ABSTRACT
Berberine (BBR) is a protoberberine alkaloid extracted from plants such as Coptis japonica (Ranunculaceae). In a previous report, we demonstrated the existence of a 11-hydroxylation pathway employed by BBR-utilizing bacteria for metabolism of BBR. In the present study, we report the identification of the genes brhA, brhB, and brhC as encoding a multicomponent BBR 11-hydroxylase in Burkholderia sp. strain CJ1. BrhA is belonging to the Rieske non-heme iron oxygenase (RO) family, a class of enzymes known to catalyze the first step in bacterial aromatic-ring hydroxylation. We further demonstrate that BrhA activity requires BrhB (ferredoxin reductase) and BrhC (ferredoxin) as electron transport chain components. A BLAST search revealed that BrhA exhibits 38% and 33% sequence identity to dicamba O-demethylase (DdmC; AY786443) and chloroacetanilide herbicides N-dealkylase (CndA; KJ461679), respectively. To our knowledge, this work represents the first report of a bacterial oxygenase catalyzing the metabolism of a polycyclic aromatic-ring alkaloid.
Abbreviations: BBR: berberine; D-BBR: demethyleneberberine; H-BBR: 11-hydroxyberberine; HD-BBR: 11-hydroxydemethyleneberberine; HDBA: 2-hydroxy-3,4-dimethoxybenzeneacetic acid; PAL: palmatine; H-PAL: 11-hydroxypalmatine; BRU: berberrubine; Fd: ferredoxin; FdR: ferredoxin reductase; ETC: electron transport chain
Graphic abstract
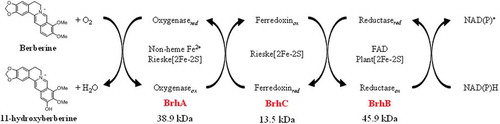
Components and functions of the BBR 11-hydroxylase from Burkholderia sp. Strain CJ1.
Berberine (BBR) is a well-known alkaloid of the protoberberine-type that can be extracted from plants such as Coptis japonica (Ranunculaceae) [Citation1] and Phellodendron amurense (Rutaceae) [Citation2]. BBR has been shown to possess anti-microbial activity against various pathogenic microorganisms [Citation3,Citation4] as well as anti-malarial activity [Citation5,Citation6]; in recent years, the compound has been reported to exhibit an inhibitory effect on tumor growth [Citation7,Citation8], and a therapeutic effect against diabetes (e.g. [Citation9,Citation10],). BBR derivatives have been investigated the biological activity. For example, Chow YL et al. [Citation11] reported the anti-adipogenic effects on 3T3-L1 adipocytes by 13-methylberberine which alkylation at position 13 of BBR. It was also reported that another BBR derivative may contribute to the prevention of osteoporosis [Citation12]. As above, the structural modification method of BBR has indicated potentially efficacy for getting stronger biological activity.
In a previous report, we isolated four BBR-utilizing bacteria, including Rhodococcus sp. strain BD7100 [Citation13], Sphingobium sp. strain BD3100 [Citation14], Arthrobacter sp. strain GBD-1, and Burkholderia sp. strain CJ1 [Citation15]. We further demonstrated the existence of two distinct pathways for the bacterial metabolism of BBR () [Citation13–Citation15]: one, which is used by all 4 strains, employs demethylenation via demethyleneberberine (D-BBR); the second, which is used by two of the 4 strains (BD3100 and CJ1), employs 11-hydroxylation via 11-hydroxyberberine (H-BBR). Additionally, we reported the isolation of a BBR demethylenase-encoding gene (brdA) and suggested that BrdA shares a common origin with the T-protein of the glycine cleavage system (GcvT) involved in C1 metabolism of glycine [Citation15].
Figure 1. Berberine and palmatine metabolism by berberine-utilizing bacteria. BBR is degraded into D-BBR as the common BBR metabolite in four distinct strains. As a gene that cleaves the methylenedioxy ring of BBR, BD7100 and GBD-1 have a brdA, and BD3100 and CJ1 have two homologous genes, brdA1 and brdA2. BD3100 and CJ1 generated a BBR 11-hydroxylation product, and BD3100 has a PAL 11-hydroxylation pathway. The 11-hydroxylation pathway of BBR is the focus of this study. BBR, berberine; D-BBR, demethyleneberberine; H-BBR, 11-hydroxyberberine; HD-BBR, 11-hydroxydemethyleneberberine; HDBA, 2-hydroxy-3,4-dimethoxybenzeneacetic acid; PAL, palmatine; H-PAL, 11-hydroxypalmatine. Solid arrows indicate the degradation pathway by BBR-utilizing bacteria, and the dashed line indicates the deduced degradation pathway. Arrows with multiple heads indicates multiple metabolic stages.
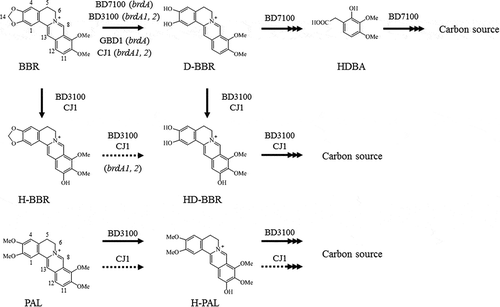
On the other hand, the genes encoding components of the 11-hydroxylation pathway for BBR metabolism had not been identified. Therefore, we sought to identify genes involved in 11-hydroxylation using a BD3100 transposon mutant library. These mutants were generated by random mutagenesis of the BD3100 genome with the EZ-Tn5™ transposon. Specifically, we screened the transposon mutant library for isolates that had lost the ability to degrade palmatine (PAL); this screen was performed using 96-well plates with LB medium containing 0.5 mM PAL. PAL was utilized because this compound is not metabolized by demethylenation in strain BD3100 (). One mutant strain, designated T443, was identified based on loss of the ability to degrade PAL; detailed characterization suggested that T443 harbored a mutation in genes related to 11-hydroxylation (data not shown). Sequences flanking the EZ-Tn5™ transposon-insertion site in strain T443 were recovered by inverse PCR [Citation16], revealing that the transposon had inserted into ORF1195 of the BD3100 genome (data not shown).
A protein BLAST homology search suggested the presence of conserved domain as the Rieske [2Fe-2S] domain at N-terminal, and the Non-heme iron domain at C-terminal in the amino acid sequence of ORF1195. These characteristic domain sequences are conserved in Rieske non-heme iron oxygenase (RO), known as a multi-component oxygenase [Citation17,Citation18]. Thus, ORF1195 protein was predicted that is one subunit of a multi-component oxygenase belonging to the RO family. The enzyme activity of RO proteins typically requires ferredoxin (Fd) and ferredoxin reductase (FdR) co-factors as electron transport chain (ETC) components. However, the BD3100 genome contains multiple candidate ETC component-encoding genes along with multiple RO homologs. In contrast, the genome of strain CJ1, another BBR-utilizing bacteria known to use the 11-hydroxylation pathway for BBR metabolism, contained single candidate Fd- and FdR-encoding genes, an observation that was expected to simplify confirmation of the function of the corresponding proteins. Therefore, in the present report, we describe the identification and characterization of genes related to BBR 11-hydroxylation in strain CJ1.
Materials and methods
Chemicals and reagents
Berberine sulfate, palmatine chloride, and other chemicals were purchased from Tokyo Chemical Industry Co., Ltd. (Tokyo, Japan), Wako Pure Chemical Industries, Ltd. (Osaka, Japan), or LKT Laboratories (St. Paul, MN, USA). Berberrubine (BRU) and BBR derivatives were synthesized according to the previously reported methods, and 1H- and 13C NMR spectra of the synthesized compounds were compared with literature values [Citation19,Citation20]. The isolation methods and spectral data of the compounds are described in the Supplemental Information (Supplemental Methods, Fig. S7-S23).
Experimental instruments
1H- and 13C-NMR spectra were recorded using a Bruker AVANCE-400 spectrometer (400.13 MHz for 1H, 100.61 MHz for 13C; Bruker Biospin K. K., Kanagawa, Japan). Chemical shifts (δ) were measured in ppm using tetramethylsilane as an internal standard. BioShakers BR43-FH (TAITEC Co., Ltd., Saitama, Japan) and FTW-502 M (Hirayama Manufacturing Corp., Saitama, Japan) were used for incubation of bacteria. The turbidity of cultures was monitored using an OD Box-A (TAITEC Co., Ltd.) and an Ultrospec 2100 Pro ultraviolet (UV) – visible spectrophotometer (GE Healthcare UK Ltd., Little Chalfont, England) at 600 nm. HPLC analysis was performed using a PU-980 and PU-1580 pump (flow rate 0.5 mL/min; JASCO Co., Ltd., Tokyo, Japan) maintained at a temperature of 30 °C using a CO-2065 Plus column oven (JASCO Co., Ltd.) and equipped with a MD-2010 Plus photodiode array (JASCO Co., Ltd.). Molecular weights and molecular formulae were measured by LC-ESI-TOF-MS with a JMS-T100LP (JEOL, Ltd., Tokyo, Japan) and a 1200-series system (flow rate 0.2 mL/min, column temperature 30 °C; Agilent Technologies, Inc., Tokyo, Japan), and an Inertsil ODS-4 column (3 µm, 2.1 × 250 mm, GL Science, Inc.). The analysis of samples by HPLC and LC-ESI-TOF-MS was performed using a gradient system of the mobile phase as described in the conditions section below. Electroporation was performed using a Gene Pulser II (Bio-Rad, Hercules, USA), and conditions were as described below. DNA amplification was performed using a MyCycler (Bio-Rad). The concentrations of purified proteins were measured using a Qubit® 2.0 (Thermo Fisher Scientific, Yokohama, Japan).
PDA-HPLC analysis conditions
Eluent A was 0.1% Trifluoroacetic acid (TFA) in H2O, and Eluent B was acetonitrile. The eluent system was as follows. Initial conditions were 15% B from 0 to 3 min with a linear gradient to 85% B from 3 to 8 min; then this proportion was maintained until 10 min. The mobile phase was returned to initial conditions at 10.1 min, and maintained until the end of the run at 15 min.
LC-ESI-TOF-MS analysis conditions
Eluent A was 0.1% TFA in H2O, and Eluent B was acetonitrile. The eluent system was as follows. Initial conditions were 15% B with a linear gradient to 90% B from initial to 10 min; then this proportion was maintained until 12 min. The mobile phase was returned to initial conditions at 12.1 min and maintained until the end of the run at 18 min.
Bacterial strains, plasmids, primers, and culture conditions
Bacterial strains, plasmids, and primers used in this study are described in Supplementary Tables S2, S3, and S4. E. coli strains were grown aerobically at 37 °C in Luria-Bertani (LB) broth medium (containing 0.5 g of Bacto Yeast Extract, 1.0 g of Bacto Tryptone, and 0.5 g of sodium chloride in 100 mL of water) or on LB agar medium [consisting of LB supplemented with 1.5% (w/v) agar], unless otherwise noted. Strain CJ1 and its mutants were grown on 0.1 × LB agar medium [containing a 10-fold dilution of LB medium, 1 mM BBR, and 1.5% (w/v) agar] or (for liquid cultures) in LB medium, 0.5 × LB medium [containing a 2-fold dilution of LB medium], or WM medium [12.4 g Na2HPO4 · 12H2O, 1.7 g KH2PO4, 1.0 g (NH4)2SO4, 2.0 mg CaCO3, 1.44 mg ZnSO4 · 7H2O, 0.25 mg CuSO4 · 5H2O, 0.28 mg CoSO4 · 7H2O, 0.06 mg H3BO3, 100 mg MgSO4 · 7H2O, 1.44 mg FeSO4 · 7H2O, 13.45 mg MgO, and 16 µL HCl in 1 L of distilled water], unless otherwise indicated. All Burkholderia cultures were incubated at 30 °C, and liquid cultures were aerobically incubated with shaking at 180 rpm. Growth was monitored using an OD monitor (TAITECH Co., Ltd.) to measure the optical density at 600 nm (OD600).
Multiple alignments and phylogenetic tree construction
All sequence alignments were constructed using the ClustalW version 2.1. The Neighbor-joining tree was constructed using ClustalW2-Phylogeny (http://clustalw.ddbj.nig.ac.jp/top-j.html).
Functional annotation of BrhA
The brhA nucleotide sequence was submitted to a BLAST search of the genome sequence of CJ1 in the RAST server. Functional annotation of the predicted BrhA protein was accomplished by BLAST analysis of protein sequences in the Kyoto Encyclopedia of Genes and Genomes (KEGG) database, Swiss-Prot database, Non-Redundant protein database (NR), and Cluster of Orthologous Groups (COG) database using an E-value cutoff of 1E−5.
Preparation of cells for electroporation
CJ1 was inoculated in 10 mL LB medium in a test tube and incubated at 30 °C for 12 h. Also, E. coli. BL21(DE3) was incubated at 37 °C for 7 h. These cells were collected by centrifugation (18,800 × g, 5 min, 4 °C) and washed three times with 10% glycerol aqueous solution. The washed cells were suspended in 1 mL of 10% glycerol aqueous solution and divided into 100-µL aliquots. The cells then were frozen and stored at −80 °C pending use in electroporation.
Construction of deletion mutants of CJ1 by homologous recombination
CJ1 mutants CJ1Δ7323, CJ1Δ7339, CJ1Δ7340, CJ1Δ7331, and CJ1Δ7332 were generated using a double-crossover method employing pK18mobsacB, a plasmid that was obtained from the National BioResource Project (NIG, Japan). Total DNA from CJ1 was extracted from a cell pellet using a Genomic DNA Purification Kit (Promega KK, Tokyo, Japan) according to the manufacturer’s protocol. The DNA solution was used as the PCR template. PCR was performed using KOD FX Neo DNA polymerase (TOYOBO, Osaka, Japan) and the appropriate primers (Table S4). Amplified products were purified using a Wizard SV Gel and PCR Clean-Up System (Promega KK) according to the manufacturer’s protocol. The plasmids pKB7323, pKB7339, pKB7340, pKB7331, and pKB7332 were derived from pK18mobSacB; the cloning methods for construction of pK18mobsacB-system plasmids are described in the Supplemental Methods. After the plasmids were electroporated into CJ1, the transformants were subjected to stepwise selection, and the presence of the gene deletions was confirmed by PCR screening of the candidate mutants. Electroporation was performed using the 100-µL aliquots of frozen CJ1 cells prepared as described above. Specifically, the aliquots of CJ1 cells were thawed on ice; 1 µg of plasmid DNA (pKB7323, pKB7339, pKB7340, pKB7331, or pKB7332) was added to the cell suspension; the mixture was transferred to a chilled cuvette (1-mm gap); and the cells were electroporated (voltage: 1,200 V, capacitance: 25 µF, resistance: 800 Ω) using a Gene Pulser Xcell PC System (Bio-Rad Laboratories, Tokyo, Japan). Immediately after the electroporation, 1 mL of LB medium was dispensed into the cuvette; the cell suspension was transferred to a sterile tube; the suspension was incubated at 30 °C for 4 h; and the cells then were collected by the centrifugation (18,800 × g, 1 min, 4 °C). The resulting cell pellet was resuspended in a nominal volume of LB broth and spread on LB agar medium containing 50 µg/mL kanamycin (to select for cells harboring chromosome-integrated plasmids); the plate then was incubated at 30 °C for 2 days. To delete the targeted gene, each kanamycin-resistant strain was grown for 12 h in LB medium (i.e., without selection), and 10 µL of the resulting culture then was spread on LB agar medium containing 10% (w/v) sucrose (to provide counterselection against chromosomes harboring the integrated plasmid-borne sacB gene). The plate was incubated at 30 °C for 2 days, and the resulting colonies were screened on LB + 50 µg/mL kanamycin plates and LB + sucrose plates to confirm the sucrose-resistant, kanamycin-sensitive phenotype of the resulting strains. The deletion of the targeted genes in the selected strains was confirmed by PCR with the appropriate primers (Table S4).
Construction of complementation strain
Plasmid pJB861 was obtained from NIG; ORF7340 sequences were inserted by cloning (as described in the Supplemental Methods), yielding plasmid pJB7340. pJB7340 was electroporated into strain CJ1Δ7340 as described above. The resulting transformants were selected by growth on LB agar medium containing 50 µg/mL kanamycin, yielding complementation strain CJ1Δ7340/pJB7340. In parallel, we constructed the negative control strain CJ1Δ7340/pJB861 (i.e., harboring the parent plasmid without ORF7340) by the same techniques.
Preparation of resting cells
Bacteria were grown at 30 °C on 0.1 × LB agar medium plates containing 1.0 mM BBR. An individual colony was inoculated into 10 mL of LB medium. This seed culture was incubated for 20 h at 30 °C on a rotary shaker (180 rpm). One milliliter of this seed culture was inoculated into 100 mL of 0.5 × LB medium in a 500-mL culture flask. For cultures of cells harboring pJB861 or pJB7340, the medium was supplemented with 2.0 mM m-toluic acid (to induce expression from the plasmid-borne promoter) and 50 µg/mL kanamycin (for plasmid selection). The resulting culture was incubated as above (20 h at 30 °C with shaking). The cells then were harvested by the centrifugation (2,610 × g, 15 min, room temperature), and the collected pellets were washed twice with 50 mM phosphate buffer (pH 7.0) and then resuspended in phosphate-buffered saline (PBS; pH 7.0) for use in the resting-cell assay.
Resting-cell assay
The densities of the resting-cell suspensions were adjusted to OD600 values of 5 using 50 mM PBS. The individual suspensions then were supplemented with BBR (the degradation substrate) to a final concentration of 0.5 mM. The resulting suspensions (10 mL each) were incubated in conical flasks at 30 °C on a rotary shaker (180 rpm). At the indicated time points, 500-µL samples were collected and combined with an equal volume of methanol containing 0.05% (v/v) HCl (to quench the degradation reactions). Each sample then was centrifuged (18,800 × g, 5 min, 4 °C) to remove remaining cells and cell debris, and the resulting supernatants were analyzed by HPLC.
Expression of recombinant BrhA, BrhB, and BrhC in E. coli
The cloning methods for construction of pET system plasmids are described in the Supplemental Methods. Each of the resulting plasmids (pET7331, pET7332, and pET7340) encoded one of the Brh proteins with a C-terminal or N-terminal His-6 tag. The plasmids were transformed into E. coli BL21(DE3) by electroporation (voltage: 1800 V, capacitance: 25 µF, resistance: 200 Ω, 1-mm cuvette). In parallel, E. coli also was transformed with the empty parent vectors (pET-28a (+) or pET-21a(+)) to provide negative control strains. The pET7331- or pET-21a (+) -harboring E. coli transformant was incubated in 100 mL of LB medium containing 50 µg/mL ampicillin at 20 °C, with shaking at 150 rpm. The pET7332-, pET7340-, or pET-28a(+) -harboring E. coli transformants were incubated in 100 mL of LB medium containing 50 µg/mL kanamycin at 30 °C, 20 °C, and 20 °C respectively. Isopropyl-β-D-thiogalactopyranoside (IPTG) (0.1 mM) was added to the cultures when the OD600 reached 0.5. After 18 h of incubation, cells were collected by centrifugation (4 °C, 18,800 × g, 5 min) and washed three times with PBS. The washed cells were resuspended in PBS and ruptured using an ultrasonic cell disruptor. The resulting suspensions were centrifuged at 18,800 × g for 10 min to pellet the cell debris. The resulting supernatants represented crude enzyme extracts containing His-BrhA, BrhB-His, or His-BrhC, or (in the cases of the negative control strains harboring the empty vectors) containing no recombinant enzyme.
Purification of recombinant proteins
The recombinant proteins in the crude extracts were purified using HisPur™ Ni-TNA Resin (Thermo Fisher Scientific) according to the manufacturer’s protocol, and the samples in the elution buffer were exchanged into 50 mM Tris-HCl buffer using Microcon centrifugal filter devices (Millipore, Bedford, MA, USA). The expression and purity of each protein was confirmed by SDS-PAGE or Tricine-PAGE (Fig. S3), and the concentration of each purified protein was measured by the Qubit™ Protein Assay Kit and Qubit 2.0 (Thermo Fisher Scientific).
Purified-enzyme assay
The activity of the 11-hydroxylase toward BBR and BBR derivatives was measured by hydroxylation of 0.5-mM substrates. Each reaction solution consisted of 200 µL of 50 mM Tris-HCl buffer (pH 6.66) containing 30 µg oxygenase protein and/or 10 µg each of ferredoxin and reductase proteins, 1 mM Fe2+, and 0.5 mM NADH; incubations were performed at 30 °C for 60 min. The optimal pH was determined by incubating for 15 min under the conditions described above but at pHs ranging from 3.26 to 9.74. Three different buffering systems were used: 20 mM citric acid buffer (pH 3.26 to 6.33), 50 mM Tris-HCl buffer (pH 6.66 to 7.83), and 20 mM carbonic-bicarbonate buffer (pH 8.29 to 9.74). The optimal reaction temperature was determined by incubating for 15 min under the conditions described above (including pH 6.66) but at temperatures ranging from 5 to 65 °C in 5-°C intervals. The effects of possible metal ion cofactors on the enzyme were determined by addition of various divalent cations (each at 1.0 mM) or the metal-chelating agent EDTA (10 mM) to the reaction mixture, with incubation at 30 °C for 60 min. The relative activity was calculated by normalizing activities to that observed with no supplementary ions. The assays were initiated by addition of the substrate at a final concentration of 0.5 mM to the enzyme mixture; the reactions were performed at 30 °C for 60 min and then 80 µL of each reaction was combined with an equal volume of MeOH containing 0.05% (v/v) HCl (to quench the degradation reactions). Each resulting sample was centrifuged (18,800 × g, 5 min, 4 °C) to remove insoluble matter, and the resulting supernatants were analyzed by HPLC. Reaction products were identified by LC-ESI-TOF-MS as described above.
Results
The BBR-11 hydroxylase-encoding gene “brhA” in Burkholderia sp. strain CJ1
The genome of strain CJ1 was BLAST searched using the predicted amino acid sequence of ORF1195 from strain BD3100. This search retrieved 3 genes encoding proteins with 67% (ORF7323), 53% (ORF7340), and 46% (ORF7339) sequence identity to the probe. Each of the 3 predicted proteins contained a Rieske non-heme iron motif, including matches to an N-terminal iron-sulfur protein domain (Cys-X-His; CXH and Cys-X-X-His; CX2H Box) with a conserved Rieske [2Fe-2S] center, and to a C-terminal catalytic domain (Asp-X-X-X-Asp-X-X-His-X-X-X-X-His; DX3DX2HX4H Box) with a conserved mononuclear iron-binding site (Fig. S1). We examined the role of these three genes (ORF7323, ORF7339, andORF7340) by generating strain harboring mutations in each of the loci (CJ1Δ7323, CJ1Δ7339, and CJ1Δ7340 respectively). When cultured on 0.1 × LB agar medium plates containing BBR, CJ1Δ7323 and CJ1Δ7339 exhibited as much as decolorization (i.e., BBR degradation) as the wild-type (WT) parent, CJ1 (Fig. S2). On the other hand, CJ1Δ7340 did not decolorize the agar at all ()). This result suggested the involvement of ORF7340 in BBR metabolism; we therefore tentatively designated ORF7340 as “brhA” (“BBR hydroxylase-encoding gene A”). BrhA belongs to the Rieske oxidase (RO) family of proteins, a class that includes dicamba O-demethylase (DdmC) from Strenotrophomonas maltophilia DI-6 (Accession No. AY786443) [Citation21] and chloroacetanilide herbicides N-dealkylase; (CndA) from Sphingomonads DC-6 and DC-2 (Accession No. KJ461679) [Citation22]. Notably, DdmC and CndA exhibit protein sequence identities of 33% to 38% with the predicted BrhA protein, and all three proteins share the conserved Rieske domains ()).
Figure 2. The growth of, and berberine degradation by, strain CJ1 and its mutants. (a) Growth of CJ1 (Wile-type; WT) and the mutants Δ7331, Δ7332 and Δ7340 after 5 days on 0.1 × LB (Luria-bertani) medium containing 1 mM BBR. (b, c) The growth (as assessed by optical density) of CJ1 and mutants in (b) LB medium and (c) minimal medium containing 1 mM berberine (BBR). Symbols: CJ1 (white circles), Δ7331 (black squares), Δ7332 (black triangles), and Δ7340 (black circles).
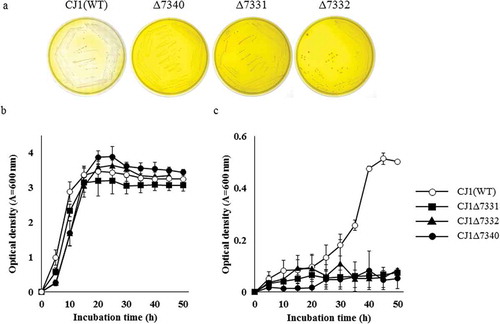
Figure 3. Alignment of the predicted amino acid sequence of BrhA, BrhB, and BrhC with the sequences of paralogs. Alignment of BrhA with DdmC and CndA shows the conserved domain (shadow box) in this class. Proteins in this family contain a Rieske domain with the consensus sequence Cys-X-His (CXH) and Cys-X-X-His (CX2H) and a non-heme iron domain with the consensus sequence Asp-X-X-X-Asp-X-X-His-X-X-X-X-His (DX3DX2HX4H). BrhA (LC500707), oxygenase component of BBR 11-hydroxylase from Burkholderia sp. CJ1 (BurkCJ1); CndA (KJ461679), oxygenase component of N-dealkylation of chloroacetanilide herbicides from Sphingomonads DC-6 (SphiDC6); DdmC (AY786443), oxygenase component of dicamba O-demethylase from Stenotrophomonas maltophilia strain DI-6 (SmalDI6). (b) Alignment of BrhB with Glutathione type (GR-type) reductases shows the conserved domain (shadow box) in this class. Proteins in this family have two ADP-binding domains (for FAD and NADH, respectively) with the consensus sequence Gly-X-Gly-X-X-Gly-X-X-X-Ala (GXGX2GX3A) and a flavin-binding domain (for FAD) with the consensus sequence Thr-X-X-X-X-X-X-Ala-X-Gly-Asp (TX6AXGD). BrhB (LC500708), reductase component of BBR 11-hydroxylase from BurkCJ1; DdmA1 (AY786444) and DdmA2 (AY786445), reductase component of DdmC from SmalDI-6; CndC1 (AHW42445), reductase component of CndA from SphiDC6. (c) Alignment of BrhC with plant-type ferredoxins shows the conserved domain (shadow box) in this class. Proteins in this family contain a [2Fe-2S] domain with the consensus sequence Cys-X-X-X-X-X-Cys-X-X-Cys-(inserted 36 or 37 of any amino acid; X)-Cys (CX5CX2CX36/37C). BrhC, ferredoxin component of BrhC (LC500709) from BurkCJ1; CndB1 (AHW42448) and CndB2 (AHW42449); ferredoxin components of from SphiDC-6; DdmB (AAV53698), ferredoxin component of DdmC from SmalDI-6 (GenBank accession numbers in parentheses).
![Figure 3. Alignment of the predicted amino acid sequence of BrhA, BrhB, and BrhC with the sequences of paralogs. Alignment of BrhA with DdmC and CndA shows the conserved domain (shadow box) in this class. Proteins in this family contain a Rieske domain with the consensus sequence Cys-X-His (CXH) and Cys-X-X-His (CX2H) and a non-heme iron domain with the consensus sequence Asp-X-X-X-Asp-X-X-His-X-X-X-X-His (DX3DX2HX4H). BrhA (LC500707), oxygenase component of BBR 11-hydroxylase from Burkholderia sp. CJ1 (BurkCJ1); CndA (KJ461679), oxygenase component of N-dealkylation of chloroacetanilide herbicides from Sphingomonads DC-6 (SphiDC6); DdmC (AY786443), oxygenase component of dicamba O-demethylase from Stenotrophomonas maltophilia strain DI-6 (SmalDI6). (b) Alignment of BrhB with Glutathione type (GR-type) reductases shows the conserved domain (shadow box) in this class. Proteins in this family have two ADP-binding domains (for FAD and NADH, respectively) with the consensus sequence Gly-X-Gly-X-X-Gly-X-X-X-Ala (GXGX2GX3A) and a flavin-binding domain (for FAD) with the consensus sequence Thr-X-X-X-X-X-X-Ala-X-Gly-Asp (TX6AXGD). BrhB (LC500708), reductase component of BBR 11-hydroxylase from BurkCJ1; DdmA1 (AY786444) and DdmA2 (AY786445), reductase component of DdmC from SmalDI-6; CndC1 (AHW42445), reductase component of CndA from SphiDC6. (c) Alignment of BrhC with plant-type ferredoxins shows the conserved domain (shadow box) in this class. Proteins in this family contain a [2Fe-2S] domain with the consensus sequence Cys-X-X-X-X-X-Cys-X-X-Cys-(inserted 36 or 37 of any amino acid; X)-Cys (CX5CX2CX36/37C). BrhC, ferredoxin component of BrhC (LC500709) from BurkCJ1; CndB1 (AHW42448) and CndB2 (AHW42449); ferredoxin components of from SphiDC-6; DdmB (AAV53698), ferredoxin component of DdmC from SmalDI-6 (GenBank accession numbers in parentheses).](/cms/asset/46cb831c-fe56-41ca-b444-8dd82b469c38/tbbb_a_1722056_f0003_b.gif)
Electron transport chain (ETC) components for BrhA
To identify ETC components that may function with BrhA, we sought homologs of ETC components of known enzymes. Notably, DdmC and CndA, which show sequence similarity with BrhA, are known to (separately) form three-component ROs containing ferredoxin (Fd) and ferredoxin reductase (FdR) as ETC proteins, and each component has been identified [Citation21,Citation22]. A previous report showed that DdmC employs DdmB (Accession No. AY786442) as a Fd, and DdmA1 (Accession No. AY786444) and DdmA2 (Accession No. AY786445) as FdRs. Similarly, CndA has been reported to employ CndB1 (Accession No. KJ020542) and CndB2 (Accession No. KJ020543) as Fds, and CndC1 (Accession No. KJ020540) as a FdR. The protein sequences of the respective ETC components were used to search the predicted proteome of strain CJ1, revealing that the CJ1-encoded proteins ORF7331 and ORF7332 show the best domain matches (contained single candidate Fd- and FdR-encoding genes) with the previously characterized ETC components (–c)). Specifically, the CJ1 ORF7332 protein showed high similarity (59–63% amino acid identity) with DdmB, CndB1, and CndB2, while the CJ1 ORF7331 protein showed high similarity (61–70% amino acid identity) with DdmA1, DdmA2, and CndC1. These results suggested that ORF7332 is a Fd-coding gene, and that ORF7331 is a FdR-coding gene. We investigated these genes for potential involvement in BBR metabolism by generating CJ1Δ7331 and CJ1Δ7332 mutant strains. Notably, neither single mutant strain decolorized solid medium when streaked to 0.1 × LB agar medium plates containing BBR, suggesting the involvement of ORF7331 and ORF7332 in BBR metabolism ()). Therefore, we redesignated the ORF7331 and ORF7332 genes “brhB” and ‘brhC, respectively Our mutant analyzes indicated that brhA, brhB, and brhC are involved in BBR metabolism, but we wanted to confirm that are these mutant phenotypes did not reflect more general effects on the growth of CJ1. To address this issue, wild-type and mutant CJ1 isolates were cultured in LB broth and growth was tracked over time via OD600. Each strain achieved full growth by 20 h, and growth rates did not appear to differ appreciably ()). On the other hand, when the same strains were cultured on W (minimal) medium containing BBR as the sole carbon source, wild type achieved full growth by 40 h, while the mutants strains exhibited only limited growth ()). These results suggested that the brhA, brhB, and brhC gene are not necessary for the growth of CJ1 under standard laboratory conditions but are essential for the BBR-utilization of CJ1.
Resting-cell assays of CJ1 mutants
BBR metabolism of CJ1Δ7331, CJ1Δ7332 and CJ1Δ7340 were investigated by measuring BBR degradation using resting-cell assays. Under our experimental conditions, the resting cells of wild-type CJ1 completely degraded BBR within 5 h ()). The depletion of BBR and the accumulation of D-BBR, H-BBR, and 11-hydroxydemethyleneberberine (HD-BBR) was demonstrated by PDA-HPLC chromatography of reactions with wild-type CJ1 cells ()). However, HPLC analysis of the mutant strains did not detect BBR metabolism nor the production of BBR hydroxylates through 5 h of reaction (). These results suggested that brhA, brhB, and brhC are necessary for BBR hydroxylation by the CJ1 strain.
Figure 4. Investigation of brhA, brhB, and brhC involvement in berberine 11-hydroxylation using a resting-cell assay. The resting-cell assay of CJ1 (wild type; WT) and the mutants CJ1Δ7331, CJ1Δ7332, and CJ1Δ7340. The curves indicate the results obtained with (a) CJ1, (b) CJ1Δ7331, (c) CJ1Δ7332, and (d) CJ1Δ7340. Each curve shows the remaining concentration of BBR (100% with 0.5 mM berberine added) indicated by black circles and the peak areas of D-BBR (black squares), H-BBR (white circles), and HD-BBR (white squares) during the resting-cell assay. BBR, berberine; D-BBR, demethyleneberberine; H-BBR, 11-hydroxyberberine; HD-BBR, 11-hydroxydemethyleneberberine. (e) HPLC chromatograms (280 nm) of resting-cell assay with BBR. CJ1(WT) degrades BBR to H-BBR and HD-BBR after 3 h in the assay.
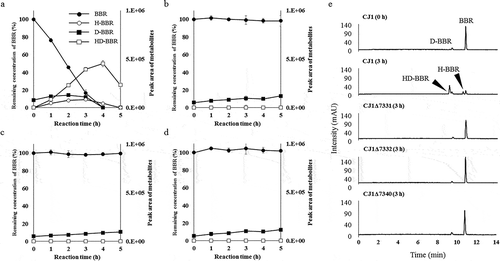
A brhA complementation experiment
A brhA complementation experiment was performed by constructing a CJ1Δ7340/pJB7340 strain, in which a plasmid carrying brhA was introduced into the Δ7340 mutant. A control strain (CJ1Δ7340/pJB861) was generated by introducing the empty vector (pJB861) into the same background. Notably, decolorization was observed around colonies of the complemented strain (CJ1Δ7340/pJB7340), but not around colonies of the control strain, when grown for 5 days on 0.1 × LB agar medium containing BBR, ()). In addition, resting-cell assays were performed with the same pair of strains in the presence of 0.5 mM BBR [in the PBS buffer (pH 7.0) at 30 °C]. HPLC monitoring of BBR and BBR metabolites after 2 h of reaction demonstrated that CJ1Δ7340/pJB7340 completely degraded BBR while producing the BBR hydroxylates H-BBR and HD-BBR (–c)). These effects were not seen with the control strain, indicating that the loss of BBR hydroxylation activity reflects the brhA gene defect.
Figure 5. Examination of BBR 11-hydroxylation ability of brhA by complementation experiment. (a) Growth of control strain (CJ1Δ7340/pJB861) and complemented strain (CJ1Δ7340/pJB7340) after 5 days on 0.1 × LB medium containing 1 mM berberine (BBR). (b) Each curves shows the remaining concentration of BBR (100% with 0.5 mM BBR added) and the peak areas of D-BBR, H-BBR, and HD-BBR. Curves indicating the results obtained with the control (BBR: black circles, D-BBR: black squares, H-BBR: black diamonds, HD-BBR: black triangles) and complemented strains (BBR: white circles, D-BBR: white squares, H-BBR: white diamonds, HD-BBR: white triangles) during the resting-cell assays. D-BBR, demethyleneberberine; H-BBR, 11-hydroxyberberine; HD-BBR, 11-hydroxydemethyleneberberine. (c) HPLC chromatograms (280 nm) of resting-cell assay with BBR. Complemented strain degraded BBR to H-BBR and HD-BBR after 1 h in the assay.
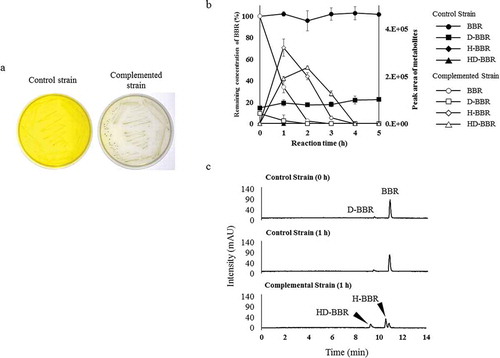
In vitro reconsititution of the BrhA, BrhB, and BrhC combined enzyme
The optimum reaction pH and temperature of the combined enzyme reaction was pH 6.6, and 25 °C to 40 °C (Fig. S4). Investigation of the role of divalent metal ions demonstrated that enzyme activity was enhanced by Fe2+ but significantly inhibited by the metal-chelating agent EDTA (Table S5). These data suggested that metal ions are required for the combined enzyme reaction. No significant difference in BBR hydroxylation activity was observed when comparing between NADH- and NADPH-containing reactions in the presence of all 3 enzyme (BrhA, BrhB, and BrhC) (Table S6). The production of H-BBR was observed only in the presence of the 3-enzyme mixture supplemented with both NADH and Fe2+ (,b)).
Figure 6. Purified-enzyme assays under various conditions. (a) Curves indicating the results of a purified-enzyme assay including all 3 enzymes in the presence of NADH and Fe2+. Each curve shows the remaining concentration of BBR (100% with 0.5 mM BBR added) and the peak area of H-BBR. Curves indicating the control (BBR: black circles, H-BBR: black squares) and enzyme added (BBR: white circles, H-BBR: white squares). BBR, berberine; H-BBR, 11-hydroxyberberine. (b) HPLC chromatograms (280 nm) of the reactions at 60 min in the presence of various combinations of purified enzymes; all reactions were performed in the presence of 0.5 mM NADH and 1 mM Fe2+. The BBR standard is shown at the bottom. The production of H-BBR was observed only with the combination of BrhA, BrhB, and BrhC in the presence of NADH and Fe2+.
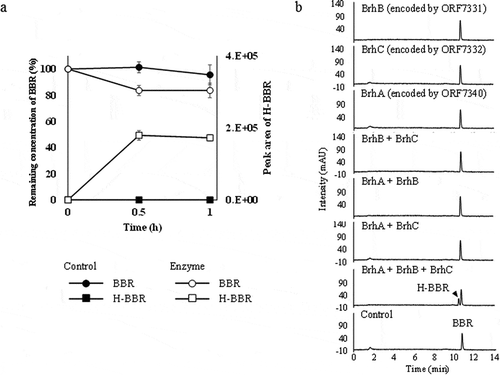
Substrate specificity of BrhA
The substrate specificities of BrhA were examined by hydroxylation activity against BBR derivatives including PAL (1), 9-O-ethyl BBR (3), 9-O-propyl BBR (4), and 9-O-isopropyl BBR (5). Compounds 3, 4, and 5 were synthesized by O-alkylation at BBR methoxy group position 9 [Citation19,Citation20]. Activities were normalized against that for BBR (2) hydroxylation. The results revealed that BrhA exhibited activity against PAL (1) that was twice that against BBR (2); activity against Compounds 3, 4, and 5 were 2-fold or more less than that against BBR (2) (Fig. S5, 7).
Figure 7. Examination of substrate specificity of recombinant berberine 11-hydroxylase protein. The bar numbers in the graph indicate the compound numbers of the tested substrates: 1, palmatine (PAL); 2, berberine (BBR); 3, 9-O-ethyl berberine (9-O-ethyl BBR); 4, 9-O-propyl berberine (9-O-propyl BBR); and 5, 9-O-isopropyl berberine (9-O-isopropyl BBR). The relative activity was calculated by normalizing 11-hydroxyberberine (H-BBR) production at 60 min against that obtained with BBR (2).
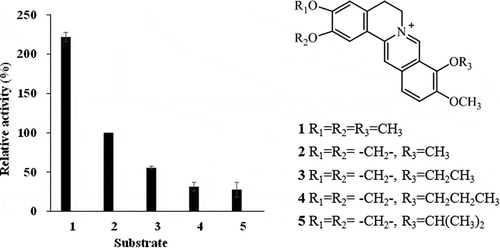
Discussion
The BBR 11-hydroxylase-encoding gene of Burkholderia sp. strain CJ1 was identified as ORF7340; this gene therefore was redesignated brhA. The predicted BrhA belongs to the RO family, members of which require ETC components Fd and FdR for enzyme activity. The genes encoding the corresponding Fd and FdR proteins in strain CJ1 were identified as ORF7332 and ORF7331, which therefore were redesignated brhC and brhB, respectively. In vitro reconstitution of the multi-component enzyme reaction indicated that BrhA, BrhB, and BrhC require NAD(P)H and Fe2+ as co-factors, as shown schematically in , yielding an activity sufficient for 11-hydroxylation of BBR.
Figure 8. Components and proposed functions of the berberine 11-hydroxylase of Burkholderia sp. strain CJ1. The inferred electron transfer reactions and the conversion of berberine (BBR) to 11-hydroxyberberine (H-BBR) are indicated.
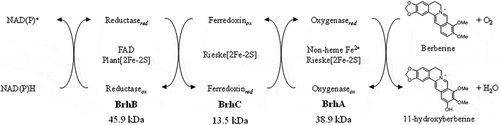
Kweon et al. [Citation23] and Chakraborty et al. [Citation24] proposed a RO classification method based on the interaction between oxygenase subunit structures and ETC component types and combinations; according to that system, the RO enzymes are assigned to 11 classes designated Iα to VIIα. These classifications are defined first by the presence or absence of a β subunit associated with the oxygenase α subunit composed of the active site [2Fe-2S]RSK[Fe+2]. The RO proteins then are divided into the two types based on the ETC components, one employing both Fd and FdR, and the other employing only FdR. Further classification is made based on variations in the Fd and FdR components. BrhA is composed only of an α subunit, and need both Fd and FdR. And more, BrhB is a Plant type Fd, and BrhC is a Glutathione reductase type FdR. According to the Kweon and Chakraborty’s classifications, we infer that BrhA, BrhB, and BrhC are class-VIIα enzymes (Fig. S6).
BrhA has novel hydroxylation activity for BBR and derivatives that possess a protoberberine skeleton. The known ROs have been shown to exhibit a variety of catalytic activities such as aromatic and olefin cis-dihydroxylation [Citation25], benzylic hydroxylation [Citation26], methyl group hydroxylation [Citation27], oxygen-dependent desaturation [Citation28], sulfosidation, O-dealkylation [Citation29], and N-dealkylation [Citation22], but previously have not been reported (to our knowledge) to exhibit oxygenase activity capable of catalyzing polycyclic aromatic-ring alkaloids. Therefore, the present work represents the first report of a bacterial multi-component oxygenase capable of catalyzing the direct hydroxylation of substrates with a protoberberine skeleton. In previous work, we demonstrated the existence of two distinct BBR degradation pathways, demethylenation and 11-hydroxylation pathway, in BBR-utilizing bacteria [Citation13,Citation14]. In the present study, we identified BrhA, BrhB, and BrhC as components of a BBR 11-hydroxylation enzyme encoded by strain CJ1 and characterized the corresponding activity following in vitro reconstitution of the enzyme. These results clarify the activities employed by BBR-utilizing bacteria and are expected to be useful for elucidating the BBR-degradation pathways.
Author contribution
HT, HY, TH conceived this work and designed the experiments. HY performed the experiments and analyzed the data together with DW, HT, and TH, and FS provided valuable advice. DW helped acquire specialized experimental data. HY wrote the manuscript. All authors contributed to and approved the final version.
Supplemental_information__20200117_.pdf
Download PDF (1.4 MB)Acknowledgments
We thank Nobuo Kawahara, Ph.D., director of the Research Center for Medicinal Plant Resources of the National Institutes of Biomedical Innovation, Health, and Nutrition, for providing soil samples. We also thank Hisashi Takeda, Ph.D., for the kind gift of the BD3100 transposon mutant library.
Disclosure statement
No potential conflict of interest was reported by the authors.
Supplementary material
Supplemental data for this article can be accessed here.
References
- Ikuta A, Itokawa H. Alkaloids of Coptis Rhizome. J Nat Prod. 1984;47(1):189–190.
- Tosa S, Ishihara S, Nose Y, et al. Studies on quality control of crude drugs (I) analysis and distribution of alkaloids in Phellodendron amurense RUPRECHT. Shoyakugaku Zasshi (in Japanese). 1989;43:28–34.
- Amin AH, Subbaiah TV, Abbasi KM. Berberine sulfate: antimicrobial activity, bioassay and mode of action. Can J Microbiol. 1969;15:1067–1076.
- Sun D, Abraham SN, Beachey EH. Influence of berberine sulfate on synthesis and expression of pap fimbrial adhesion in uropathogenic Escherichia coli. Antimicrob Agents Chemother. 1988;32(8):1274–1277.
- Vennerstrom JL, Klayman DL. Protoberberine alkaloids as antimalarials. J Med Chem. 1988;31:1084–1087.
- Vollekova A, Kostalova D, Sochorova R. Isoquinoline alkaloids from Mhonia aquifolium stem bark are active against Malassezia spp. Folia Microbiol (Praha). 2001;46:107–111.
- Kim S, Lee J, You D. et al. Berberine suppresses cell motility through downregulation of TGF-b1 in triple negative breast cancer cells. Physiol Biochem. 2018;45(2):795–807.
- Agnarelli A, Natali M, Garcia-Gil M. et al. Cell-specific pattern of berberine pleiotropic effects on different human cell lines. Sci Rep. 2018;8(1):1–15.
- Jung HA, Yoon NY, Bae HJ, et al. Inhibitory activities of the alkaloids from Coptidis Rhizoma against aldose reductase. Arch Pharm Res. 2008;31:1405–1412.
- Shi R, Xu Z, Xu X, et al. Organic cation transporter and multidrug and toxin extrusion 1 co-mediated interaction between metformin and berberine. Eur J Pharm Sci. 2019;127:282–290.
- Chow YL, Sogame M, Sato F. 13-Methylberberine, a berberine analogue with stronger anti-adipogenic effects on mouse 3T3-L1 cells. Sci Rep. 2016;6:38129.
- Han Y, Kim MJ, Lee KY. Berberine derivative, Q8, stimulates osteogenic differentiation. Biochem Biophys Res Commun. 2018;504:340–345.
- Ishikawa K, Takeda H, Wakana D, et al. Isolation and identification of berberine and berberrubine metabolites by berberine-utilizing bacterium Rhodococcus sp. Strain BD7100. Biosci Biotechnol Biochem. 2016;80:856–862.
- Takeda H, Ishikawa K, Wakana D, et al. 11-hydroxylation of protoberberine by the novel berberine-utilizing aerebic bacterium sphingobium sp. strain BD3100. J Nat Prod. 2015;78:2880–2886.
- Takeda H, Ishikawa K, Yoshida H, et al. Common origin of methylenedioxy ring degradation and demethylation in bacteria. Sci Rep. 2017;7(1):1–8.
- Ochman H, Gerber AS, Hartl DL. Genetic applications of an inverse polymerase chain reaction. Genetics. 1988;120:621–623.
- Brunel F, Davison J. Cloning and Sequencing of Pseudomonas genes encoding vanillate demethylase. J Bacteriol. 1988;170(10):4924–4930.
- Priefert H, Rabenhorst J, Steinbüchel A. molecular characterization of genes of Pseudomonas sp. Strain HR199 involved in bioconversion of vanillin to protocatechuate. J Bacteriol. 1997;179(8):2595–2607.
- Kim JH, Lee SJ, Kim SH. New derivatives of berberrubine and their salts for antibiotics and antifungal agents. PCT. WO2003051875A1. 2003.
- Li YH, Li Y, Yang P, et al. Design, synthesis, and cholesterol-lowering efficacy for prodrugs of berberrubine. Bioorg Med Chem. 2010;18:6422–6428.
- Herman PL, Behrens M, Chakraborty S, et al. A three-component dicamba O-demethylase from Pseudomonas maltophilia, Strain DI-6. J Biol Chem. 2005;280(26):24759–24767.
- Chen Q, Wang CH, Deng SK, et al. A novel three-component rieske non-heme iron oxygenase (ROH) system catalyzing the N-dealkylation of chroloacetanilide herbicides in Sphingomonads DC-6 and DC-2. Appl Environ Microbial. 2014;80(16):5078–5085.
- Kweon O, Kim SJ, Baek S, et al. A new classification system for bacterial Rieske non-heme iron aromatic ring-hydroxylating oxygenases. BMC Biochem. 2008;9(1):11.
- Chakraborty J, Ghosal D, Dutta A, et al. An insight into the origin and functional evolution of bacterial aromatic ring-hydroxylating oxygenases. J Biomol Struct Dyn. 2012;30(4):419–436.
- Axcell BC, Geary PJ. Purification and some properties of a soluble benzene-oxidizing system from a strain of Pseudomonas. Biochem J. 1975;146:173–183.
- Rosche B, Tshisuaka B, Fetzner S, et al. 2-Oxo-1, 2-dihydroquinoline 8-monooxygenase, a two-component enzyme system from Pseudomonas putida 86. J Biol Chem. 1995;270(30):17836–17842.
- Schäfer F, Schuster J, Würz B, et al. Synthesis of short-chain diols and unsaturated alcohols from secondary alcoholic substrates by the Rieske non-heme mononuclear iron oxygenase MdpJ. Appl Environ Microbiol. 2012;78(17):6280–6284.
- Wollam J, Magomedva L, Magner DB, et al. The rieske oxygenase DAF-36 functions as a cholesterol 7-desaturase in steroidogenic pathways governing longevity. Aging Cell. 2011;10:879–884.
- Gallagher JR, Olson ES, Stanley DC. Microbial desulfurization of dibenzothiophene: A sulfur-specific pathway. FEMS Microbiol Lett. 1993;107:31–36.