ABSTRACT
Cellulose nanofiber (CN) consumption with exercise could be a potential strategy to control obesity. Here, we studied the effects of CN supplementation and voluntary exercise on obesity and gut microbiota in high-fat diet (HFD)-fed mice. Consumption of CN increased voluntary wheel running activity. CN intake and exercise together suppressed the increase in body weight and fat mass, and improved glucose tolerance. The fecal gut microbiota was analyzed by sequencing 16S ribosomal RNA genes. Principal component analysis revealed a shift in the microbiota composition resulting from exercise, but not from CN supplementation. Erysipelotrichaceae and Rikenellaceae decreased with exercise. Exercise also increased Ruminococcaceae, whereas exercise and CN intake together increased Eubacteriaceae. These two families are butyrate producers. Exercise increased the amount of acetate in the cecum. These results suggest that CN consumption improves exercise performance and exerts anti-obesity effects by modulating the balance of the gut microbiota.
GRAPHICAL ABSTRACT
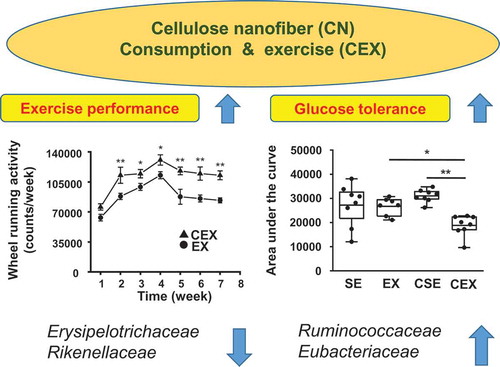
CN intake improves exercise performance and suppresses obesity by controlling the balance of the gut microbiota.
The rate of obesity is rising worldwide and it is associated with high-fat diet (HFD) and low level of dietary fibers (DFs) [Citation1–Citation3]. Clinical studies have indicated that highly viscous soluble DFs, such as β-glucan, psyllium, and guar gum, improve glycemic control and lower the levels of cholesterols. These beneficial effects are not provided by non-viscous soluble DFs, such as fructooligosaccharides and inulin, and insoluble DFs, such as cellulose and hemicellulose [Citation4–Citation7]. Thus, cellulose nanofibers (CNs) may help control obesity because of their pronounced viscosity [Citation8,Citation9].
In a prior study, CNs were prepared from plant cellulose and their inhibitory effects on postprandial increases in blood glucose and insulin were investigated in mice. The authors described that the postprandial increase in blood insulin was suppressed by CN supplementation in a concentration-dependent manner [Citation10]. In our previous study [Citation11], the effects of CN supplementation on obesity in HFD-fed mice were studied. The HFD-induced increase in fat mass and body weight was suppressed by 0.2% CN intake. Principal component analysis (PCA) showed that feeding HFD resulted in a shift of the microbiota composition, which was reversed with 0.2% CN consumption. Moreover, the relative abundance of Lactobacillaceae increased with increasing CN supplementation. These results suggested that CN intake could be of therapeutic value for obesity due to the control of the balance of the gut microbiota [Citation11].
In addition to the consumption of highly viscous DF, exercise training is also a good therapeutic method for obesity [Citation12]. Recent studies have suggested that exercise training influences the composition of the gut microbiota and increases the relative abundance of butyrate-producing taxa in the gut in mice and humans [Citation13–Citation15]. This exercise associated increase in butyrate might be beneficial in suppressing obesity [Citation14,Citation15]. Another study reported the effects of physical activity on gut microbiota collected cecum contents from mice that were exercised by voluntary wheel running and from sedentary mice, and transplanted the contents to antibiotic-treated mice [Citation16]. Mice receiving cecum contents from the exercised mice displayed higher levels of physical activity than mice receiving cecum contents from the sedentary mice. The findings indicated that exercise-induced high physical activity may be transmitted via gut microbiota [Citation16]. However, the beneficial effects of CN intake and exercise on obesity and gut microbiota remain unclear.
Presently, we studied the effect of CN supplementation and exercise on obesity in a HFD-fed mouse model. Furthermore, we analyzed the gut microbiota composition and short-chain fatty acids (SCFAs) that included butyrate, propionate, and acetate, to elucidate the effect of CN intake and exercise on the intestinal microbiota.
Materials and methods
Materials
The HFD (60% kcal fat; D12492G) with no added cellulose was purchased from Research Diets (New Brunswick, NJ, USA). CN (BiNFi-sTM, WFo-10002) was obtained from Sugino Machine (Uozu, Japan).
Animal experiments
Eight-week-old male C57BL/6N mice were obtained from Clea Japan (Tokyo, Japan). Each mouse was housed in a temperature-controlled room (22 ± 1°C, 12-h light/dark cycle) using conventional conditions. Experiments involving the mice, including the procedures performed, were approved by the Institutional Animal Care and Use Committee of Kawasaki University of Medical Welfare (#18-015). After three-day acclimatization, the mice were randomly divided into CN-untreated sedentary (SE, n = 8) and exercise (EX, n = 8) groups and CN-treated sedentary (CSE, n = 8) and exercise (CEX, n = 8) groups. The EX and CEX mice were provided a wheel (10 × 23 × 10 cm cage with wide 5.5 cm × φ 22 cm; Natsume Seisakusho, Tokyo, Japan) that was available for unlimited use. All groups were administered HFD. The CSE and CEX groups were additionally provided 0.2% CN in their diet via drinking water, with a water bottle throughout the experiment. Glucose tolerance test (GTT) was conducted at week 7 as described previously [Citation11]. On the final day of the experiment, mice were rendered unconscious by isoflurane exposure and sacrificed by cervical dislocation. The cecum and epididymal, visceral, and subcutaneous fat were collected and weighed. The fecal contents were collected from the proximal colon. Collected samples were stored at −80°C until use.
Bacterial DNA sequencing and taxonomic analysis
Bacteria were recovered from lysates of the fecal samples using the FastDNA Spin Kit for Soil (MP Biomedicals, Santa Ana, CA, USA). PCR and sequencing was performed using the Miseq platform (Illumina, San Diego, CA, USA) as described previously [Citation17]. Taxonomic analysis of the 16S rDNA sequences was conducted by the TechnoSuruga laboratory (Shizuoka, Japan) based on the Ribosomal Database Project using the Metagenome@KIN analysis software (World Fusion, Tokyo, Japan) [Citation18].
SCFAs
SCFAs in cecum contents were determined using gas chromatography as previously reported [Citation19].
Statistical analysis
Statistical calculations and principal component analysis (PCA) were conducted using Origin 2019 software (OriginLab, Northampton, MA, USA). Data for wheeling activity was analyzed with the two-way repeated-measures analysis of variance (ANOVA) followed by Sheffé’s post-hoc test. The remaining data were analyzed using two-way ANOVA followed by Tukey’s post-hoc tests. The data were considered significant at P < 0.05.
Results
Feed, water, and CN intake
Feed consumption was 2.37 ± 0.03, 2.75 ± 0.06, 2.35 ± 0.05, and 2.77 ± 0.04 g/day for CN-untreated sedentary and exercise mice and CN-treated sedentary and exercise mice, respectively. Feed intake was significantly higher in the exercise groups compared with that in the sedentary groups (P < 0.0001). Water consumption was 8.84 ± 0.48, 9.32 ± 0.52, 7.73 ± 0.43, and 11.1 ± 0.47 mL/day for CN-untreated sedentary and exercise mice and CN-treated sedentary and exercise mice, respectively. As mice were provided CN via drinking water, CN intake was estimated to be 15.2 ± 0.9 and 21.3 ± 0.8 mg/day for CN-treated sedentary and exercise mice, respectively.
Voluntary activity, body weight, fat accumulation, and GTT
Voluntary wheel running activity per week was determined for CN-untreated and treated exercise groups ()). The activity of CN-treated exercise mice was significantly greater than that of CN-untreated exercise mice throughout the experiment (P < 0.05). In contrast, body weight of each group was also measured per week for 7 weeks ()). Body weight gain was determined after 7 weeks ()) and the results of the ANOVA were as follows: CN treatment, F (1,27) = 11.34, P < 0.01, exercise, F (1,27) = 46.75, P < 0.001. Thus, body weight increase was inhibited by both CN-treatment and exercise.
Figure 1. Effects of cellulose nanofiber (CN) intake and exercise on wheel running activity and body weight in high-fat diet-fed mice.
(a) Wheel running activity in exercised mice. (b) Body weight change over 7 weeks. SE, CN-untreated sedentary group; EX, CN-untreated exercise group; CSE, CN-treated sedentary group; CEX, CN-treated exercise group. The data are presented as the means ± SEM; *P < 0.05; **P < 0.01.
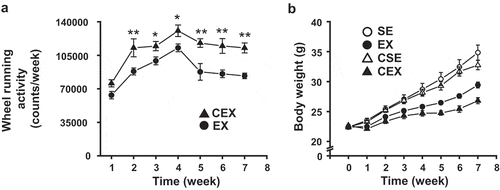
Figure 2. Effects of cellulose nanofiber (CN) intake and exercise on body weight gain and fat accumulation in high-fat diet-fed mice.
(a) Increase in body weight after 7 weeks. Changes in (b) epididymal fat mass, (c) visceral fat mass, and (d) subcutaneous fat mass. SE, CN-untreated sedentary group; EX, CN-untreated exercise group; CSE, CN-treated sedentary group; CEX, CN-treated exercise group. *P < 0.05; ***P < 0.001.
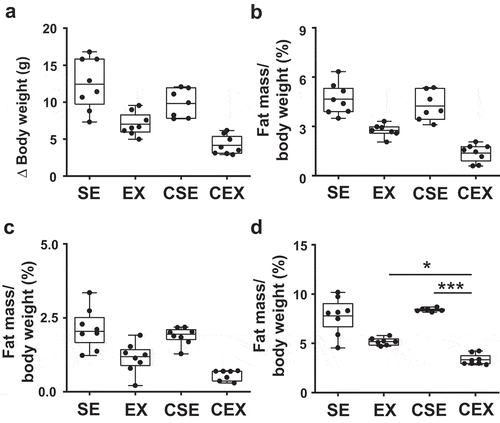
The accumulation of epididymal, visceral, and subcutaneous fat was measured (). The results of the ANOVA were as follows: CN treatment, F (1,26) = 11.23, P < 0.01, exercise, F (1,26) = 78.73, P < 0.001 for epididymal fat, CN treatment, F (1,26) = 8.96, P < 0.01, exercise, F (1,26) = 46.69, P < 0.001 for visceral fat, and exercise, F (1,25) = 96.51, P < 0.001, CN treatment × exercise interaction, F (1,25) = 9.74, P < 0.01 for subcutaneous fat. The amount of epididymal and visceral fat was lower with CN treatment and exercise. In contrast, the mass of subcutaneous fat was inhibited only with exercise. The mass of subcutaneous fat in CN-treated exercise mice was decreased compared with that of the CN-untreated exercise mice (P < 0.05) and CN-treated sedentary mice (P < 0.001) ()).
GTTs were conducted ()) and the area under the curve (AUC) was determined ((b)). The ANOVA results were as follows: exercise, F (1,27) = 10.79, P < 0.01, CN treatment × exercise interaction, F (1,27) = 9.14, P < 0.01. The increase in AUC was inhibited with exercise. The AUC in CN-treated exercise mice was lower than that in CN-untreated exercise mice (P < 0.05) and CN-treated sedentary mice (P < 0.01) ()).
Figure 3. Effects of cellulose nanofiber (CN) intake and exercise on glucose tolerant test (GTT) results in high-fat diet-fed mice.
(a) GTTs at week 7. (b) Area under the curves of blood glucose. SE, CN-untreated sedentary group; EX, CN-untreated exercise group; CSE, CN-treated sedentary group; CEX, CN-treated exercise group. *P < 0.05; **P < 0.01.
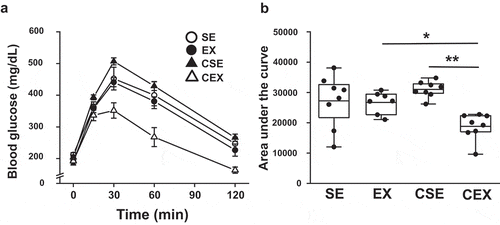
Gut microbiota composition in mice
PCA ()) and analysis of the composition of the gut microbiota ()) were conducted at the family level. In the PCA, the microbiota compositions were not separated on the PC1 axis (26.8% of explained variance), whereas those of the sedentary and exercise groups were separated on the PC2 axis (23.7% of explained variance).
Figure 4. Effects of cellulose nanofiber (CN) intake and exercise on the gut microbiota composition in high-fat diet-fed mice at the family level.
(a) Principal component analysis (PCA). (b) Taxonomic distribution of gut microbiota. SE, CN-untreated sedentary group; EX, CN-untreated exercise group; CSE, CN-treated sedentary group; CEX, CN-treated exercise group.
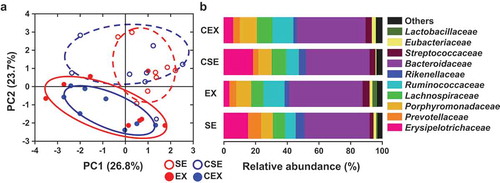
Significant differences were observed in four gut bacteria at the family levels: Erysipelotrichaceae, Ruminococcaceae, Rikenellaceae, and Eubacteriaceae (). The ANOVA results were as follows: exercise, F (1,28) = 25.16, P < 0.001 for Erysipelotrichaceae, exercise, F (1,25) = 15.35, P < 0.001 for Ruminococcaceae, exercise, F (1,28) = 14.11, P < 0.001 for Rikenellaceae, and exercise, F (1,25) = 16.85, P < 0.001, CN treatment × exercise interaction, F (1,25) = 11.31, P < 0.01 for Eubacteriaceae. The relative abundance of Erysipelotrichaceae and Rikenellaceae decreased with exercise, whereas the relative abundance of Ruminococcaceae increased with exercise. Furthermore, the relative abundance of Eubacteriaceae increased with exercise, and was higher in the CN-treated exercise mice than in the CN-untreated exercise mice (P < 0.05) and CN-treated sedentary mice (P < 0.001) ()).
Figure 5. Effects of cellulose nanofiber (CN) intake and exercise on the gut microbiota composition in high-fat diet-fed mice.
Results for (a) Erysipelotrichaceae, (b) Ruminococcaceae, (c) Rikenellaceae, and (d) Eubacteriaceae. SE, CN-untreated sedentary group; EX, CN-untreated exercise group; CSE, CN-treated sedentary group; CEX, CN-treated exercise group. *P < 0.05; ***P < 0.001.
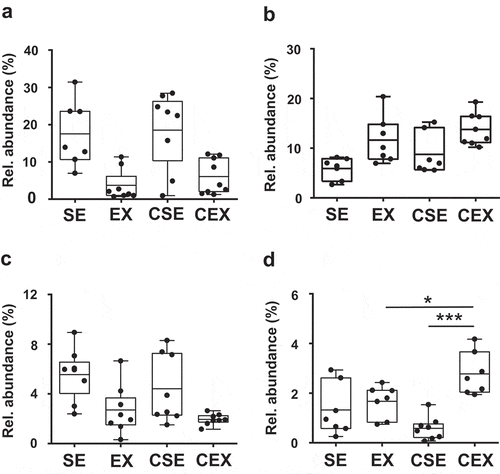
SCFAs
The amounts of acetate, propionate, butyrate, and valerate in cecum contents were determined (). The results of the ANOVA comparing acetate content were as follows: exercise, F (1,22) = 18.48, P < 0.001. The amount of acetate increased with exercise. There were no significant differences in the amounts of propionate, butyrate, and valerate.
Discussion
CN intake and exercise suppressed HFD-induced obesity in mice, as evidenced by reduced body weight gain and improved glucose tolerance. In addition, voluntary wheel running activity increased with CN treatment and accumulation of epididymal, visceral, and subcutaneous fat was inhibited with exercise. The amount of subcutaneous fat in CN-treated exercise mice was also suppressed more than that in CN-untreated exercise and CN-treated sedentary mice. These results suggest that CN consumption increases exercise performance, resulting in the control of obesity. In previous studies involving rodent models, exercise performance was improved by supplementation with resveratrol [Citation20] and fermented milk Kefir [Citation21].
We previously investigated the effects of CN consumption on HFD-induced obesity in mice. The increase in body weight and fat mass in 0.2% CN-treated HFD-fed mice was suppressed compared with CN-untreated HFD-fed mice [Citation11]. However, in this study, we did not observe this anti-obesity effects in CN-treated sedentary mice although anti-obesity effects were observed with CN treatment. In addition, the amount of CN intake in 0.2% CN-treated HFD-fed mice was 9.34 ± 1.07 mg/day in our former study. In this study, the amount of CN consumption was 15.2 ± 0.9 mg/day for CN-treated sedentary mice. Thus, the amount of CN consumption by CN-treated sedentary mice in this study was higher than that in our previous study. This discrepancy between our two studies suggests that mice did not consume CN as we recorded in this study. One possible reason is that this study was conducted with one mouse per cage, while the previous study was performed with four mice per cage. The loss of CN dispersions could not be ignored, although we changed water bottles carefully in this study.
Recent studies in animal models and humans showed that Western-style diets (WDs) and HFD are accompanied by changes in gut microbiota composition, which connect obesity with obesity-related diseases [Citation1–Citation3]. Exercise also alters the gut microbiota independent of diet [Citation14,Citation15]. In the present study, in the PCA plot at family level, PC1 indicated that the microbiota composition was not changed by CN consumption, while PC2 revealed a shift in microbiota structure resulting from exercise.
Our results for the composition of gut microbiota also indicated that Erysipelotrichaceae and Rikenellaceae decreased with exercise. Previous studies suggest that Erysipelotrichacea is correlated to lipid metabolism and the related diseases [Citation22]. Turnbaugh et al. investigated the connection of gut microbial ecology and consumption of WD. They observed a high level of Erysipelotrichaceae in WD-induced obese mice [Citation23]. Fleissner et al. also studied the effect of HFD and WD in mice. They found that Erysipelotrichaceae increased in HFD and WD-induced obese mice [Citation24]. In addition, Zhang et al. reported that the proportion of Erysipelotrichaceae increased in obese individuals in humans [Citation25]. Moreover, plant sterol esters reduced the abundance of Erysipelotrichaceae and Coriobacteriaceae in hamsters. The abundance of these bacteria was highly correlated with cholesterol metabolism [Citation26]. On the other hand, Rikenellaceae is reported to be related to diet-induced obesity [Citation27–Citation29]. Daniel et al. reported that HFD consumption increased Rikenellaceae in mice [Citation27]. In addition, a genus in Rikenellaceae was correlated to type-2 diabetes in humans [Citation28]. Welly et al. also investigated the effect of HFD and exercise on gut microbiota in rats. They found that Rikenellaceae decreased with exercise compared with sedentary rats [Citation29].
The major products from gut microbiota are SCFAs that can activate G protein-coupled receptors (GPR), such as GPR43 and GPR41, and thereby exert beneficial effects on health [Citation30,Citation31]. In the present study, the amount of acetate in the cecum was high with exercise compared with sedentary mice. It was reported that consumption of acetate improved glucose tolerance and protected against obesity [Citation1]. Fukuda et al. also reported that acetate produced by bifidobacteria in the gut improved intestinal defense against Escherichia coli O157 in mice [Citation32]. In contrast, Matsumoto et al. reported that exercise resulted in an increase of butyrate in rat cecum [Citation13]. Another study provided conformation and also showed that the butyrate-producing bacteria increased with exercise [Citation14]. In this study, exercise increased the relative abundance of Ruminococcaceae, while CN intake and exercise together increased the relative abundance of Eubacteriaceae. These two bacteria families are butyrate producers [Citation33,Citation34]. However, there no significant difference in the amount of butyrate in cecum contents was evident. Further studies are warranted to elucidate the anti-obesity effects of CN consumption and exercise.
In summary, our data provide evidence that CN intake increases exercise performance and has beneficial effects on HFD-induced obesity through regulation of the balance of the gut microbiota. Analysis of the gut microbiota revealed decreased relative abundance of Erysipelotrichaceae and Rikenellaceae with exercise. Exercise also increased the relative abundance of Ruminococcaceae, and exercise and CN intake together increased the relative abundance of Eubacteriaceae. Furthermore, exercise increased the amount of acetate in the cecum, while CN intake did not.
Author contributions
TN and HY conceived and designed the experiments, and performed the experiments; TN wrote, and HY reviewed and edited the manuscript.
Acknowledgments
We thank TechnoSuruga laboratory (Shizuoka, Japan) for taxonomic analysis of the 16S rRNA gene sequences and determination of SCFAs. We thank Editage (www.editage.jp) for English language editing.
Disclosure statement
No potential conflict of interest was reported by the authors.
Additional information
Funding
References
- Makki K, Deehan EC, Walter J, et al. The impact of dietary fiber on gut microbiota in host health and disease. Cell Host Microbe. 2018;23:705–715.
- Derrien M, Veiga P. Rethinking diet to aid human-microbe symbiosis. Trends Microbiol. 2017;25:100–112.
- Turnbaugh PJ. Microbes and diet-induced obesity: fast, cheap, and out of control. Cell Host Microbe. 2017;21:278–281.
- Cassidy YM, McSorley EM, Allsopp PJ. Effect of soluble dietary fibre on postprandial blood glucose response and its potential as a functional food ingredient. J Funct Food. 2018;46:423–439.
- Gill S, Chater PI, Wilcox MD, et al. The impact of dietary fibres on the physiological processes of the large intestine. Bioact Carbohydr Dietary Fibre. 2018;16:62–74.
- McRorie JW, McKeown NM. Understanding the physics of functional fibers in the gastrointestinal tract: an evidence-based approach to resolving enduring misconceptions about insoluble and soluble fiber. J Acad Nutr Diet. 2017;117:251–264.
- Mackie A, Bajka B, Rigby N. Roles for dietary fibre in the upper GI tract: the importance of viscosity. Food Res Internat. 2016;88:234–238.
- Nechyporchuk O, Belgacem MN, Bras J. Production of cellulose nanofibrils: a review of recent advances. Ind Crop Prod. 2016;93:2–25.
- Watanabe Y, Kitamura S, Kawasaki K, et al. Application of a water jet system to the pretreatment of cellulose. Biopolym. 2011;95:833–839.
- Shimotoyodome A, Suzuki J, Kumamoto Y, et al. Regulation of postprandial blood metabolic variables by TEMPO-oxidized cellulose nanofibers. Biomacromol. 2011;12:3812–3818.
- Nagano T, Yano H. Dietary cellulose nanofiber modulates obesity and gut microbiota in high-fat-fed mice. Manuscript submitted for publication.
- Colberg SR, Sigal RJ, Yardley JR, et al. Tate, physical activity/exercise and diabetes: a position statement of the American Diabetes Association. Diabetes Care. 2016;39:2065–2079.
- Matsumoto M, Inoue R, Tsukahara T, et al. Voluntary running exercise alters microbiota composition and increases n-butyrate concentration in the rat cecum. Biosci Biotechnol Biochem. 2008;72:572–576.
- Mailing LJ, Allen JM, Buford TW, et al. Exercise and the gut microbiome: A review of the evidence, potential mechanisms, and implications for human health. Exerc Sport Sci Rev. 2019;47:75–85.
- O’Sullivan O, Cronin O, Clarke SF, et al. Exercise and the microbiota. Gut Microb. 2015;6:131–136.
- Oyanagi E, Uchida M, Kremenik MJ. Altered gut microbiota by voluntary exercise induces high physical activity in high-fat diet mice. J Phys Fit Sport Med. 2018;7:81–85.
- Nagano T, Katase M, Tsumura K. Dietary soyasaponin attenuates 2,4-dinitrofluorobenzene-induced contact hypersensitivity via gut microbiota in mice. Clin Exp Immunol. 2019;195:86–95.
- Takahashi S, Tomita J, Nishioka K, et al. Development of a prokaryotic universal primer for simultaneous analysis of bacteria and archaea using next-generation sequencing. PLoS One. 2014;9:e105592.
- García-Villalba R, Giménez-Bastida JA, García-Conesa MT, et al. Alternative method for gas chromatography-mass spectrometry analysis of short-chain fatty acids in faecal samples. J Sep Sci. 2012;35:1906–1913.
- Hart N, Sarga L, Csende Z, et al. Resveratrol enhances exercise training responses in rats selectively bred for high running performance. Food Chem Toxicol. 2013;61:53–59.
- Hsu YJ, Huang WC, Lin JS, et al. Kefir supplementation modifies gut microbiota composition, reduces physical fatigue, and improves exercise performance in mice. Nutrient. 2018;10:862.
- Kaakoush NO. Insights into the role of Erysipelotrichaceae in the human host. Front Cell Infec Microbiol. 2015;5:84.
- Turnbaugh PJ, Bäckhed F, Fulton L, et al. Diet-induced obesity is linked to marked but reversible alterations in the mouse distal gut microbiome. Cell Host Microbe. 2008;3:213–223.
- Fleissner CK, Huebel N, Abd El-Bary MM, et al. Absence of intestinal microbiota does not protect mice from diet-induced obesity. Br J Nutr. 2010;104:919–929.
- Zhang H, DiBaise JK, Zuccolo A, et al. Human gut microbiota in obesity and after gastric bypass. Proc Natil Acad Sci USA. 2009;106:2365–2370.
- Martinez I, Perdicaro DJ, Brown AW, et al. Diet-induced alterations of host cholesterol metabolism are likely to affect the gut microbiota composition in hamsters. Appl Environm Microbial. 2013;79:516–524.
- Daniel H, Gholami AM, Berry D, et al. High-fat diet alters gut microbiota physiology in mice. Isme J. 2013;8:295–308.
- Jiang W, Wu N, Wang X, et al. Dysbiosis gut microbiota associated with inflammation and impaired mucosal immune function in intestine of humans with non-alcoholic fatty liver disease. Sci Rep. 2015;5:8096.
- Welly RJ, Liu TW, Zidon TM, et al. Comparison of diet versus exercise on metabolic function and gut microbiota in obese rats. Med Sci Sport Exerc. 2016;48:1688–1698.
- Koh A, De Vadder F, Kovatcheva-Datchary P, et al. From dietary fiber to host physiology: short-chain fatty acids as key bacterial metabolites. Cell. 2016;165:1332–1345.
- Kasubuchi M, Hasegawa S, Hiramatsu T, et al. Dietary gut microbial metabolites, short-chain fatty acids, and host metabolic regulation. Nutrient. 2015;7:2839–2849.
- Fukuda S, Toh H, Hase K, et al. Bifidobacteria can protect from enteropathogenic infection through production of acetate. Nature. 2011;469:543–547.
- Vital M, Howe AC, Tiedje JM. Revealing the bacterial butyrate synthesis pathways by analyzing (meta)genomic data. MBio. 2014;5:e00889.
- Louis P, Flint HJ. Diversity, metabolism and microbial ecology of butyrate-producing bacteria from the human large intestine. FEMS Microbiol Lett. 2009;294:1–8.