ABSTRACT
The oleaginous yeast Rhodosporodium toruloides is receiving widespread attention as an alternative energy source for biofuels due to its unicellular nature, high growth rate and because it can be fermented on a large-scale. In this study, R. toruloides was cultured under both light and dark conditions in order to understand the light response involved in lipid and carotenoid biosynthesis. Our results from phenotype and gene expression analysis showed that R. toruloides responded to light by producing darker pigmentation with an associated increase in carotenoid production. Whilst there was no observable difference in lipid production, slight changes in the fatty acid composition were recorded. Furthermore, a two-step response was found in three genes (GGPSI, CAR1, and CAR2) under light conditions and the expression of the gene encoding the photoreceptor CRY1 was similarly affected.
GRAPHICAL ABSTRACT
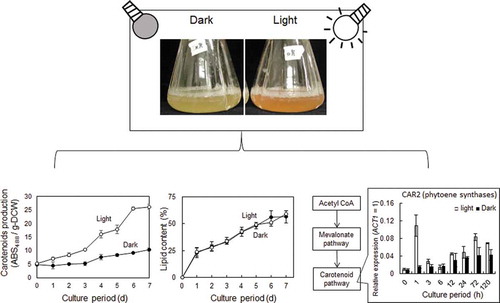
Light effect on carotenoid production in Rhodosporidium toruloides
The world’s population is currently estimated at 7.6 billion people and is predicted to reach 9.8 billion by 2050, according to a United Nations report dated June 21st, 2017 [Citation1]. Such an increase in population is predicted to nearly triple the current worldwide demand for energy and to disrupt the global food balance thus threatening our global food security. In recent years, there has been much attention and financial investment in renewable energy resources to deliver future energy demands in a sustainable and environmentally friendly way. However, more attention needs to be paid to microorganisms that can produce alternative energy sources or valuable material. Rhodosporidium toruloides is a nonpathogenic, red basidiomycetous fungus, which can accumulate large of lipids in its cell from various monosaccharides [Citation2]. It can also produce carotenoids and biotechnologically important enzymes [Citation3,Citation4]. Furthermore, because the lipids produced by R. toruloides have a similar fatty acid composition to vegetable oil, it is possible to use them to produce an edible oil [Citation5]. Thus, microbial lipid production by R. toruloides offers a promising source of alternative oil for both the biodiesel and food industries. As in the R. glutinis ATCC 26085 and Sporobolomyces roseus B99040, total fatty acid was reported 19.7 and 14.3 µg/g dry weight respectively. In which, C16:1, C16:0, C18:2 and C18:0 fatty acid as the main fatty acid were 2.08%, 15.52%, 72.1%, 5.72% and 1.75%, 13.38%, 60.2%, 16.58% respectively with the former was R. glutinis ATCC 26085 and the latter was S. roseus B99040 [Citation6].
Table 1. Carotenoid composition of R. toruloides cell grown under light or dark conditions.
Table 2. Fatty acid composition of R. toruloides cell grown under light or dark conditions.
R. toruloides belongs to the red yeast category known for its ability to produce carotenoids. Carotenoids are lipid-soluble hydrocarbons, usually comprised of 40 carbon atoms. They are widely distributed in nature and over 600 types have been identified. They are divided into two classes; one which contain oxygen (xanthophylls) and another which do not (carotenes) [Citation7]. Xanthophylls are synthesized in a wide variety of photosynthetic and nonphotosynthetic organisms including plants and microorganisms. Carotenoids have many uses. As pigments, they are utilized by the food industry as a natural red and yellow colorant. Some carotenoids are precursors of vitamin A (β-carotene) or antioxidant agents (for example torulene, astaxanthin), both of which are used as vitamins and dietary supplements [Citation8]. In addition, they are among the bioactive phytochemicals that are associated with reducing the risk of developing degenerative diseases such as cancers, cardiovascular diseases, macular degeneration, and cataracts. Hence, the potential to produce carotenoids using oleaginous yeasts makes R. toruloides an important organism. Among of carotenoids, the principle of carotenoids synthesized by R. toruloides are β-carotene, γ-carotene, torulene and torularhodin [Citation9–Citation12]. The R. glitinis DBVPG 6081 had 112.2 µg/g cell dry mass of carotenoids that distributed in 46.8% of torularhodin, 30.57% of torulene, 4.55% of γ-carotene and 18% of β-carotene. High and main carotenoids also reported in the R. toruloides DBVDG 6739 strain with 122.6 µg/g cell dry mass of carotenoids (16.23%, 42.17%, 7.34% and 18.1% of torularhodin, torulene, γ-carotene and β-carotene, respectively) [Citation9]. In other strain, R. glutinis mutant 32, 5.4 mg/g dry cell was carotenoids; in which, torularhodin, torulene and β-carotenoid were 2.3%, 17% and 80%, respectively [Citation13]
Carotenoid production by microbes offers several advantages over other methods, including the absence of seasonal and geographical variation and the potential to achieve high rates of production using low-cost natural substrates (such as carbohydrates) [Citation14,Citation15]. However, it is influenced by many factors including choice of carbon source, light, temperature, pH, aeration, metal ion and salts [Citation16,Citation17]. Light is a particularly important factor because carotenogenesis is a photoprotective mechanism; microorganisms produce carotenoids as an antioxidant which perform a role in protecting cells from light or other environmental stresses [Citation16]. Thus, the potential carotenoid yield is dependent on the period and intensity of light exposure. Two important aspects of photo-induction have previously reported: the first is that conditions that increase microbial growth also increase carotenoid production, the second is that increased activity of the enzymes essential to carotenoid biosynthesis results in an associated increase in cellular accumulation of carotenoids [Citation8]. There is evidence that fungal growth and carotenoid production is influenced by exposure to light [Citation18,Citation19]. In Rhodotorula glutilis, increased biomass concentration and carotenoid formation have been observed in light-grown cultures [Citation12] and in R. toruloides carotenogenesis has been shown to be light inducible [Citation20].
Braunwald et al. reported that in R. glutilis both carotenoids and lipids accumulated in cultures that contained a high carbon to nitrogen ratio and that carotenoids were produced under conditions that also promoted lipid synthesis [Citation21]. Thus, it is important to understand the bioregulation of both carotenoids and lipids in order to determine the optimal conditions for carotenoid production. When glucose enters glycolysis, it is converted into pyruvate. In the oleaginous yeast, the conversion of pyruvate to acetyl CoA for the TCA cycles delivers citrate to the next step. Acetyl CoA molecule is a precursor for the fatty acid biosynthesis pathway or the mevalonate biosynthesis pathway that continues to the carotenoid biosynthesis pathway (). There may be a relationship between the carotenoid and lipid biosynthesis pathways.
In this study, we investigated the effects of light on lipid and carotenoid production of R. toruloides NBRC 10032. NBRC 10032 was identified as the strong lipid producer screened from the National Institute of Technology and Evaluation strains. Carotenoids are localized in the lipid body in oleaginous yeast. Thus, NBRC 10032 can be considered a suitable strain for carotenoid production. By comparing microbial growth and gene expression patterns, we demonstrate that carotenoid biosynthesis is controlled at the transcription level in response to light.
Materials and methods
Yeast strain and culture condition
The R. toruloides NBRC 10032 strain was obtained from the National Institute of Technology and Evaluation (NITE, Chiba, Japan). R. toruloides was grown on Yeast Peptone D-Glucose (YPD) agar plates (1% Yeast extract, 1% Peptone, 2% D-Glucose, 2% Agar) at 30°C for four days and then maintained at 4°C. Plates were sub-cultured every month. YPD medium was used for pre-cultivation and LS10 medium (0.805% Powder Yeast extract, Kyokuto Seiyaku Co., Tokyo, Japan, 15% D-Glucose, 0.047% NH2CONH2, 0.15% MgSO4.7H2O, 0.04% KH2PO4, 1.9 × 10−6 mmol/L ZnSO4, 1.22 × 10−4 mmol//L MnCl2, 1 × 10−4 mmol/L CuSO4 and 1.5 mmol/L CaCl2, Nacalai Tesque, Tokyo, Japan) was used for lipid accumulation. Yeast cells were pre-cultivated for 24 h in YPD medium in L-shaped test tubes and then cultured in 200 mL Erlenmeyer flasks in 50 mL LS10 medium, at an initial optical density (O.D.) of 660 nm = 0.1. The experiment was performed in a dark room. Dark conditions were achieved by wrapping samples in aluminum foil (two layers, 24 µm thick in total) and light conditions by using a 27-Watt fluorescent lamp (98 µmol/m2/s photon at the surface of the medium).
Samples used for the analysis of gene expression during the earlier cultivation stages were produced from cells cultured in LS10 medium (15% glucose) in the dark for 24 h, after which the cultures were transferred to either light or dark conditions and sampled at appropriate times post induction.
Biochemical analysis
Yeast growth rate was measured by ultraviolet-visible spectroscopy (UV–Vis) UV mini-1240 (Shimadzu, Kyoto, Japan) and the O.D. of each culture was recorded at a wavelength of 660 nm. Dry cell weight (DCW) was quantified in lyophilized cells derived from 1 mL of broth. The glucose concentration of the culture supernatant was determined using the Glucose CII Test Wako kit (Wako Pure Chemical Industries Ltd., Osaka, Japan) to provide a measure of glucose consumption over the growth period.
Lipids were extracted from cells in a solvent mixture of petroleum ether and ethanol (1:3, v/v) using a Multi Beads Shocker (Yasui Kikai, Osaka, Japan). Dry cells suspended in 200 µL of the solvent mixture with 500 mg of glass beads (0.5 mm diameter) were disrupted in the Multi Beads Shocker (conditions: 4°C, 2500 rpm, 30 cycles of 30 s ON time and 30 s OFF time). 800 µL of the solvent mixture was then added to the resultant cell lysate and centrifuged at 14,000 rpm at 4°C for 10 min. The supernatant was then aliquoted into 1 mL samples in new microtubes and an additional 1 mL of the solvent mixture was added to the cell debris. This procedure was then repeated in order to ensure that all cellular lipids were extracted. Lipid content was quantified using the E-test Wako TG kit (Wako Pure Chemical Industries Ltd., Osaka, Japan). To determine the fatty acid composition, lipids were extracted in a mixture of chloroform and methanol (2:1, v/v) as described by Folch et al. [Citation23] using the Multi Beads Shocker (conditions: 4°C, 2500 rpm, 30 cycles of 30 s ON time and 0 s OFF time). 500 µL of the solvent mixture was then added to the resultant cell lysate which was then shaken (250 rpm at room temperature) for 10 min, then the resultant solution was collected in new microtubes. This final step was then repeated to ensure that all cellular lipids were extracted. 1 mL of the supernatant was collected after centrifuging at 14,000 rpm at 4°C for 10 min. The solvent was removed using an ultra-small centrifugal concentrator (TAITEC, Saitama, Japan) and methyl esterification was performed using a fatty acid methylation kit (Nacalai Tesque, Tokyo, Japan). The final lipid content of the total DCW was determined by gas chromatography (GC) analysis using a GC-2014 instrument (Shimadzu, Kyoto, Japan) with a DB-25 column (Agilent Technologies).
Carotenoids were extracted from dry cells in 100% acetone using the Multi Beads Shocker. Dry cells were mixed with 200 µL of acetone and 500 mg of glass beads (0.5 mm diameter), followed by treatment by the Multi Beads Shocker (conditions: 4°C, 2500 rpm, 30 cycles of 30 s ON time and 30 s OFF time). After cell disruption, 800 µL of acetone was added. The mixture was centrifugated at 4°C with 14,000 rpm for 10 min. The 1 mL of supernatant was collected in a new microtube and a further 1 mL of acetone was added to the cell debris to obtain all cellular carotenoids. Infinite M200 PRO (TECAN, Kanagawa, Japan) was used to measure 100 µL carotenoid samples into a 96-well glass plate at 488 nm. Carotenoid production was expressed as the absorbance of samples at 488 nm/g-DCW.
Quantification of gene expression amounts
Yeast cells for total RNA extraction were initially cultured in the dark for 24 h, transferred into either light or dark conditions and sampled at appropriate times post transfer. Total RNA was extracted from frozen mycelia by a modified hot-phenol method using TRIzol®LS (Invitrogen, Carlsbad, USA) and an RNA spin (GE Healthcare, Chicago, USA). Total RNA (1 µg) was reverse-transcribed and complementary DNA was synthesized using a Transcriptor First-Strand cDNA Synthesis Kit (Roche Applied Science, Bavaria, Germany). Quantitative real-time polymerase chain reaction (qRT-PCR) was performed using a Light Cycler® 480 SYBR Green I Master kit (Roche Applied Science, Bavaria, Germany) with 0.5 µM forward primer, 0.5 µM reverse primer and 2 µL of cDNA (100-fold dilution of synthesized cDNA) in a final volume of 20 µL. Thermal cycling was conducted under the following conditions: 5 min at 95°C followed by 45 cycles of 10 s at 60°C, and 10 s at 72°C. The relative expression level was calculated as the ΔCt power of 2, in which ΔCt value was obtained by subtracting the Ct value of the housekeeping gene (ACT1, the gene encoding actin) from that of the target gene. PCR primers for expression analysis are listed in Supplemental Table S1.
Genome sequencing of R. toruloides
Frozen yeast cells were disrupted by Multi beads shocker (Yasui Kikai, Japan) and suspended in extraction buffer (50 mM Tris-HCl pH7.5, 10 mM EDTA·2Na, 1% SDS). After addition of 3 M potassium acetate, the mixture was centrifuged, and the supernatant was purified through RNase treatment, phenol-chloroform treatment and ethanol precipitation. Finally, the precipitated genomic DNA was dissolved in TE buffer.
Genome sequence was analyzed by the PacBio and Illmina Miseq platform. Assembly of PacBio no control filtered subreads (raw reads) was performed by using Canu ver. 1.6, in which the putative genome size was set as 20 Mb. Contig sequences were polished by aligning raw reads to each contig by pbalign ver. 0.3.1 and then correction or sequence error was carried out by Genomic Consensus Ver. 2.20 applied variant Caller (quiver algorithm). This step was repeated five times. In order to perform correction of the contig sequences by using Miseq read, Illumina short reads were trimmed by Trimmomatic ver. 0.38. Trimmed short reads were aligned to contig by bwa ver.0.7.12 and error correction was performed by Pilon ver. 1.22. This step was repeated five times. Among the protein sequences of R. toruloides NP11, IFO1236, IFO0880 opened at JGI website (https://mycocosm.jgi.doe.gov/mycocosm/home), conserved sequences in more than one strain were extracted and mapped to contig sequence. DNA sequences that were mapped the whole length of protein were used as the sample; gene model optimization was performed by etraining program of AUGUSTUS ver. 3.3.1. Finally, AUGUSTUS was applied to the contig sequence for gene prediction.
HPLC analysis
The carotenoid solution after acetone extraction was filtered by a DISMIC®-03CP filter with a pore size of 0.45 µm (Toyo Roshi Kaisha, Japan). Carotenoids were analyzed by an HPLC system equipped with a UV/Vis detector SPD-20A (Shimadzu, Japan). A separation C18 column used was Kinetex C18 (5 µm particles size, 250 × 4.6 mm, Phenomenex, USA). The method was modified by Roland W.S Weber (2007) and P. Davoli (2004) [Citation6,Citation24]. The flow rate was 1 mL/min with a gradient from 70–100% acetone. Absorbance at 450 nm was recorded.
Effect of the various wavelength of the visible light experiment
NBRC 10032 was plated on YPD plate and grown under four condition: blue -, red – and white – light and dark. The blue – and red – light source were used by 3 in 1 LED Lighting Unit 3LH Series (NK System, Japan) with 445 nm irradiation as blue light and 660 nm irradiation as red light. For the white light source, the mixture of 445, 520 and 660 nm were used. The dark condition was established in the incubator.
Results and discussion
Influence of light on the phenotypes of R. toruloides
The influence of light on yeast growth was examined by culturing cells under light irradiation or in the dark. Samples were taken every 24 h to analyze cell turbidity, glucose consumption, DCW and carotenoid and lipid production. Red colourization of cells was observed in light irradiated cultures after 24 h ()). The maximum absorption wavelengths of β-carotene, torulene and torularhodin were 450 (or 454) nm, 482 (or 485) nm and 492 nm, respectively [Citation25–Citation28]. The maximum absorption wavelength of the extracted carotenoids was analyzed over a range of 350–650 nm. Spectral shape exhibited considerable variation but in both light and dark conditions, the maximum absorbance was 488 nm (Supplemental Figure S1). Therefore, we used the value of ABS 488 nm per g-DCW as a measure of carotenoid production.
Figure 2. Growth rate parameters of R. toruloides in the light (white circle) and dark (black circle).
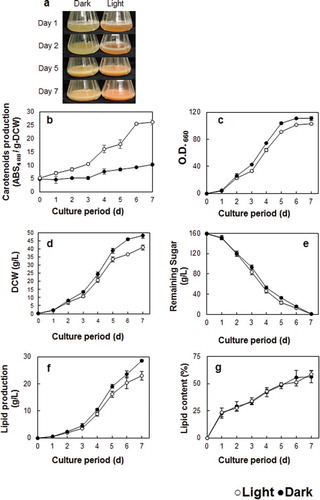
The carotenoid sample from light irradiated cells showed a much higher absorbance than that from cells cultivated in the dark. Indeed, the cell-free extract after six days of cultivation showed that cells grown in the light exhibited a fivefold greater production of carotenoids compared to those grown in the dark ()). It has been previously reported that the fungus Mucor hiemalis produces more carotenoids when grown in the light; carotenoid production in blue and white light was 2.5 and 3-fold of that measured in the dark-grown sample, respectively [Citation19]. Similar results have been also reported in R. glutinis [Citation12,Citation29,Citation30]. Therefore, it is likely that Rhodosporidium species possess a light responsive mechanism for carotenoid production.
The major carotenoids in R. toruloides were analyzed by HPLC. Because of the unavailability of commercial standards of γ-carotene, torulene and torularhodin, the peak area of each carotenoid were directly used for calculation of composition due to similarity in structure and extinction coefficient between β-carotene and γ-carotene, torulene and torularhodin [Citation31]. As the results, β -carotene and torulene were suggested as the major pigments in the R. toruloides NBRC 10032 (Supplemental Figure S2, Table 1). In the dark condition, the amount of β-carotene and torulene were seemed to be similar. However, in the light condition, the peak of β-carotene was higher than that in the dark (Supplemental Figure S2). From the carotenoid composition (Table 1), the ratio of β-carotene was higher under the light. Interestingly, torulene composition under dark was higher than that under the light. This might imply that metabolic steps to β-carotene biosynthesis were affected by light irradiation.
The effect of light irradiation on fungal growth and lipid accumulation was analyzed in a number of different phenotypes of R. toruloides. Our data show that cell density and DCW are both higher in the samples that were grown in the dark (,d)). There was no significant difference in overall glucose consumption between 0–7 d, however, the samples grown in the light consumed the glucose a little earlier ()). Although higher amounts of overall lipid production were observed in the dark-grown samples, lipid content per cell was the same under light and dark conditions (). Growth inhibition by light has been previously reported in other fungi such as R. glutilis and Phaffia rhodozyma [Citation28,Citation29,Citation32]. However, contrasting results were reported by Zhang et al., where samples of R. glutilis grown in the light exhibited lower biomass but similar total lipid content to those grown in the dark [Citation12]. The lower biomass, but similar in lipid content, glucose consumption might suggest that this strain utilized glucose for carotenoid producing, not for growth or lipid producing under light exposure.
A comparison of lipid composition derived from both growth conditions showed that the percentage of unsaturated fatty acids (C16:1, C18:3) was greater in light-grown samples (Table 2). Double bonds in the unsaturated fatty acid are known to act as antioxidants against harmful factors. Thus, increasing the amount of unsaturated fatty acid in response to exposure to light could be a natural defense response in R. toruloides. According to previous studies, co-consumption of dietary lipid, in the form of fats containing rich unsaturated fatty acids, might promote the bioavailability of fat-soluble micronutrients and phytochemicals in Caco-2 cells [Citation33]. Furthermore, fatty acid unsaturation significantly affects the antioxidant role of β-carotenoid by inhibiting lipid peroxidation in homogenous solution [Citation34]. Therefore, oils derived from R. toruloides with a high carotenoid and unsaturated fatty acid content offer great potential as a new source of functional edible oils.
Determination of genome sequence of R. toruloides NBRC 10032
Expression analysis was performed on the genes related to the biosynthesis of carotenoids, fatty acids and triacylglycerols in order to determine whether the observed light-responsive phenotypes were regulated at the transcription level. Firstly, the genome of the strain NBRC 10032, which was used in this study, was sequenced by a combination of next-generation sequencers. The de novo assembly of sequence reads resulted in a 19.4 Mb of sequence with an average coverage of 84 × and 49 × by MiSeq and PacBio platform, respectively. There were 39 assembled contigs and one of them was the mitochondrial DNA and they were deposited to the DNA data bank of Japan (DDBJ) (accession No. BJWK01000001 to BJWK01000039) (Supplemental Table S2). Among them, it is possible that 7 contigs had a telomere in both termini because TTAGGG sequence repeats were detected in both. Therefore, the NBRC10032 strain could have at least 7 contigs with the full length of the chromosome sequence. Genes from R. toruloides that are available in public database were used as a model for predicting NBRC 10032 genes. In addition, two sets of RNA sequence were utilized for intron prediction. The final number of predicted genes was 6,909. Genomic DNA and transcriptome sequences of the R. tourloides NP11 strain have revealed that it has a 20.2 Mb genome and 8,171 protein-coding genes [Citation2]. Other reports have shown that the IFO0880 and CECT1137 strains have 7,920 and 8206 predicted proteins, respectively [Citation35]. In the genome sequence data of the NBRC10032 strain, the number of predicted genes were fewer than those of other reports for Rhodosporidium. To identify genes that NBRC10032 did not possess, bidirectional BLAST analysis was carried out and 1058 genes were extracted. The genes were classified based on the information from NP11 annotation (https://mycocosm.jgi.doe.gov/Rhoto1/Rhoto1.home.html) and comprised: 248 enzymes, 29 transporters, 18 transcription factors, 20 ribosomal proteins, 340 functional proteins and 403 hypothetical proteins (Supplemental Figure S3). We were able to identify genes related to carotenoid biosynthesis, fatty acid biosynthesis and triacylglycerol biosynthesis by BLAST (, Supplemental Table S3). However, MVAK2 encoding mevalonate kinase was predicted in the form of two fusion genes but the boundary was unclear and so it could not be identified.
Expression analysis of major genes related to lipid or carotenoid biosynthesis
Since the enhancement of carotenoid productivity by light irradiation was observed, the gene expression profile of NBRC 10032 was investigated. For expression analysis, cells were cultured under light and dark conditions and RNA was extracted. The general pathway of carotenoid biosynthesis has two main steps; phytoene synthesis and desaturation. In nonphotosynthetic organisms, CrtE and CrtYB encode the enzyme phytoene synthase and CtrI encodes phytoene desaturase. The latter is the enzyme that performs four consecutive desaturations to produce lycopene [Citation36–Citation38]. The genes CAR1 and CAR2 which were identified in the R. toruloides genome were homologues of phytoene desaturase and phytoene synthase, respectively (). Thus, the expression of CAR1 and CAR2 was analyzed. After three days the transcription level of CAR2 and CAR1 in light-grown cells increased to approximately 2-fold and 3-fold of that in dark-grown cells, respectively (). In the carotenogenic fungus Xanthophyllomyces dendrorhous, overall carotenoid content was increased by the overexpression of CrtE, CrtYB and CrtI [Citation39]. It has also been reported that carotenoids were produced in Saccharomyces cerevisiae when carotenoid biosynthesis genes (CrtE, CrtYB and Crt, derived from X. dendrorhous) were overexpressed [Citation37]. Furthermore, albino transformants were observed after deletion of the CAR2 gene in R. toruloides [Citation40]. Therefore, it was likely that the observed increase in carotenoid production in R. toruloides was due to enhanced expression of CAR1 and CAR2 in response to exposure to light.
Figure 3. Effect of light irradiation on the expression of the genes related to carotenoid and lipid biosynthetic pathways.
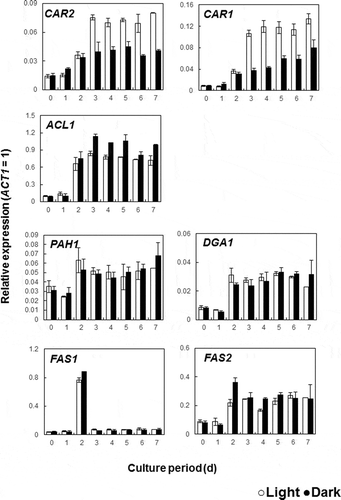
We performed qRT-PCR analysis on several genes involved in the lipid biosynthesis pathway (). ATP citrate lyase (ACL1) is the primary enzyme responsible for the synthesis of acetyl CoA. This is an essential precursor to many biologically important molecules including triacylglycerols, fatty acids and carotenoids. We hypothesized that increased carotenoid production in response to light irradiation could be because the expression of the ACL1 was enhanced by light, and/or that the genes in the fatty acid (FAS1, FAS2) or triacylglycerol (PAH1, DGA1) biosynthesis pathways were down expressed. However, results showed that the expression profiles for the genes FAS2, PAH1 and DGA1 observed in light and dark conditions were very similar (). The only gene that exhibited a difference in expression between the light and dark samples was ACL1. This gene has been shown to play an essential role in fatty acid synthesis in the lipid producing yeast Yarrowia lipolytica [Citation41–Citation43]. In ACL1 inactivated mutants of Y. lipolytica W29, fatty acid content was shown to be dramatically lower and there was an increase in the proportion of unsaturated fatty acids [Citation43]. In this study, the overall amount of lipids () and the transcription level of other lipid producing genes were unchanged () but fatty acid composition showed differences between light- and dark-grown cells. These findings suggest that light does not directly affect lipid production but that it could be influential in determining the fatty acid composition.
Expression of the carotenoid biosynthesis genes in the early cultivation stage
The expression of genes involved in carotenoid biosynthesis was analyzed. The level of transcription of CAR1 and CAR2 was detectably greater at day three in the light samples. However, cultures became visibly red after one day of light irradiation, indicating that carotenoid levels had already increased ()). Furthermore, carotenoids were present in cultures after one day of growth in the light and the levels were greater than those detected in dark-grown cultures ()). These results imply that carotenoid biosynthesis had already occurred during the first day of light irradiation. Therefore, a light stimulating experiment was performed in which dark-grown cells were transferred to light growing conditions and the expression of the genes involved in carotenoid biosynthesis (CAR2 and CAR1) was analyzed. We found that the level of transcription of CAR1 and CAR2 significantly increased after one hour of light irradiation and that the amount of transcripts then decreased after a further two hours. The difference in gene transcription was observed again at 72 h (). Expression of CAR1 and CAR2 was higher in light- compared to dark-grown cells, being three and two folds higher, respectively. This result suggests that CAR1 and CAR2 are regulated by a two steps photoresponse mechanism. Light irradiation leads to the generation of reactive oxygen species that are harmful to microorganisms. Thus, it is possible that R. toruloides produce carotenoids in response to light irradiation as part of a defense system against oxidative stress and that the observed two-step transcriptional response could be a defense system against acute or sustained light irradiation.
Figure 4. Effect of light irradiation on the expression of the genes for mevalonate and carotenoid biosynthetic pathways during the early stages of culture growth.
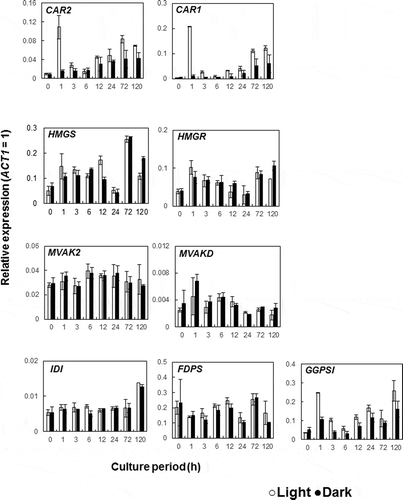
To determine which step of the biosynthetic pathway leading to phytoene was affected by light, genes involved in the mevalonate pathway were analyzed. In order to do this, the following genes were included: HMGS, HMGR, MVAK2, MVAD, IDI, FDPS and GGPSI encode 3-hydroxy-3-methylglutaryl-CoA synthase, 3-hydroxy-3-methylglutaryl-CoA reductase, phosphomevalonate kinase, mevalonate pyrophosphate decarboxylase, isopentenyl pyrophosphate isomerase, farnesyl diphosphate synthase and geranylgeranyl diphosphate synthase, respectively (). A two-step transcription activation profile was also observed in the GGPSI when exposed to light irradiation (), but the expression of genes involved in the upstream of the pathway leading to geranylgeranyl diphosphate did not differ in response to light. Phytoene synthesis is the step that produces the precursor to the carotenoid pathway (). It condenses two GGPP molecules to form phytoene, a colorless C40 carotenoid with only three conjugated double bonds. GGPSI is the first light response gene in carotenoid biosynthesis. It has been hypothesized that the increased level of expression of GGPSI could lead to associated increases in the amount of GGPP which in turn could stimulate carotenoid synthesis.
Light response control factors
Carotenoids are groups of pigments that absorb violet to blue-green light. Differences in the effect of various wavelengths of light on carotenoid formation and composition have been previously reported [Citation19,Citation20,Citation44]. Therefore, the effect of specific wavelengths of light was studied in R. toruloides. Cultures grown on agar were irradiated with blue, red and white light with a dark condition as a control. R. toruloides colonies were darker red in color when exposed to blue and white light (Supplemental Figure S4) compared with red light and dark-grown cultures. This finding suggests that R. toruloides is sensitive to blue light.
The filamentous fungus Neurospora crassa possesses the white-collar complex (WCC), a protein complex formed by the proteins WC-1 and WC-2. It is widely known as the primary photoreceptor system for blue light [Citation18]. The protein complex, WC-1 and WC-2 also are involved in the promotion of carotenoid biosynthesis by light irradiation [Citation45–Citation47]. Cryptochrome DASH has recently been reported to bind to FAD as a coenzyme. It is thought to have evolved from a DNA repair enzyme, dependent on light absorption. It has also been identified as the blue light receptor in plants and has been shown to have been conserved in microorganisms [Citation48–Citation50]. In the draft genome sequence of R. toruloides, there were two genes that encoded a protein that showed homology to WC-1 (37%) and WC-2 (36%) from N. crassa. We also founded a gene that encoded a protein exhibiting high homology (41%) to Cryptochrome DASH from the carotenoid producing yeast X. dendrorhous. Analysis of the expression response of the genes responsible for encoding WC-1, WC-2, and Cryptochrome DASH (CRY1) was performed by qRT-PCR. WC-1 and WC-2 constitutively expressed under light and dark conditions (). In addition, CRY1 was expressed exponentially at one hour of light irradiation, after which it decreased. At 72 h of light irradiation, significant enhancement of gene expression was observed (). These results agree with the expression profile for CAR1 and CAR2 (). It has been speculated that the gene CRY1 plays a role in photoreceptive responses. In the filamentous fungus Fusarium fujikuroi, WcoA which encodes a protein that is highly homology to WC-1 serving as a photoreceptor and cryD act as DASH cryptochrome gene [Citation51,Citation52]. WcoA played a role as light-signaling, acted together cryD when suddenly stimulated light, and promote the expression of carotenoid genes [Citation52]. In comparison with R. toruloides, CRY1 may serve as light-signaling when it overexpressed in the first hour. Rapid response in CRY1 promotes the expression of carotenoid genes resulting in rapid carotenoid producing. Further research is required to determine the role of WC-1 and CRY1 in R. toruloides cell responses to light, and these should include molecular genetic strategies such as gene deletion or overexpression.
Conclusions
In this study, we investigated the effects of light on lipid and carotenoid production. By comparing microbial growth and gene expression patterns, we demonstrated that carotenoid biosynthesis is controlled at the transcription level in response to light. Future research is required to enable us to use R. toruloides at an industrial level in the production of important molecules.
Author’s contributions
Khanh Dung Pham conceived of this study and coordinated the manuscript draft and revision. A. Miyata, T. Takamizawa, Y. Shida, Y. Suzuki, K. Masaki executed the experimental work and data analysis. K. Mori, S. Aburatani, H. Hirakawa, K. Tashiro, S. Kuhara helped with genome data analysis. S. Ara, H. Yamazaki, H. Takaku helped with the fatty acid analysis. Y. Shida, W. Ogasawara participated in the design of the study and helped to revise the manuscript. All authors read and approved the final manuscript.
Supplementary_material.R2.pdf
Download PDF (302 KB)Acknowledgments
We thank Dr. Yoshinori Takahara (Nagaoka University of Technology) for the experiment assistance.
Disclosure statement
No potential conflict of interest was reported by the authors.
Supplementary material
supplemental data for this article can be accessed here.
Additional information
Funding
References
- News. World population projected to reach 9.8 billion in 2050, and 11.2 billion in 2100. UNITED NATIONS-Department Econ. Soc. Aff. 2017.
- Zhu Z, Zhang S, Liu H, et al. A multi-omic map of the lipid-producing yeast Rhodosporidium toruloides. Nat Commun. 2012;3:1–11.
- Botes AL. Affinity purification and characterization of a yeast epoxide hydrolase. Biotechnol Lett. 1999;21(6):511–517.
- Politino M, Tonzi SM, Burnett WV, et al. Purification and characterization of a cephalosporin esterase from Rhodosporidium toruloides. Appl Environ Microbiol. 1997;63(12):4807–4811.
- Kitahara Y, Yin T, Zhao X, et al. Isolation of oleaginous yeast (Rhodosporidium toruloides) mutants tolerant of sugarcane bagasse hydrolysate. Biosci Biotechnol Biochem. 2014;78(2):336–342.
- Davoli P, Mierau V, Weber RWS. Carotenoids and fatty acids in red yeasts Sporobolomyces roseus and Rhodotorula glutinis. Appl Biochem Microbiol. 2004;40(4):460–465.
- Britton G. Carotenoids handbook. 1st ed. Britton G, Liaen-Jensen S, Pfander H, editors. Basel: Birkhäuser; 2004.
- Frengova GI, Beshkova DM. Carotenoids from Rhodotorula and Phaffia: yeasts of biotechnological importance. J Ind Microbiol Biotechnol. 2009;36(2):163–180.
- Buzzini P, Innocenti M, Turchetti B, et al. Carotenoid profiles of yeasts belonging to the genera Rhodotorula, Rhodosporidium, Sporobolomyces, and Sporidiobolus. Can J Microbiol. 2007;53(8):1024–1031.
- Lee JJL, Chen L, Shi J, et al. Metabolomic profiling of Rhodosporidium toruloides grown on glycerol for carotenoid production during different growth phases. Agric Food Chem. 2014;62:10203–10209.
- Saenge C, Cheirsilp B, Suksaroge TT, et al. Efficient concomitant production of lipids and carotenoids by oleaginous red yeast Rhodotorula glutinis cultured in palm oil mill effluent and application of lipids for biodiesel production. Biotechnol Bioprocess Eng. 2011;16(1):23–33.
- Zhang Z, Zhang X, Tan T. Lipid and carotenoid production by Rhodotorula glutinis under irradiation/high-temperature and dark/low-temperature cultivation. Bioresour Technol. 2014;157:149–153.
- Bhosale P, Gadre RV. Optimization of carotenoid production from hyper-producing Rhodotorula glutinis mutant 32 by a factorial approach. Lett Appl Microbiol. 2001;33(1):12–16.
- Lin Y, Jain R, Yan Y. Microbial production of antioxidant food ingredients via metabolic engineering. Curr Opin Biotechnol. 2014;26:71–78.
- Lin X, Gao N, Liu S, et al. Characterization the carotenoid productions and profiles of three Rhodosporidium toruloides mutants from Agrobacterium tumefaciens-mediated transformation Xinping. Yeast. 2017;34(8):335–342.
- Mata-Gómez LC, Montañez JC, Méndez-Zavala A. et al. Biotechnological production of carotenoids by yeasts: an overview. Microb Cell Fact. 2014;13(1):12.
- Alcantara S, Sanchez S. Influence of carbon and nitrogen sources on Flavobacterium growth and zeaxanthin biosynthesis. J Ind Microbiol Biotechnol. 1999;23(1):697–700.
- Linden H, Ballario P, Macino G. Blue light regulation in Neurospora crassa. Fungal Genet Biol. 1997;22(3):141–150.
- Khanafari A, Tayari K, Emami M. Light requirement for the carotenoids production by Mucor hiemalis. Iran J Basic Med Sci. 2008;11:25–32.
- Takano H, Obitsu S, Beppu T, et al. Light-induced carotenogenesis in Streptomyces coelicolor A3(2): identification of an extracytoplasmic function sigma factor that directs photodependent transcription of the carotenoid biosynthesis gene cluster. J Bacteriol. 2005;187(5):1825–1832.
- Braunwald T, Schwemmlein L, Graeff-Hönninger S, et al. Effect of different C/N ratios on carotenoid and lipid production by Rhodotorula glutinis. Appl Microbiol Biotechnol. 2013;97(14):6581–6588.
- Kot AM, Błażejak S, Kurcz A, et al. Rhodotorula glutinis—potential source of lipids, carotenoids, and enzymes for use in industries. Appl Microbiol Biotechnol. 2016;100(14):6103–6117.
- Folch J, Lees M, Stanley GHS. A simple method for the isolation and purification of total lipids from animal tissues. J Biol Chem. 1957;226:497–509.
- Weber RWS, Anke H, Davoli P. Simple method for the extraction and reversed-phase high-performance liquid chromatographic analysis of carotenoid pigments from red yeasts (Basidiomycota, Fungi). J Chromatogr A. 2007;1145(1–2):118–122.
- Lichtenthler HK. Chlorophylls carotenoids. Methods Enzymol. 1987;148:350–382.
- Hornero-Méndez D, Minguez-Mosquera MI. Rapid spectrophotometric determination of red and yellow isochromic carotenoid fractions in paprika and red pepper oleoresins. J Agric Food Chem. 2001;49(8):3584–3588.
- Davoli P, Weber RWS. Carotenoid pigments from the red mirror yeast, Sporobolomyces roseus. Mycologist. 2002;16(3):102–108.
- El-Banna AA, El-Razek AMA, El-Mahdy AR. Some factors affecting the production of carotenoids by Rhodotorula glutinis var. glutinis. Food Nutr Sci. 2012;3:64–71.
- Sakaki H, Nakanishi T, Tada A, et al. Activation of torularhodin production by Rhodotorula glutinis using weak white light irradiation. J Biosci Bioeng. 2001;92(3):294–297.
- Yen HW, Yang YC. The effects of irradiation and microfiltration on the cells growing and total lipids production in the cultivation of Rhodotorula glutinis. Bioresour Technol. 2012;107:539–541.
- Shi Q, Wang H, Du C, et al. Tentative identification of torulene Cis/trans geometrical isomers isolated from Sporidiobolus pararoseus by high-performance liquid chromatography-diode array detection-mass spectrometry and preparation by column chromatography. Anal Sci. 2013;29(10):997–1002.
- Vázquez M. Effect of the light on carotenoid profiles of Xanthophyllomyces dendrorhous strains (formerly Phaffia rhodozyma). Food Technol Biotechnol. 2001;39:123–128.
- Failla ML, Chitchumronchokchai C, Ferruzzi MG, et al. Unsaturated fatty acids promote bioaccessibility and basolateral secretion of carotenoids and α-tocopherol by Caco-2 cells. Food Funct. 2014;5(6):1101–1112.
- Palozza P, Luberto C, Bartoli GM. The effect of fatty acid unsaturation on the antioxidant acitivity of β-carotene and α-tocopherol in hexan solutions. Free Radic Biol Med. 1995;18(5):943–948.
- Zhang S, Skerker JM, Rutter CD, et al. Engineering Rhodosporidium toruloides for increased lipid production. Biotechnol Bioeng. 2016;113(5):1056–1066.
- Sieiro C, Poza M, De Miguel T, et al. Genetic basis of microbial carotenogenesis. Int Microbiol. 2003;6(1):11–16.
- Verwaal R, Wang J, Meijnen JP, et al. High-level production of beta-carotene in Saccharomyces cerevisiae by successive transformation with carotenogenic genes from Xanthophyllomyces dendrorhous. Appl Environ Microbiol. 2007;73(13):4342–4350.
- Lodato P, Alcaíno J, Barahona S, et al. Expression of the carotenoid biosynthesis genes in Xanthophyllomyces dendrorhous. Biol Res. 2007;40(1):73–84.
- Verdoes JC, Sandmann G, Visser H, et al. Metabolic engineering of the carotenoid biosynthetic pathway in the yeast. Appl Environ Microbiol. 2003;69:3728–3738.
- Koh CMJ, Liu Y, Moehninsi, et al. Molecular characterization of KU70 and KU80 homologues and exploitation of a KU70-deficient mutant for improving gene deletion frequency in Rhodosporidium toruloides. BMC Microbiol. 2014;14(1):1–10.
- Wang GY, Zhang Y, Chi Z, et al. Role of pyruvate carboxylase in accumulation of intracellular lipid of the oleaginous yeast Yarrowia lipolytica ACA-DC 50109. Appl Microbiol Biotechnol. 2014;99(4):1637–1645.
- Liu XY, Chi Z, Liu GL, et al. Both decrease in ACL1 gene expression and increase in ICL1 gene expression in marine-derived yeast Yarrowia lipolytica expressing INU1 gene enhance citric acid production from inulin. Mar Biotechnol. 2013;15:26–36.
- Dulermo T, Lazar Z, Dulermo R, et al. Analysis of ATP-citrate lyase and malic enzyme mutants of Yarrowia lipolytica points out the importance of mannitol metabolism in fatty acid synthesis. Biochim Biophys Acta - Mol Cell Biol Lipids. 2015;1851:1107–1117.
- An GH, Johnson EA. Influence of light on growth and pigmentation of the yeast Phaffia rhodozyma. Antonie Van Leeuwenhoek. 1990;57(4):191–203.
- Belozerskaya TA, Gessler NN, Isakova EP, et al. Neurospora crassa light signal transduction is affected by ROS. J Signal Transduct. 2012;2012:1–13.
- He Q, Cheng P, Yang Y, et al. White collar-1, a DNA binding transcription factor and a light sensor. Science (New York, NY). 2002;297:840–843.
- Iigusa H, Yoshida Y, Hasunuma K. Oxygen and hydrogen peroxide enhance light-induced carotenoid synthesis in Neurospora crassa. FEBS Lett. 2005;579(18):4012–4016.
- Yu X, Liu H, Klejnot J, et al. The cryptochrome blue light receptors. Arab B. 2010;8:e0135.
- Gong Z, Shen H, Zhou W, et al. Efficient conversion of acetate into lipids by the oleaginous yeast Cryptococcus curvatus. Biotechnol Biofuels. 2015;8(1):189.
- Facella P, Lopez L, Chiappetta A, et al. CRY-DASH gene expression is under the control of the circadian clock machinery in tomato. FEBS Lett. 2006;580(19):4618–4624.
- Estrada AF, Avalos J. The white collar protein WcoA of Fusarium fujikuroi is not essential for photocarotenogenesis, but is involved in the regulation of secondary metabolism and conidiation. Fungal Genet Biol. 2008;45(5):705–718.
- Castrillo M, Avalos J. The flavoproteins CryD and VvdA cooperate with the white collar protein WcoA in the control of photocarotenogenesis in Fusarium fujikuroi. PLoS One. 2015;10:1–23.