ABSTRACT
Membrane-bound quinoprotein glucose dehydrogenase from acetic acid bacteria produces lactobionic acid by the oxidation of lactose. Its enzymatic activity on lactose and maltose is much lower than that on D-glucose. For that reason, the activity of the enzyme on disaccharides has been considered low. In this study, we show that the isomaltose-oxidizing activity of acetic acid bacteria is much higher than their lactose-oxidizing activity. In addition to isomaltose, the enzyme oxidized gentiobiose and melibiose to the same extent. According to the characteristics of the isomaltose-oxidizing activity and investigations using dehydrogenase-deficient mutant bacteria, we identified the responsible enzyme as membrane-bound quinoprotein glucose dehydrogenase.
Abbreviations: AAB: acetic acid bacteria; m-GDH: membrane-bound quinoprotein glucose dehydrogenase; DCIP: 2,6-dichlorophenolindophenol; DP: degree of polymerization; HPAEC-PAD: high-performance anion-exchange chromatography with pulsed amperometric detection; NMR: nuclear magnetic resonance; TLC: thin layer chromatography; COSY: correlation spectroscopy
Graphic Abstract
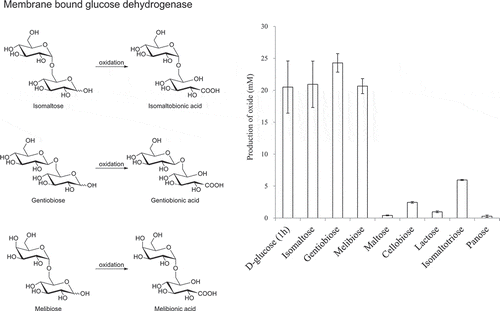
Membrane-bound quinoprotein glucose dehydrogenase (m-GDH) from acetic acid bacteria oxidizes disaccharides having α/β-1➝6 glycosidic linkages.
Conversion of the reducing end of the aldose residue of an oligosaccharide to aldonic acid produces oligoaldonic acid. Oligoaldonic acid has potential health benefits when used in foods. For example, lactobionic acid (β-d-galactopyranosyl-(1➝4)-d-gluconic acid) is an oligoaldonic acid that promotes the intestinal absorption of calcium [Citation1].
In our previous study, we investigated the production of lactobionic acid by acetic acid bacteria (AAB) [Citation2–Citation5], and found that membrane-bound quinoprotein glucose dehydrogenase (m-GDH) from AAB produced lactobionic acid from lactose [Citation6]. Lactobionic acid produced using AAB is now added in yogurt, beverages, and dietary supplements available in Japanese supermarkets [Citation5,Citation7]. However, the relative oxidizing activities of AAB toward lactose are not high (< 10% of those toward D-glucose) [Citation2,Citation5]. Thus, the purpose of this study was to find disaccharides that are effectively oxidized by AAB. We found that isomaltose and other disaccharides with α and β1,6 linkages are more effectively oxidized by AAB than lactose. In addition, we identified the oxidized products and the oxidizing enzyme responsible.
Materials and methods
Acetic acid bacteria
Acetobacter orientalis KYG22, which produces lactobionic acid in Caspian Sea yogurt, was isolated from this yogurt [Citation2]. Gluconobacter frateurii NBRC 3285 was selected as the highest isomaltose-oxidizing acetic acid bacterial strain. Gluconobacter oxydans IFO 3292, Gluconobacter cerinus IAM 14721, Gluconacetobacter hansenii NBRC 14816, and Komagataeibacter medellinensis NBRC 3288 were also selected as high isomaltose-oxidizing strains. In addition to KYG22, Acetobacter pasteurianus NBRC 3283 and Acetobacter aceti NBRC 3281 were selected as typical strains of Acetobacter spp. The genome and plasmid sequences of K. medellinensis NBRC 3288 are available for download from the public genome database of the Kyoto Encyclopedia of Genes and Genomes (KEGG) [Citation8]. The m-GDH-deficient mutant was prepared in our previous study via knockout of the m-GDH gene from its genome [Citation6]. Another twenty-two acetic acid bacterial strains were also used for screening of isomaltose-oxidizing acetic acid bacterial strains, as described below.
Analysis of oxidizing activity and production of saccharide oxide
The measurement of the sugar-oxidizing activities of cell suspensions or membrane fractions using the 2,6-dichlorophenolindophenol (DCIP) method was performed as described previously [Citation4]. The absorbance at 530 nm (ε530 = 7500 M−1 cm−1) was measured and used to calculate the consumption rate of DCIP. One unit of the cells and fractions was defined as the amount of cells and enzyme that consumed 1 μmol of DCIP per min. The production of saccharide oxide was measured by thin layer chromatography (TLC) [Citation2] and high-performance anion-exchange chromatography with pulsed amperometric detection (HPAEC-PAD) [Citation2]. The amounts of oligosaccharide oxides were calculated from the amounts of lactobionic acid.
Mass spectrometer analysis
The oligosaccharide oxides were diluted with 60% acetonitrile and the mass spectra were recorded on a Thermo Finnigan LCQ Deca spectrometer (San Diego, CA) as follows: ionization, electrospray ionization; ion spray voltage, −5 kV (negative ionization mode).
Nuclear magnetic resonance (NMR)
1H NMR and 13C NMR spectra were recorded on a JEOL AL-300 spectrometer (Tokyo, Japan) at 300 and 75 MHz, respectively. Two-dimensional spectra (H-H COSY and C-H COSY) were also recoded on the JEOL AL-300. The sample concentration was 1% in deuterium oxide. 3-(Trimethylsilyl)-1-propanesulfonic acid sodium salt was used as the internal standard.
Preparation of resting cells
AAB were precultured in YPGL medium (pH 7.0) [Citation2] at 27°C for 2 days with shaking at 240 oscillations/min, after which the cultures (1/100 volume) were transferred to new test tubes containing YPGL medium (pH 7.0) or SG medium (pH 7.0) [Citation6]. After cultivation for 3 days under the same conditions as the preculture, the cells were harvested from the broths, washed twice with saline, and then resuspended in saline (1/20 volume of the broth). This 20-fold-concentrated cell suspension was used in experiments as 20× resting cells. The activities of the cells were measured by the DCIP method described above and expressed as activities in 1 mL of 1× cell suspension (U/mL).
Screening of AAB that effectively oxidize isomaltose
The resting cells of the thirty acetic acid bacterial strains were cultivated in YPGL medium. The cell growth (absorbance at 660 nm, A660) and pH of the reaction mixture were measured. The resting cells (2×) of each strain were incubated in a reaction mixture consisting of 1% isomaltose and 100 mM acetate buffer (pH 5.5) at 27°C for 24 h with shaking (240 oscillation/min). After shaking, the reaction mixtures were analyzed using TLC (n = 1) or HPAEC-PAD (n = 3–5).
Substrate specificities of resting cells determined using the DCIP method
Strains NBRC 3288, NBRC 3285, IFO 3292, and IAM 14721 were cultivated in YPGL medium. The resting cells of NBRC 3288 (0.017–0.68 U/mL), NBRC 3285 (0.016–0.16 U/mL), IFO 3292 (0.036–0.36 U/mL), and IAM 14721 (0.060–0.60 U/mL) were examined. The reaction mixtures containing resting cells, 7.5 μM DCIP, 100 mM acetate buffer (pH 5.5), and 50 mM substrate were incubated at 40°C for 6–30 min; subsequently, the absorbance at 530 nm of the reaction mixtures was measured and the DCIP-consumption rate was used to calculate oxidizing activity.
Production of aldonic acid by resting NBRC 3285 cells and other AAB
AAB were cultivated in YPGL medium. The resting cells of NBRC 3285 (0.036 U/mL) were incubated with 27.8 mM substrate in 100 mM acetate buffer (pH 5.5) at 27°C for 1 h (D-glucose) or 24 h (other saccharides) with shaking (240 oscillations/min). The resting cells (2×) of the other AAB were incubated with 27.8 mM disaccharide or 18.5 mM trisaccharide (isomaltotriose or panose) in 100 mM acetate buffer (pH 5.5) at 27°C for 24 h or 72 h (panose) with shaking (240 oscillations/min). After the incubation, the reaction mixtures were boiled for 5 min and the production of aldonic acid was measured by HPAEC-PAD.
Effect of D-glucose on isomaltose oxidation by resting cells
G. frateurii NBRC 3285 was cultivated in YPGL medium. NBRC 3285 resting cells (0.070 U/mL) were mixed with 27.6 or 55.2 mM isomaltose, 0–111 mM d-glucose, and 500 mM acetate buffer (pH 5.5). The reaction mixtures were shaken (240 oscillations/min) at 27°C for 1 h. The production of oxide was then measured using HPAEC-PAD.
Purification and identification of oligoaldonic acid
Reaction mixtures (20 mL) containing 2.0 U/mL of the NBRC 3285 resting cells, 2.8 mg/mL CaCO3, and 1.0% substrate (isomaltose, melibiose, gentiobiose, or isomaltotriose), were incubated at 27°C for 3 days with shaking (240 oscillation/min). Conversions of the oligosaccharides were confirmed by TLC analysis. The purification method for isomaltobionic acid and melibionic acid is described below. After removal of the cells, the reaction mixture was loaded on an IRA-93ZU anion exchange (Orugano Co., Tokyo, Japan) column (3.0 × 15 cm) equilibrated with 100 mM NaCl. After washing with distilled water, the aldonic acid was eluted with a linear gradient of 0–0.5 M NaCl at 0.5 mL/min. The content of aldonic acid in each fraction was monitored by the phenol-sulfuric acid method [Citation9] and HPAEC-PAD. The product-containing fractions were collected and lyophilized. The products were resuspended in 0.5 mL of distilled water and loaded on a Bio-Gel P-2 (Bio-Rad Laboratories, Hercules, CA) column (2.5 × 90 cm) equilibrated with 5% ethanol. The aldonic acid was eluted with 5% ethanol at 10 mL/h. The eluates were collected, and the fractions corresponding to the oxidized products were combined, evaporated, and lyophilized.
The oxidized products were identified and compared with the disaccharide substrates reported using 1H, 13C, and mass spectrometry [Citation10]. Gentiobionic acid and isomaltotrionic acid were purified using TLC [Citation2] and their molecular masses were verified using mass spectrometry.
Oxidation of oligosaccharides by m-GDH-deficient resting cells
The m-GDH-deficient mutant was prepared by insertion of the chloramphenicol acetyltransferase gene into the m-GDH locus on the genome of K. medellinensis NBRC 3288 by homologous recombination, as described previously [Citation6]. Wild-type NBRC 3288 and the m-GDH-deficient mutant were cultivated in SG medium. The 20× resting-cell suspensions were appropriately diluted, 1-fold or 10-fold (for d-glucose), and mixtures containing the diluted suspensions of resting cells (1/40 volume), 100 mM acetate buffer (pH 5.5), and substrate (25 mM d-glucose or 100 mM for other disaccharides) were incubated at 27°C with shaking (240 oscillations/min). After incubating for 2 h (d-glucose), 7 h, or 24 h (lactose), aldonic acid production by the AAB was measured using HPAEC-PAD.
Assimilation of isomaltooligosaccharide and isomaltobionic acid
G. frateurii NBRC 3285 and K. medellinensis NBRC 3288 were precultured in YPGL medium (5 mL) for 2 days. The cultured bacteria (10 µL) were inoculated in media (1.0 mL), consisting of 0.5% dried yeast extract D-3 (Nihon Pharmaceutical Co. Ltd., Tokyo, Japan), 0.5% Polypepton (Nihon Pharmaceutical Co. Ltd.), 0.2% anhydrous MgSO4, and 1% each of D-glucose, lactose, calcium lactobionate, calcium isomaltobionate, and isomaltooligosaccharide (Wako Pure Chemical Industries, Ltd., Osaka, Japan). Distilled water was added to the media instead of the saccharides for the controls. The media was incubated at 27°C with shaking (240 oscillation/min). The pH of the media and the growth of the bacteria (A660) were measured after 3 days of culture.
Results
Comparison of oxidation of isomaltose and lactose by the resting cells of A. orientalis KYG22
In our previous studies, we reported that various AAB possess lactose-oxidizing activities. However, the activities on lactose were much lower than those on D-glucose [Citation2,Citation5]. Because the oxidizing activity of AAB on isomaltose was unknown, we compared the oxidation of lactose by the resting cells of A. orientalis KYG22 with that of isomaltose (). The amount of isomaltose oxide produced was much larger than that of lactobionic acid. The lactose-oxidizing enzyme is m-GDH, which is embedded in the cell membrane [Citation6]. Thus, we investigated the activities of the membrane fraction of A. orientalis KYG22 on disaccharides having α- and β-1,6-glycosidic linkages () by consumption of DCIP, as previously described [Citation4]. The relative activities on isomaltose, gentiobiose, and melibiose were also much higher than those on other disaccharides.
Figure 1. Oxidation of lactose and isomaltose by resting cells of A. orientalis KYG22.
The resting cells of KYG22 (2.7 U/mL) were incubated with 1% lactose or isomaltose in 1.4 mg/mL CaCO3 at 27°C with shaking for the indicated times (h). The reaction mixtures were analyzed using TLC. The spots of lactobionic acid and isomaltose oxide migrated slower than those of lactose and isomaltose, respectively. The pH of the cultures was 5.1–5.7.
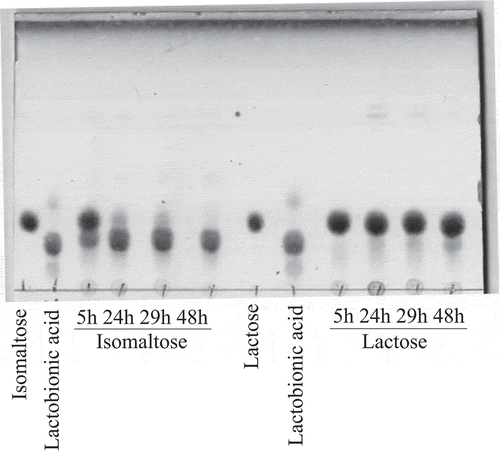
Screening of AAB that effectively oxidize isomaltose
To select AAB that effectively oxidize isomaltose, the resting cells of thirty acetic acid bacterial strains were incubated with 1% isomaltose at pH 5.5 for 24 h with shaking. The reaction mixtures were then analyzed using TLC (n = 1). The production of isomaltose oxide was detected with all of the AAB (data not shown). Five acetic acid bacterial strains (Gluconobacter spp., Gluconacetobacter sp., and Komagataeibacter sp.) were selected as high isomaltose-oxidizing acetic acid bacterial strains and three Acetobacter spp. were also selected as typical Acetobacter strains. Isomaltose and lactose were oxidized by the resting cells of these 8 AAB. The reaction mixtures were boiled for 5 min and analyzed by HPAEC-PAD (). The amounts of isomaltose oxide were calculated from calibration curves of lactobionic acid. All of the strains showed much higher isomaltose-oxidizing activities than lactose-oxidizing activities. NBRC 3285 cells produced the largest amount of isomaltose oxide. Similar to that observed with lactose, the oxidizing activities toward isomaltose of the Acetobacter spp. were lower than those of the other AAB.
Figure 2. Isomaltose and lactose oxidation by resting cells of various AAB.
AAB were cultivated in YPGL medium for 3 days. After the cultivation pH and A660 of the media were measured, the resting AAB were incubated with 1% isomaltose or lactose in 100 mM acetate buffer (pH 5.5) for 24 h with shaking. After boiling the reaction mixture for 5 min, the production of isomaltobionic acid (white bars) and lactobionic acid (black bars) was measured by HPAEC-PAD. The amounts of isomaltobionic acid are shown as the equivalent amounts of lactobionic acid. The experiments were performed 3–5 times and means ± standard deviations are plotted.
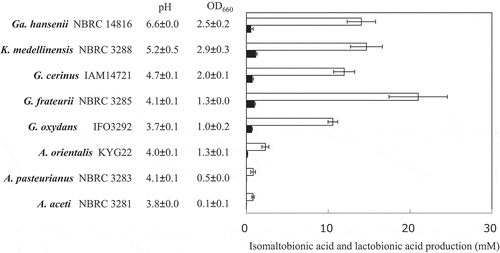
Oxidizing activities of AAB on oligosaccharides as assessed by the DCIP method
The substrate specificities on oligosaccharides having α- or β-1,6-glycosidic linkages were investigated using the DCIP method (). NBRC 3285, NBRC 3288, IFO 3292, and IAM 14721 resting cells were incubated with the oligosaccharides indicated in . These strains showed much higher oxidizing activities on isomaltose than on lactose or maltose. Similar to those on isomaltose, the oxidizing activities of the resting cells were higher on gentiobiose and melibiose than on lactose (). The resting cells of NBRC 3285 also oxidized isomaltooligosaccharide (degree of polymerization [DP] = 3–5), although this activity was lower than that on isomaltose.
Table 2. Substrate specificities of the resting cells of AABs on oligosaccharides.
Production of oligosaccharide oxides having β-1,6-glucosidic linkages by resting cells of NBRC 3285 and other AAB
The DCIP method is only an indirect measure of saccharide oxidation. Thus, we next investigated the oxidizing activities of NBRC 3285 cells on disaccharides by directly measuring the production of aldosaccharides using HPAEC-PAD (). Isomaltose, gentiobiose, and melibiose were oxidized by NBRC 3285 resting cells. The oxide production from isomaltose, melibiose, and gentiobiose (after 24 h) was almost the same as that from D-glucose (after 1 h) and was greater than that from lactose and maltose (after 24 h). The production of isomaltotriose oxide was lower than that of isomaltose oxide. However, compared with the production of lactobionic acid, maltobionic acid, and panose oxide (), a larger amount of isomaltose oxide was produced by the resting cells.
Figure 3. Production of various aldonic acids by the resting cells of NBRC 3285.
The resting cells of NBRC 3285 were incubated with 27.8 mM substrate in 100 mM acetate buffer (pH 5.5) at 27°C for 1 h (D-glucose) or 24 h (other saccharides) with shaking. The production of each saccharide oxide was measured by HPAEC-PAD. Oxidized products of D-glucose were measured using D-gluconic acid as a standard. The amounts of other oxides were measured as the equivalent amounts of lactobionic acid. The experiments were performed 3–5 times and means ± standard deviations are plotted.
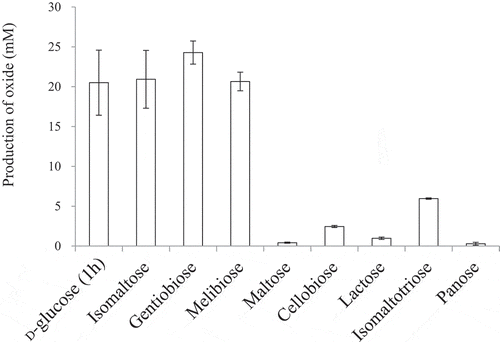
Next, we investigated the oxidation of oligosaccharides by other AAB (). The oxidizing activities of A. aceti NBRC 3281 and A. pasteurianus NBRC 3285 were too low to detect. However, similar to that observed with NBRC 3285 (), the resting cells of NBRC 3292, IAM 14721, NBRC 3288, and NBRC 14816 oxidized gentiobiose and melibiose to the same extent as isomaltose. The oxidation of isomaltotriose and panose by these AAB was lower than that of isomaltose.
Table 3. Production of aldonic acids by the resting cells of AAB.
Identification of the oxidized products
To identify the isomaltose oxide, it was purified from reaction mixtures of NBRC 3255 resting cells using anion exchange and gel filtration chromatography. Isomaltose oxide was hydrolyzed by boiling for 1 h in 1.0 M HCl. After neutralizing with 1M NaOH, the hydrolysis products were analyzed using D-gluconic acid (F-kit D-gluconic acid/glucono-δ-lactone, J.K. International Inc., Tokyo, Japan) and D-glucose (Glucose C2, Wako Pure Chemical Industries) assay kits. The assay results indicated that the isomaltose oxide contained D-glucose and D-gluconic acid residues. In the mass spectrum, the observed signal at m/z 357 was assigned to the negative ion of deprotonated isomaltose oxide [M − H]−. The product was also assigned by NMR spectroscopy ( and Supplemental figure S-1 to S-4). Studies have reported that the 13C chemical shift of C-1 of D-glucose residue and that of C’-6 of D-glucose residue of isomaltose was observed at 98.5/98.5 ppm and 66.5/66.5 ppm, respectively. In this work, a similar chemical shift was observed: 98.8 ppm for C-1 of D-glucose residue and 68.7 ppm for C’-6 of the D-gluconic acid residue of isomaltobionic acid, respectively. In contrast, C’-1 position of the glucose residue was significantly shifted to the lower magnetic field from 92.9/96.8 ppm to 179.2 ppm by the formation of a gluconic acid residue. All protons were assigned by the correlation with the corresponding carbon by 2D spectrometry (, Supplementary figure S-3). Both the mass spectrometry and NMR analyses clearly demonstrated the formation of isomaltobionic acid, whilst the α-1,6 linkage was retained in the course of the oxidation process. According to these data, the oxide was identified as isomaltobionic acid (α-d-glucopyranosyl-(1➝6)-d-gluconic acid).
Table 4. Assiment of isomaltobionic acid and melibionic acid.
Melibiose oxide was also prepared and purified by the same methods. Analysis of the hydrolysis products of melibiose oxide using a D-gluconic acid and D-galactose assay kit (F-kit Lactose/D-galactose J.K. International Inc.) indicated that the melibiose oxide consisted of D-galactose and D-gluconic acid residues. In the mass spectrometry analysis, the signal of the deprotonated molecular ion was observed at m/z 357. The product was also assigned by NMR spectroscopy in the same manner as isomaltobionic acid (, Supplemental figure S-5 to S-8). The linkage carbon (C-1 to C’-6) between D-galactose and D-glucose residues shifted a little, but significant lower magnetic field shift was observed at C’-1. All protons were assigned by the correlation with the corresponding carbon by 2D spectrometry (, Supplementary figure S-7). Both the mass spectrometry and NMR analyses clearly demonstrated the formation of melibionic acid (α-d-galactopyranosyl-(1➝6)-d-gluconic acid), whilst the α-1,6 linkage was maintained in the course of the oxidation process. In addition, the oxides of isomaltotriose and gentiobiose were determined to be isomaltotrionic acid (α-D-glucopyranosyl-(1➝6)-α-D-glucopyranosyl-(1➝6)-D-gluconic acid) and gentiobionic acid (β-D-glucopyranosyl-(1➝6)-D-gluconic acid), respectively, based on the m/z of the respective deprotonated molecular ions ([M − H]−) (m/z 519 and 357, respectively).
Identification of isomaltose-, melibiose-, and gentiobiose-oxidizing enzyme
In our previous study, the lactose-oxidizing enzyme was identified as m-GDH and the oxidation of lactose was inhibited (probably competitively) by D-glucose [Citation6]. Thus, the effects of D-glucose on isomaltose oxidation were investigated (). Isomaltose was oxidized by NBRC 3285 cells in the presence of 0–111 mM D-glucose and the production of isomaltobionic acid was found to be inhibited by D-glucose addition. Similar inhibition was observed with NBRC 3288, NBRC 3292, and IAM 14721 cells (n = 1, data not shown). These results suggest that the isomaltose-oxidizing enzyme is m-GDH. In our previous study, we prepared an m-GDH-deficient mutant of NBRC 3288 and identified the lactose-oxidizing enzyme as m-GDH because this mutant lacked the ability to oxidize lactose [Citation6]. Thus, in the present study, cells of the m-GDH-deficient mutant and wild-type strain were cultivated in SG medium that contained no D-glucose as a carbon source. The cells were incubated with oligosaccharides and the production of oxides was measured using HPAEC-PAD (). Similar to that observed with lactose and D-glucose, the enzyme-deficient cells produced no oxides from isomaltose, gentiobiose, or melibiose. These results indicate that the oxidizing enzyme for these oligosaccharides is m-GDH.
Figure 4. Effects of D-glucose addition on isomaltose oxidation.
Inhibition of isomaltose oxidation by D-glucose was investigated. The resting cells of NBRC 3285 were incubated with 27.6 mM (triangles) or 55.2 mM (squares) isomaltose and 0–111 mM D-glucose. After incubation for 1 h, isomaltobionic acid production was measured using HPAEC-PAD (left ordinate, white symbols, means ± standard deviations). The pH values of the reaction mixtures (right ordinate, black symbols, means) were also measured in two independent experiments.
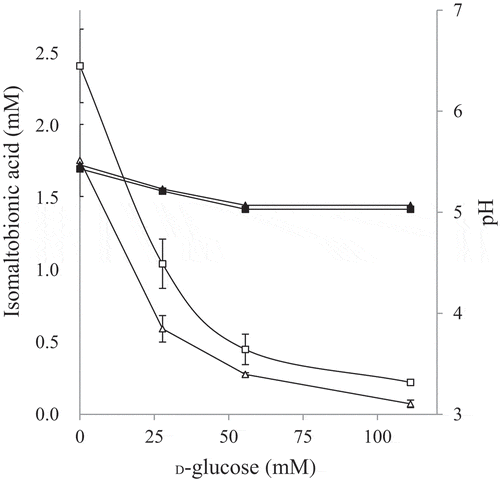
Figure 5. Oxidation of disaccharides by m-GDH-deficient NBRC 3288 mutant.
Resting cells of NBRC 3288 wild-type (Wild) and m-GDH-deficient mutant (ΔGDH) were prepared, and sugar-oxidizing activities were measured using HPAEC-PAD. The reaction times of D-glucose, lactose, and the other oligosaccharides were 1 h, 24 h, and 7 h, respectively. The graph shows the production of oligoaldonic acids by the wild-type strain (gray bars) and mutant strain (white bars, too low to see). The experiments were performed 3–5 times and means ± standard deviations are plotted.
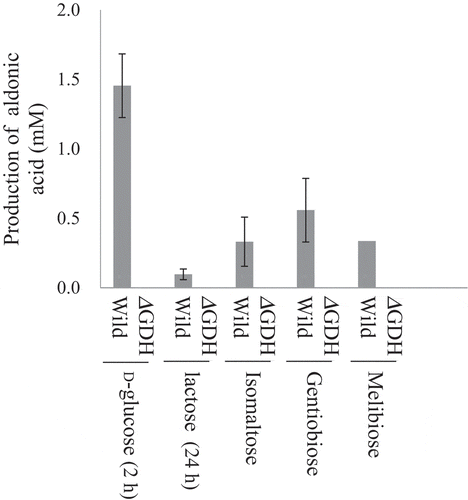
Assimilation of isomaltooligosaccharide and isomaltobionic acid
During the oxidation of isomaltooligosaccharide, any hydrolysis of isomaltooligosaccharide and isomaltobionic acid results in yield loss because hydrolysis not only leads to loss of substrate and product, respectively, but it also produces D-glucose, which inhibits the oxidation reaction (). Thus, we needed to investigate the degradation of isomaltose, isomaltotriose, and isomaltobionic acid by resting AAB. The products of hydrolysis (D-glucose and D-gluconic acid) were, however, produced in too little quantity to measure by HPAEC-PAD (data not shown). This likely occurred because the hydrolytic activity of NBRC 3285 cells was too low and the hydrolysis products were probably assimilated by the cells. Thus, NBRC 3285 cells were cultivated in medium that contained isomaltooligosaccharide or isomaltobionic acid as the only carbon source. The growth of NBRC 3285 cells in these media was measured by their absorbance at 660 nm ()). The AAB grew well in the media containing D-glucose. In contrast, we did not observe significant differences in proliferation between the control medium (water) and the media containing other disaccharides (isomaltobionic acid, lactose, and lactobionic acid). However, a slight proliferation was detected in the medium containing isomaltooligosaccharide, which was significantly higher than that of the control medium. The same experiments were repeated using NBRC 3288 cells ()), except that the assimilation of isomaltobionic acid was not tested because we could not obtain sufficient quantities of this substrate. NRBC 3288 cells also showed little or no assimilation of lactose and lactobionic acid, and they assimilated isomaltooligosaccharide slightly.
Figure 6. Assimilation of isomaltose and isomaltooligosaccharide by NBRC 3285 and NBRC 3288.
NBRC 3285 (a) and NBRC 3288 (b) were cultivated with isomaltose, lactose, lactobionic acid, isomaltooligosaccharide, D-glucose, or isomaltobionic acid. The growth of both of AAB (A660, white bars) and the production of acid (pH, gray circles) were measured to evaluate the assimilation of the sugars. The growth of NBRC 3288 in isomaltobionic acid was not determined. The experiments were performed 3–5 times (means ± standard deviations are plotted). Only the pH measurements for NBRC 3288 were carried out twice (means are plotted). The indicated statistical comparisons were performed using Student’s t tests.
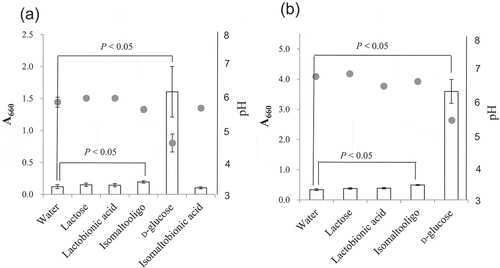
Discussion
Before our study, it had also been reported that m-GDH and AAB effectively oxidized D-glucose, but not lactose [Citation11–Citation14]. We have reported in a prior publication that most AAB demonstrate lactose-oxidizing activity [Citation5]. The oxidizing activities of AAB on lactose, however, were much lower than those on D-glucose. In our previous study, we also reported that the oxidizing activities toward disaccharides having α/β-(1,2)-, α/β-(1,3)-, and α/β-(1,4)-glycosidic linkages were as low as that on lactose () [Citation4,Citation5]. Thus, we had concluded that the oxidizing activities of AAB on disaccharides were much lower than that on D-glucose. In the current study, we tested the oxidation of isomaltose using resting AAB (, and ; ). The results of these investigations indicated that isomaltose-oxidizing activity is widely distributed in AAB and that the oxidizing activity of AAB on isomaltose is much higher than that on lactose.
Soluble quinoprotein glucose dehydrogenase (EC 1.1.99.17) is reported to oxidize not only D-glucose effectively, but also disaccharides such as lactose and maltose [Citation15,Citation16]. This enzyme is not membrane-embedded, has not been detected in AAB through immunological analysis [Citation17], and is unlikely to be the enzyme responsible for the activity observed in the present study. In our study, isomaltose-oxidizing activities were detected in the membrane fraction (), indicating that the isomaltose-oxidizing enzyme is embedded in the membrane. The results of suggest that the activities on lactose are correlated with those on isomaltose. Specifically, the strains having higher lactose-oxidizing activities showed higher isomaltose-oxidizing activities. Furthermore, the properties of the isomaltose-oxidizing enzyme were similar to those of the lactose-oxidizing enzyme. For example, both used DCIP as an electron acceptor [Citation2], possessed the same pH optimum (pH 5.5, data not shown) [Citation2], and were inhibited (probably competitively) by D-glucose () [Citation6]. These results suggest that the lactose-oxidizing enzyme (m-GDH) and isomaltose-oxidizing enzyme are the same enzyme. We also generated an m-GDH-deficient NBRC 3288 mutant [Citation6] and the isomaltose-oxidizing activity of the resting mutant cells was compared with that of the resting wild-type cells (). The deficient mutant showed no activities on isomaltose. In accordance with these results, we identified the isomaltose-oxidizing enzyme as m-GDH. Furthermore, the absence of isomaltobionic acid production by the mutant cells () indicated that no other enzyme capable of oxidizing isomaltose was expressed in NBRC 3288 cells.
AAB were also able to oxidize gentiobiose (), having a β-1,6-glucosidic linkage, and melibiose (), having an α-1,6-galactosidic linkage (; , , and 3). Experiments using the deficient mutant () also indicated that gentiobiose and melibiose are oxidized by m-GDH. The oxidizing activities on gentiobiose and melibiose were higher than those on lactose, maltose, and the other disaccharides shown in , and were almost the same as that on isomaltose (; , , and 3). These results indicate that m-GDH more effectively oxidizes disaccharides having α/β-1,6-glycosidic linkages than other disaccharides. This indication is supported by the following results. The enzymatic activities on panose (), having an α-1,4-glucosidic linkage at its reducing end, were lower than those on isomaltotriose, having an α-1,6-glucosidic linkage (). The comparison of gentiobiose and isomaltose oxidation indicates that the m-GDHs do not strictly distinguish β-1,6-glucosidic linkages from α-1,6 glucosidic linkages (). Isomaltose and melibiose differ in the positioning of their C-4 hydroxyl groups at their nonreducing sides. Almost the same activities on isomaltose and melibiose indicate that the recognition of those C-4 hydroxyl groups by m-GDH is not specific. However, activities of the membrane fraction of A. orientalis on D-galactose are 16% those of D-glucose [Citation4,Citation5]. Thus, we conclude that the recognition of the C-4 position is specific. The relative activities on D-galactose are different for AAB; some m-GDH proteins of AAB show no or low activity on D-galactose (7% of the activity on D-glucose) [Citation13,Citation18]. On the other hand, the activity on D-galactose of G. oxydans resting cells is approximately 1/2 of that on D-glucose [Citation19]. The activities of all AAB on isomaltose and melibiose were almost the same in the present study. However, most of these AAB were selected as strains that showed high oxidizing activity on saccharides having α/β-1,6 glycosidic linkages. Thus, further investigation is needed to draw firm conclusions about the effects of C-4 position on oxidizing activity.
As shown in and , the oxidizing activities on isomaltooligosaccharide (DP = 3–5) were lower than those on isomaltose (DP = 2). The activities on D-glucose (DP = 1) were the highest. Furthermore, the activities on panose (), having an α-1,4-glucosidic linkage at its reducing end, were lower than those on maltose (), also having an α-1,4-glucosidic linkage (). These results indicate that the activities of m-GDH are lower on sugars of longer length.
Compared with the oxidizing activities of AAB on D-glucose (a substrate of m-GDH), the activities on lactose were very low. We, however, found that AAB could tolerate high lactose concentrations (~30%) [Citation5]. Thus, for effective production of lactose, we incubated a large number of the cells with a high concentration of lactose (30%) [Citation5]. On the other hand, isomaltose is much more effectively oxidized by AAB than lactose, and its water solubility is higher than that of lactose (~30% for lactose). A solution of 75% isomaltose (in water) is commercially available. Although we need to investigate the tolerance of AAB to high concentrations of isomaltose (over 30%), the higher efficiency of oxidation and higher water solubility of isomaltose compared to that of lactose would probably reduce costs in the industrial production of isomaltobionic acid.
In our previous study, we oxidized lactose under anaerobic conditions and found that the production of lactobionic acid after 24 h was reduced to 65% of that under aerobic conditions [Citation9]. m-GDH is known not to use oxygen as an electron acceptor [Citation13]. However, as shown in past studies of resting cells of A. aceti and G. oxydans, sugar oxidation occurs with oxygen uptake and the oxidizing activities could be evaluated by this uptake [Citation14,Citation17]. Using a simple calculation, if we try to completely oxidize 30% isomaltose (877 mM) for 24 h, 305 μmol/L isomaltose is needed to be oxidized per minute using 153 μmol/L of oxygen. The oxygen content of a saturated aqueous solution at 25°C is 8.11 mg/L (253 μM), and oxygen is also used by other processes, including respiration. Thus, we were concerned about the disruption of oxygen levels by the addition of large numbers of the cells in the reaction. The supply of oxygen is not a problem in the oxidation of lactose [Citation14], but the efficiency of oxidation of isomaltose is higher and oxygen is probably consumed faster by oxidation of the latter. Thus, we need to be aware of the supply of oxygen during isomaltose oxidation by large numbers of cells for the effective production of isomaltobionic acid.
Isomaltose is expensive. For this reason, the use of isomaltose as a substrate in the industrial production of isomaltobionic acid is not practical. However, isomaltooligosaccharide syrup for industrial food production is available on the market. This syrup is one of the cheapest functional oligosaccharides, and could be a source of isomaltose and isomaltotriose for the industrial production of isomaltooligosaccharide oxide (a compound that we call isomaltooligoaldonic acid). Isomaltooligosaccharide syrup, however, contains considerable amounts of D-glucose. In this study, we found that D-glucose inhibited the oxidation of isomaltose (). Thus, the isomaltooligosaccharide syrup needs to have the D-glucose removed in order to be used. Fortunately, isomaltooligosaccharide syrup without D-glucose or with reduced D-glucose is available. However, the source of D-glucose in the reaction mixture is not limited to the isomaltooligosaccharide syrup itself, since D-glucose is also generated during the degradation of isomaltooligosaccharide or isomaltobionic acid. We attempted to investigate the hydrolysis of isomaltooligosaccharide and isomaltobionic acid by resting AAB. However, it was difficult to detect the hydrolysis products, D-glucose and D-gluconic acid, by HPAEC-PAD because hydrolytic activities were low and the hydrolysis products were probably assimilated by the AAB. Thus, we evaluated the hydrolytic activities by growing the AAB in medium containing isomaltooligosaccharide or isomaltobionic acid as the only carbon source (). Growth in the medium containing isomaltobionic acid was almost identical to that with no carbon source (i.e., in water). This result indicates that the hydrolytic activity of G. frateurii NBRC 3285 on isomaltobionic acid was nonexistent or extremely weak. In contrast, both G. frateurii NBRC 3285 and K. medellinensis NBRC 3288 assimilated isomaltooligosaccharide slightly. However, the assimilation (production of D-glucose) was probably too low to inhibit the oxidation of isomaltose and isomaltotriose.
Author contributions
T. Kiryu designed the study. T. Kiryu, T. Kiso, and H. Murakami discussed the results. T. Kiryu performed the experiments. T. Kiryu and H. Sato analyzed the data. T. Kiryu wrote the manuscript with the help of all other authors. All authors reviewed the manuscript.
supplment_data__final.pptx
Download MS Power Point (298.8 KB)Acknowledgments
We would like to thank Editage (www.editage.com) for English language editing.
Disclosure statement
T. Kiryu, T. Kiso, and H. Murakami received research support from Unitika Ltd. (Osaka, Japan).
Supplementary material
Supplemental data for this article can be accessed here.
Additional information
Funding
References
- Brommage R, Binacua C, Antille S, et al. Intestinal calcium absorption in rats is stimulated by dietary lactulose and other resistant sugars. J Nutr. 1993;123(12):2186–2194.
- Kiryu T, Kiso T, Nakano H, et al. Involvement of Acetobacter orientalis in the production of lactobionic acid in Caucasian yogurt (“Caspian Sea yogurt”) in Japan. J Daily Sci. 2009;92(1):25–34.
- Kiryu T, Yamauchi K, Masuyama A, et al. Optimization of lactobionic acid production by Acetobacter orientalis isolated from Caucasian fermented milk, “Caspian Sea yogurt”. Biosci Biotechnol Biochem. 2012;76:361–363.
- Kiryu T, Ooe K, Kimura T, et al. Sugar oxidation profiles of membrane-bound dehydrogenase from Acetobacter orientalis. Biocat Agric Biotech. 2015;1:262–263.
- Kiryu T, Kiso T, Nakano H, et al. Lactobionic and cellobionic acid production profiles of the resting cells of acetic acid bacteria. Biosci Biotechnol Biochem. 2015;79(10):1712–1718.
- Kiryu T, Kiso T, Koma D, et al. Identifying membrane-bound quinoprotein glucose dehydrogenase from acetic acid bacteria that produce lactobionic and cellobionic acids. Biosci Biotechnol Biochem. 2019;83(6):1171–1179.
- Kiryu T, Kiso T, Nakano F, et al. Development of disaccharide with aldonic acid such as lactobionic acid by resting cells and enzymes. Kagaku to Kogyo. 2013;87:55–59. (In Japanese).
- Ogino H, Azuma Y, Hosoyama A, et al. Complete genome sequence of NBRC 3288, a unique cellulose-nonproducing strain of Gluconacetobacter xylinus isolated from vinegar. J Bacteriol. 2011;193(24):6997–6998.
- Dubois M, Gilles KA. Colorimetric method for determination of sugars and related substances. Anal Chem. 1956;28:350–356.
- Bock K, Pedersen C, Pedersen H. Carbon-13 nuclear magnetic resonance data for oligosaccharides. Adv Carbohydr Chem Biochem. 1984;42:193–225.
- Matsushita K, Shinagawa E, Adachi O, et al. Quinoprotein D-glucose dehydrogenases in Acinetobacter calcoaceticus LMD 79.41: purification and characterization of the membrane-bound enzyme distinct from the soluble enzyme. Antonie Van Leeuwenhoek. 1989;56(1):63–72.
- Ameyama M, Nonobe M, Shinagawa E, et al. Purification and characterization of the quinoprotein D-glucose dehydrogenase apoenzyme from Escherichia coli. Agric Biol Chem. 1986;50(1):49–57.
- Ameyama M, Shinagawa E, Matsushita K, et al. D-Glucose dehydrogenase of Gluconobacter suboxydans: solubilization, purification and characterization. Agric Biol Chem. 1981;45(4):851–861.
- DeLey J, Schell J. Oxidation of several substrates by Acetobacter aceti. J Bacteriol. 1959;77(4):445–451.
- Dokter P, Frank J, Duine JA, et al. Purification and characterization of quinoprotein glucose dehydrogenase from Acinetobacter calcoaceticus L.M.D. 79.41. Biochem J. 1986;239(1):163–167.
- Southall SM, Doel JJ, Richardson DJ, et al. Soluble aldose sugar dehydrogenase from Escherichia coli: a highly exposed active site conferring broad substrate specificity. J Biol Chem. 2006;281(41):30650–30659.
- Matsushita K, Toyama H, Ameyama M, et al. Soluble and membrane-bound quinoprotein D-glucose dehydrogenases of the Acinetobacter calcoaceticus: the binding process of PQQ to the apoenzymes. Biosci Biotechnol Biochem. 1995;59(8):1548–1555.
- Meyer M, Schweiger P, Deppenmeier U. Effects of membrane-bound glucose dehydrogenase overproduction on the respiratory chain of Gluconobacter oxydans. Appl Microbiol Biotechnol. 2013;97(8):3457–3466.
- Peters B, Mientus M, Kostner D, et al. Characterization of membrane-bound dehydrogenases from Gluconobacter oxydans 621H via whole-cell activity assays using multideletion strains. Appl Microbiol Biotechnol. 2013;97(14):6397–6412.