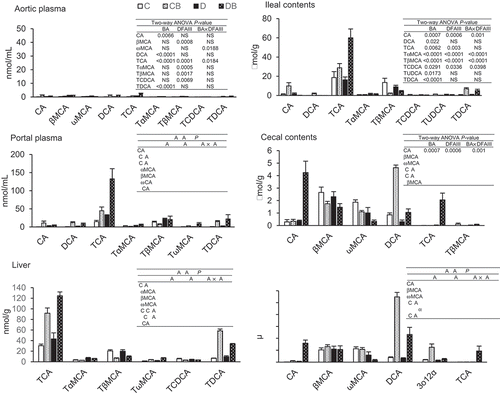
Biochemical parameters in the rats fed the DFAIII diet
Growth and biochemical parameters are shown in . DFAIII-supplemented diets decreased the total food intake, final body weight, and epididymal adipose tissue weight. The weights of whole cecum and its contents were higher in the rats fed with DFAIII-supplemented diets than in those from the other groups and a reduction in the pH of the cecal content was observed. The pH reduction was significantly affected by CA supplementation. CA supplementation enhanced gut permeability, as observed with urinary Cr excretion, but no difference was reported in the rats fed with DFAIII-supplemented diet.
Table 2. Growth and other parameters in the rats.
Discussion
Gas chromatography/MS mainly used for the determination of BA profiles necessitates the derivatization step. This derivatization process breaks taurine or glycine conjugation of BAs and poses difficulty in the discrimination between conjugated and unconjugated BAs [Citation25]. In the present study, we analyzed not only unconjugated BAs but also the conjugated forms of BAs using LC/MS [Citation24] that enabled us to elucidate the effect of DFAIII ingestion on BA metabolism, especially in the organs related with enterohepatic circulation.
In the rats fed control diet, the proportion of 12α-hydroxylated BAs was almost comparable that of non-12α-hydroxylated BAs in liver, ileal contents, and portal plasma (). On the other hand, an increase in the proportion of non-12α-hydroxylated BAs was observed in large intestine and in feces such as βMCA and ωMCA. Those results suggest that 12α-hydroxylated BAs are selectively reabsorbed in ileal epithelial cells. This notion is supported by observations in mice [Citation26] that show enormous excretion of ωMCA in feces rather than 12α-hydroxylated BAs. As expected, the CA-supplemented diet raised the concentration of 12α-hydroxylated BAs, for example TCA in enterohepatic circulation and DCA in cecal contents (). The DFAIII-supplemented diet reduced cecal and fecal ωMCA concentrations. βMCA is converted into ωMCA in the large intestine by anaerobic bacteria, such as Eubacterium lentum strain [Citation27], suggesting that the ingestion of DFAIII reduces conversion of βMCA into ωMCA by the gut microbes. Interestingly, in combination of CA with DFAIII, massive increase of TCA concentration was observed in the organs related with enterohepatic circulation accompanied by decreased fecal DCA excretion. These results suggest that 12α-hydroxylated BAs were selectively reused in enterohepatic circulation.
Simple feeding with CA-supplemented diet resulted in an increase in BA concentration mainly in the organs associated with enterohepatic circulation. CA supplementation increased DCA concentration in the large intestine and feces, indicating that the ingested CA was completely dehydroxylated into DCA by the gut microbes. On the other hand, the elevated DCA concentration was normalized in the rats fed with DFAIII that resulted in the reduction in the pH of the cecal contents. Minamida and colleagues [Citation25] measured the conversion of CA to DCA using thin-layer chromatography and found that DCA production was significantly suppressed at pH 5.8 as compared to that observed at pH 7.5, suggestive of the connection between low pH and reduction in 7α-dehydroxylation under DFAIII-fed condition. The decrease in pH of the large intestine is thought to inhibit 7α-dehydroxylase activities in the luminal contents, contributing to the reduction in DCA concentration. In addition, DFAIII ingestion increased acetate and other organic acid levels in the cecal contents that may serve as a contributing factor for the reduced pH of the cecal contents [Citation20]. A significant increase in the population of Ruminococcus productus was observed in the cecal contents of the rats fed with DFAIII that negatively correlated with the reduction in DFAIII excretion in the feces [Citation20]. On the other hand, R. productus was undetected in control rats. These results suggest that DFAIII is consumed by, and contributes to the growth of, R. productus. R. productus was shown to assimilate DFAIII under in vitro conditions. Almost all BAs of the feces in control rats were secondary BAs but those in DFAIII-fed rats were primary BAs [Citation20], suggesting that the altered gut microbiota by DFAIII ingestion had less ability to perform 7α-dehydroxylation.
Deconjugation of BA is catalyzed by BSHs in the intestinal bacteria that hydrolyze the amino bond and liberate taurine or glycine moiety from the steroid ring of BA [Citation8]. In general, an enormous decrease in conjugated BA levels was observed in the large intestine after deconjugation; these deconjugated BAs were converted into secondary BAs in the subsequent dehydroxylation step [Citation24]. High concentration of TCA was observed in the large intestine of the rats fed with CA and DFAIII, wherein BAs underwent 7α-dehydroxylation by the gut microbiota. The suppression of BA deconjugation reaction by DFAIII ingestion was responsible for the subsequent reduction in secondary BA production.
A decrease in body weight was observed in the rats fed with DFAIII supplementation as compared to those fed with DFAIII-free diets. As mentioned before, an increase in acetic acid level was observed in the gut that served as a potent stimulator to secrete anorexic gut hormones such as glucagon-like peptide-1 and peptide YY [Citation28]. It was recently revealed that acetic acid reduces appetite via direct influence on hypothalamus in the brain without induction of these gut hormones [Citation29]. In both cases, acetic acid is one of the candidates that suppressed appetite in the rats fed with DFAIII, resulting in the reduction in body weight. Furthermore, we observed a different influence of CA supplementation on BA concentrations in portal and aorta blood. CA supplementation increased portal BAs but not aorta BAs. The absorbed BAs in the ileal epithelia entered the enterohepatic circulation, as reflected in the BA profiles of the portal blood. The supplemented CA also enters this route rather than the systemic blood flow (aortic blood). This observation suggests that the effect of CA supplementation on the host may be restricted in the organs related to enterohepatic circulation. We observed that CA supplementation increased the gut permeability, but DFAIII intervention had no significant influence on permeability (). DCA is reported to disrupt the gut barrier in the large intestine [Citation30] and cultured epithelial cells [Citation31]. Regardless of the significant reduction in DCA concentration observed in the cecal contents and feces following DFAIII supplementation in the present study, no influence was observed on gut permeability following the CA supplementation (). In the rats fed with CA-supplemented diet, an increase in DCA or TCA was found in cecal contents or ileal contents, respectively (). TCA might induce leaky gut in small intestine, not in the large intestine.
In our previous study, we found that BA metabolism especially in the gut induced after CA supplementation (0.5 g/kg) resembles that observed in the rats fed with a high-fat diet, suggesting that the consumption of energy-dense diet may selectively increase DCA levels in the large intestine [Citation11]. Such increases in DCA levels in the stool were reported in the patient with colorectal cancer, suggestive of the link between DCA production in the large intestine and cancer development in the colon [Citation32]. Only limited data are available on conjugated BAs in feces. Under normal conditions, almost no conjugated BAs were observed in the stool of humans and feces in experimental animals [Citation33,Citation34]. In our previous study, we measured the levels of conjugated BAs in the feces of rats fed with soy pulp and Bacillus coagulans and found that conjugated BAs were absent in the feces [Citation35]. It may be rare to observe fecal-conjugated BAs following dietary intervention, as observed in the present study. Therefore, dietary DFAIII may be a prebiotic that reduces secondary BA concentration via suppression of 7α-dehydroxylation and deconjugation.
In conclusion, DFAIII altered BA metabolism, especially in the gut, through the suppression of deconjugation and 7α-dehydroxylation, which results in the reduction in DCA level in the large intestine probably through the modulation of gut microbiota. Hence, DFAIII may serve as a prebiotic source that reduces secondary BA production.
Author contribution
S.I., T.N., H.S., S.F, and A.Y. designed the experiments. DG.L., S.H., O.K., S.K., R.Y., Y.T., K.T., S.F. and S.I. performed experiments. All authors discussed the data. DG.L. and S.I. wrote the paper.
Disclosure statement
No potential conflict of interest was reported by the authors.
Additional information
Funding
References
- Riley L, Cowan M. Noncommunicable diseases country profiles. Geneva (Switzerland): World Health Organization; 2018.
- Renzella J, Townsend N, Jewell J, et al. Health evidence network synthesis report. 58. Copenhagen (Denmark): World Health Organization; 2018.
- Alwan A. Global status report on noncommunicable diseases 2010. Geneva (Switzerland): World Health Organization; 2011.
- Mendoza JA, Drewnowski A, Christakis DA. Dietary energy density is associated with obesity and the metabolic syndrome in U.S. adults. Diabetes Care. 2007;30:947–979.
- Yao M, Roberts SB. Dietary energy density and weight regulation. Nutr Rev. 2001;59:247–258.
- Uchida K, Nomura Y, Takeuchi N. Effect of cholic acid, chenodeoxycholic acid, and their related bile acids on cholesterol, phospholipid, and bile acid levels in serum, liver, bile, and feces of rats. J Biochem. 1980;87:187–194.
- Chiang JYL, Ferrell JM. Bile acid metabolism in liver pathobiology. Gene Expr. 2018;18:71–87.
- Begley M, Hill C, Gahan CGM. Bile salt hydrolase activity in probiotics. Appl Environ Microbiol. 2006;72:1729–1738.
- Ahn YT, Kim GB, Lim KS, et al. Deconjugation of bile salts by Lactobacillus acidophilus isolates. Int Dairy J. 2003;13:303–311.
- Liong MT, Shah NP. Bile salt deconjugation ability, bile salt hydrolase activity and cholesterol co-precipitation ability of lactobacilli strains. Int Dairy J. 2005;15:391–398.
- Yoshitsugu R, Kikuchi K, Iwaya H, et al. Alteration of bile acid metabolism by a high-fat diet is associated with plasma transaminase activities and glucose intolerance in rats. J Nutr Sci Vitaminol. In press.
- Islam KBMS, Fukiya S, Hagio M, et al. Bile acid is a host factor that regulates the composition of the cecal microbiota in rats. Gastroenterology. 2011;141:1773–1781.
- Kakiyama G, Pandak WM, Gillevet PM, et al. Modulation of the fecal bile acid profile by gut microbiota in cirrhosis. J Hepatol. 2013;58:949–955.
- Cao H, Xu M, Dong W, et al. Secondary bile acid-induced dysbiosis promotes intestinal carcinogenesis. Int J Cancer. 2017;140:2545–2556.
- Yoshimoto S, Loo TM, Atarashi K, et al. Obesity-induced gut microbial metabolite promotes liver cancer through senescence secretome. Nature. 2013;499:97–101.
- Hang H, Mu W, Jiang B, et al. Recent advances on biological difructose anhydride III production using inulase II from inulin. Appl Microbiol Biotechnol. 2011;92:457–465.
- Zhu Y, Yu S, Zhang W, et al. Recent advances on biological production of difructose dianhydride III. Appl Microbiol Biotechnol. 2018;102:3007–3015.
- Tamura A, Nino H, Minobe T, et al. Difructose anhydride III does not contribute to body energy accumulation in rats. Biosci Biotechnol Biochem. 2006;70:1416–1422.
- Mineo H, Amano M, Minaminida K, et al. Two-week feeding of difructose anhydride III enhances calcium absorptive activity with epithelial cell proliferation in isolated rat cecal mucosa. Nutrition. 2006;22:312–320.
- Minamida K, Kaneko M, Ohashi M, et al. Effects of difructose anhydride III (DFA III) administration on bile acids and growth of DFA III-assimilating bacterium Ruminococcus productus on rat intestine. J Biosci Bioeng. 2005;99:548–554.
- Reeves PG, Nielsen FH, Fahey GC Jr. AIN-93 purified diets for laboratory rodents: final report of the American institute of nutrition ad hoc writing committee on the reformulation of the AIN-76A rodent diet. J Nutr. 1993;123:1939–1951.
- Downes AM, McDonald IW. The chromium-51 complex of ethylenediamine tetraacetic acid as a soluble rumen marker. Br J Nutr. 1964;18:153–162.
- Ten Bruggencate SJM, Bovee-Oudenhoven IMJ, Lettink-Wissink MLG, et al. Dietary fructooligosaccharides increase intestinal permeability. J Nutr. 2005;135:837–842.
- Hagio M, Matsumoto M, Fukushima M, et al. Improved analysis of bile acids in tissues and intestinal contents of rats using LC/ESI-MS. J Lipid Res. 2009;50:173–180.
- Minamida K, Asakawa C, Sujaya IN, et al. Effects of long-term ingestion of difructose anhydride III (DFA III) on intestinal bacteria and bile acid metabolism in humans. J Biosci Bioeng. 2006;101:149–156.
- Fu ZD, Klaassen CD. Increased bile acids in enterohepatic circulation by short-term calorie restriction in male mice. Toxicol Appl Pharmacol. 2013;273:680–690.
- Eyssen H, De Pauw G, Stragier J, et al. Cooperative formation of ω-muricholic acid by intestinal microorganisms. Appl Environ Microbiol. 1983;45:141–147.
- Hira T, Yanagihara K, Koga T, et al. Impact of difructose anhydride III, raffinose, and fructooligosaccharides on energy intake, gut hormones, and cecal fermentation in rats fed a high-fat and high-sucrose diet. Biosci Biotechnol Biochem. 2017;81:2184–2194.
- Frost G, Sleeth ML, Sahuri-Arisoylu M, et al. The short-chain fatty acid acetate reduces appetite via a central homeostatic mechanism. Nat Commun. 2014;5:3611.
- Münch A, Ström M, Söderholm JD. Dihydroxy bile acids increase mucosal permeability and bacterial uptake in human colon biopsies. Scand J Gastroenterol. 2007;42:1167–1174.
- Raimondi F, Santoro P, Barone MV, et al. Bile acids modulate tight junction structure and barrier function of Caco-2 monolayers via EGFR activation. Am J Physiol Gastrointest Liver Physiol. 2008;294:G906–G910.
- Reddy BS, Mangat S, Sheinfil A, et al. Effect of type and amount of dietary fat and 1,2-dimethylhydrazine on biliary bile acids, fecal bile acids, and neutral sterols in rats. Cancer Res. 1977;37:2123–2127.
- Korpela JT, Adlercreutz H, Turunen MJ. Fecal free and conjugated bile acids and neutral sterols in vegetarians, omnivores, and patients with colorectal cancer. Scand J Gastroenterol. 1988;23:277–283.
- Nagengast FM, Hectors MPC, Buys WAM, et al. Inhibition of secondary bile acid formation in the large intestine by lactulose in healthy subjects of two different age groups. Eur J Clin Invest. 1988;18:56–61.
- Lee Y, Yoshitsugu R, Kikuchi K, et al. Combination of soya pulp and Bacillus coagulans lilac-01 improves intestinal bile acid metabolism without impairing the effects of prebiotics in rats fed a cholic acid-supplemented diet. Br J Nutr. 2016;116:603–610.