ABSTRACT
Sequences surrounding the miRNA binding domain of the miRNA inhibitor LidNA were selected intracellularly. The library was transfected into cells, and then, inhibitors that were associated with argonaute 2 were selected. The potent inhibitors were slowly degraded intracellularly, while the lower-activity inhibitors were rapidly degraded. A combination of the selected sequences surrounding the miRNA binding domain enhanced miRNA inhibitory activity.
Abbreviations
LidNA: DNA that puts a lid on miRNA function; LNA: locked nucleic acid; Ago2: argonaute 2; LNA: locked nucleic acid
KEYWORDS:
Many miRNA inhibitors have previously been developed, including chemically modified oligonucleotides, such as 2′-O-methylated RNA, and locked nucleic acids (LNAs) [Citation1–Citation7]. Unmodified DNA has not yet been reported as a miRNA inhibitor due to the relatively low affinity of DNA/miRNA binding [Citation1]. In prior studies, we designed a structured DNA, LidNA, that consisted of unmodified DNA, and inhibited miRNA more potently than 2′-O-methylated RNA or LNA [Citation8–Citation10]. LidNA consists of a complementary sequence to the target miRNA flanked by two structured DNA regions, such as double-stranded DNA [Citation8–Citation10]. Surprisingly, variations in the insertion sequence in the middle of the miRNA binding domain significantly affects inhibitor activity [Citation8]. LidNAs with TCGA in the central insertion sequence possessed higher miRNA inhibitory activity, while LidNAs with AATC in the central insertion sequence possessed very low activity [Citation8]. In the present study, we sought to develop more effective sequences around the miRNA binding domain using intracellular selection.
We postulated that potent miRNA inhibitors would tightly bind miRNA on argonaute 2 (Ago2), while less-potent miRNA inhibitors would not bind Ago2-bound miRNA as tightly. The insertion and spacer sequences of LidNA were important for its inhibitory activity. Therefore, we hypothesized that LidNAs with optimal insertion and spacer sequences would tightly bind Ago2, while LidNAs with non-optimal insertion spacer sequences would bind with less affinity, and would therefore be more rapidly degraded intracellularly. Based on this notion, we improved the binding affinity of LidNA ()) [Citation11]. LidNA has a miRNA binding domain between two duplex domains, formed by a closed-structure delta-forming oligo. The duplex formation between the miRNA binding domain and miRNA could place tension on the two flanking spacer sequences. Therefore, we postulated that the two spacer sequences would be important for relaxation of the miRNA binding domain.
Figure 1. (a) Structure and miRNA-binding domain sequence of delta-type LidNA consisting of two strands, the delta forming oligo and d-21. (b) Scheme for intracellular selection of LidNA21.
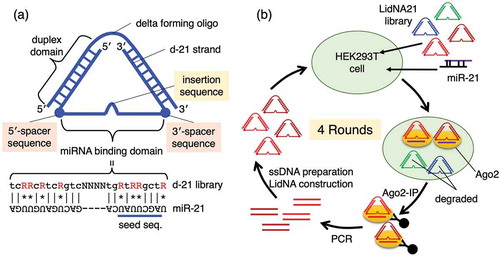
We expected that if a LidNA library with random insertion and spacer sequences was selected intracellularly, tightly bound LidNA could be immunoprecipitated with an anti-Ago2 antibody. However, LidNAs with non-optimal insertion and spacer sequences could also co-precipitate with an anti-Ago2 antibody to some extent, as all LidNAs in the library would have an antisense sequence to bind miRNA on Ago2. Therefore, we designed a LidNA library with a minimal miRNA binding domain, such that LidNAs with sub-optimal spacer sequences would not immunoprecipitate. Thus, the miRNA binding domain of LidNA was modified to a minimal binding sequence. The complementary position to U in miR-21 was replaced with “R”, which was G or A. G and U could pair by Hoogsteen-type pairing with lower binding affinity. Therefore, a LidNA library with this “wobbling” miRNA binding domain was used for intracellular selection of efficient insertion and spacer sequencing. Using this platform, if the insertion and spacer sequences were not efficient, these candidates would not persist intracellularly due to lack of Ago2 binding. On the other hand, efficient insertion and spacer sequences would allow for binding of candidates to Ago2. This selection system was designed to identify effective insertion and spacer sequences rather than to design the most potent miRNA inhibitor possible, such as for clinical applications. Rather, this approach can be used to identify effective insertion and spacer sequences, and this information can be used to construct the most potent possible miRNA inhibitors.
LidNAs for the library consisted of two oligonucleotides, a delta forming oligo and a d-21 library, for example, d-21 N2-N4-N2, 5′-CTCGAGGTCGACGGTATCGNNtcRRcRtcRgtcNNNNtgRtRRgctRNNCTTAACCAGGCTGAACTTGCT-3′. The first “N2” is an underlined 5′-spacer sequence, the second “N4” is an italicized insertion sequence, and the third “N2” is a double-underlined 3′-spacer sequence. The d-21 library contained N2-N4-N2, N2-N3-N2, N2-N2-N2, N2-N1-N2, N2-N0-N2, N1-N4-N2, N0-N4-N2, N2-N4-N1, and N2-N4-N0, as shown in Figure S1. All d-21s could be amplified with a KS primer and a lambdagt10 primer, as shown in Figure S1. The LidNA library was transfected with miR-21 into HEK293T cells. Potent Ago2 binders, which had optimal insertion and spacer sequences, co-precipitated by immunoprecipitation with an anti-Ago2 antibody. On the other hand, non-binders were degraded intracellularly within several days after transfection. Therefore, LidNAs with optimal insertion and spacer sequences can be selected intracellularly ()).
HEK293T cells were cultured on a cell attachment dish in DMEM containing 10% FBS and 1% penicillin-streptomycin (Wako, Japan). Cells were harvested with a conventional trypsin-EDTA method. Harvested cells were seeded onto 24-well plates (6.0 x 104 cells/well). On the subsequent day, the LidNA library (total 20 nM) and 10 nM miR-21 were transfected into cells with Lipofectamine LTX according to the manufacturer’s instructions. After 2 days, cells were harvested and disrupted with 200 µL RIPA buffer containing 50 mM Tris, pH 7.6, 150 mM NaCl, 1% Nonidet P40, 0.5% sodium deoxycholate, and 0.1% sodium dodecyl sulfate. The cell lysate was centrifuged at 12,000 x g for 10 min, the supernatant was added to anti-Ago2 antibody (clone number: 4G8, Wako) with Pierce Protein G Magnetic Beads (Thermo scientific) prepared according to the manufacturer’s instructions. The mixture was incubated at 4°C for 2 h with a rotator. The magnetic beads were separated with a magnetic separation rack, and washed with 200 µL PBS three times, 200 µL TBST three times and 200 µL PBS once, and then eluted with 5 µL 0.1 M glycine, pH 2.0 for 10 min. The eluate was neutralized with 0.75 µL 1.0 M Tris-HCl, pH 8.5, and 0.5 µL RNase was then added to digest the miRNA and mRNA bound to Ago2. The resulting sample was amplified by PCR with a pre-KS primer and a pre-Lambdagt10 primer (Figure S1(c)) for 15 cycles. The sample was amplified by nested PCR with a fluorescein KS primer and a biotin Lambdagt10 primer (Figure S1(c)) for 20 cycles. The amplified dsDNA was bound to Dynabeads® MyOne™ Streptavidin C1 (Invitrogen) and washed twice with 500 µL PBS. Fluorescein-labeled ssDNA was eluted with 30 µL 200 mM NaOH at 37°C for 1 h. The solution was applied to a microspin G-25 column (GE healthcare) to separate ssDNA from NaOH. The purified ssDNA was confirmed with fluorescence and annealed with a delta-forming oligo to form delta LidNA and to apply the next round of selection. After four rounds, the selected LidNAs were amplified by PCR with a KSEcoRI primer and a lambdagt10XbaI primer (Figure S1(c)). PCR products were digested with BsmBI and ligated at the EcoRI/XbaI sites of pUC18. Escherichia coli MRF’ was transformed with the resulting plasmids. Plasmids with about 100 bp insert were purified, and the sequences were determined.
The reporter gene assay was conducted according to our previous report [Citation8]. Briefly, LidNA and a synthetic miR-21 duplex were independently annealed and transfected into HEK293T cells with a reporter gene expression vector, pDsRed2-21target, and an internal standard vector, pGFP, with Lipofectamine LTX according to the manufacturer’s instructions. After 2 days, cell lysates were measured with a fluorescent microplate reader. Activity was expressed as the ratio of DsRed2/GFP. Data were presented as mean ± SD.
At least three biological replicates were included for all experiments. Statistical significance was calculated by ANOVA (Tukey’s test) using SPSS Statistics software (IBM). Asterisks indicate significance as follows: **P < 0.01, ***P < 0.001.
After four rounds, the selected d-21s were cloned, and the sequences were determined as shown in Figure S2. “R” sites in the miRNA binding domain were divided by G and A. All 5′-spacer sequences consisted of two nucleotides. 3′-spacer and insertion sequences varied in length (Figure S2).
We determined the activity of LidNAs with selected insertion sequences and TT sequences at the 5′- and 3′-spacers ()). LidNAs with the TTTA insertion sequence exhibited significantly higher activity than LidNAs with TCGA, the original insertion. The activities of LidNAs with other insertion sequences varied. Next, the TTTA variants with different spacer sequences were evaluated ()). In the present report, we selected AA, GA, and GT, which are found in two of the selected sequences, as shown in Figure S2, as 5′-spacer sequences. Other sequences were not tested. We also selected TT, TA, and G as 3′-spacer sequences, as TT was found in three of the selected sequences, TA was found in two of the selected sequences, and G was the only single sequence (Figure S2). Combinations of 5′-spacer sequences (AA, GA, and GT) and 3′-spacer sequences (TT, TA, and G) were tested ()). TTTA LidNA with GA and G (GA-TTTA-G variant) as 5′- and 3′-spacer sequences, respectively, exhibited significantly higher activity than the TT-TCGA-TT or TT-TTTA-TT variants. Three variants with G in the 3′-spacer were more effective than other spacers, whereas there was no tendency in the 5′-spacer sequences. We speculate that the 3′-spacer sequence was required to be rigid, such as G, not flexible, such as TT, for higher activity, as the 3′-spacer was close to the miR-21 seed sequence binding site, which first bound to miR-21 on Ago2 protein.
Figure 2. (a) miR-21 inhibitory activities of LidNA variants with various insertion sequences. The variant sequences were listed in Figure S3. (b) miR-21 inhibitory activities of LidNA variants with various spacer sequences. The variant sequences were listed in Figure S4. (c) Comparison of original TT-TCGA-TT and GA-TTTA-G variants.
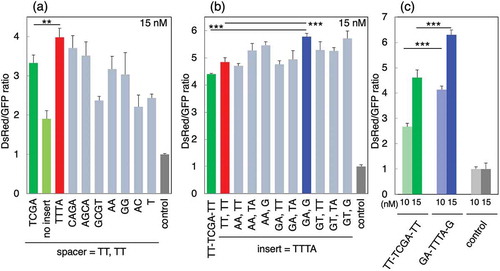
Finally, we compared the activities of the TT-TCGA-TT and GA-TTTA-G variants ()). The GA-TTTA-G variant exhibited much higher activity than the TT-TCGA-TT variant at 10 and 15 nM. The effects of the insertion and spacer sequences were also reported. As tough decoys and miRNA sponges, miRNA inhibitors expressed from DNA vectors also have insertion and spacer sequences [Citation12,Citation13]. Some insertion sequences result in high miRNA inhibitory activity, while other insertion sequences result in low or no activity, the mechanism is as yet unknown [Citation14]. The spacer sequence in tough decoys is usually a flexible sequence, such as TT. However, our results suggested that non-flexible sequences, including GA and G, were optimal.
The shape of delta LidNA should restrict the movement of the miRNA binding site. The conformation of the miRNA binding site can be more strongly affected by spacer sequences than that of the tough decoy. This will be addressed in future studies. In conclusion, we selected potent insertion and spacer sequences of miR-21 inhibitors using an intracellular selection system. We plan to select inhibitors targeting to other miRNAs, and expect similar results.
We confirmed whether potent LidNA miRNA inhibitors survived for 2 days in cells in comparison to less potent LidNAs. Three variants, GA-TTTA-G, TT-TCGA-TT, and TT-no insert-TT, were compared. Fluorescein-labeled LidNA variants (Figure S5(a)) were transfected and analyzed with flow cytometry at 5 h and 2 days after transfection (Figure S5(b)(c)). In this assay, cellular fluorescence was affected by transfection efficiency, LidNA degradation, and fluorescein decay. Although transfection efficiency generally varies between independent experiments, we used the fluorescence decreasing rate (5 h to 2 d) that is affected primarily by LidNA degradation.
Cellular fluorescence in cells transfected with the low-activity LidNA variant, TT- no insert-TT, rapidly decreased. Highly fluorescent cells decreased from 25.6% at 5 h to 2.5% at 2 days. The GA-TTTA-G variant decreased to approximately one-third after 2 days (31.8% to 11.8%), and the TT-TCGA-TT variant decreased by to approximately one-quarter at 2 days (40.0% to 11.5%). The most potent inhibitor, the GA-TTTA-G variant, decreased more slowly than the TT-TCGA-TT variant. The difference in fluorescence intensity between GA-TTTA-G, a potent inhibitor, and TT-no insert-TT, a weak inhibitor, was clear at 2 days. This supported the efficacy of our intracellular selection strategy.
Author contribution
AY performed all experiments. AT analyzed the data and wrote this paper. All authors approved the final version of the manuscript.
supporting.pdf
Download PDF (572 KB)Disclosure statement
No potential conflict of interest was reported by the authors.
Supplementary material
Supplemental data for this article can be accessed here.
References
- Meister G, Landthaler M, Dorsett Y, et al. Sequence-specific inhibition of microRNA- and siRNA-induced RNA silencing. RNA. 2004;10:544–550.
- Robertson B, Dalby AB, Karpilow J, et al. Specificity and functionality of microRNA inhibitors. Silence. 2010;1:10.
- Vermeulen A, Robertson B, Dalby AB, et al. Double-stranded regions are essential design components of potent inhibitors of RISC function. RNA. 2007;13:723–730.
- Hutvágner G, Simard MJ, Mello CC, et al. Sequence-specific inhibition of small RNA function. PLoS Biol. 2004;2:e98.
- Elmén J, Lindow M, Schütz S, et al. LNA-mediated microRNA silencing in non-human primates. Nature. 2008;452:896–899.
- Davis S, Lollo B, Freier S, et al. Improved targeting of miRNA with antisense oligonucleotides. Nucleic Acids Res. 2006;34:2294–2304.
- Kurreck J, Wyszko E, Gillen C, et al. Silencing of microRNAs in vivo with ‘antagomirs’. Nature. 2005;438:685–689.
- Tachibana A, Yamada Y, Ida H, et al. LidNA, a novel miRNA inhibitor constructed with unmodified DNA. FEBS Lett. 2012;586:1529–1532.
- Tachibana A, Saito S, Fujiyama Y, et al. LidNA, a miRNA inhibitor constructed with unmodified DNA, requires an xxxA insertion sequence in miRNA binding site for its potent inhibitory activity. FEBS Lett. 2020. in press. DOI: 10.1002/1873-3468.13756
- Ida H, Tachibana A, Tanabe T. Binding affinity of ssDNA is improved by attachment of dsDNA regions. J Biosci Bioeng. 2014;118:239–241.
- Tachibana A, Komeda Y, Yamamoto A. Structural improvement of LidNA: delta-type LidNA is a potent miRNA inhibitor constructed with unmodified DNA. Biosci Biotechnol Biochem. 2020. in press.
- Haraguchi T, Ozaki Y, Iba H. Vectors expressing efficient RNA decoys achieve the long-term suppression of specific microRNA activity in mammalian cells. Nucleic Acids Res. 2009;37:e43.
- Ebert MS, Neilson JR, Sharp PA. MicroRNA sponges: competitive inhibitors of small RNAs in mammalian cells. Nat Methods. 2007;4:721–726.
- Hooykaas MJG, Soppe JA, De Buhr HM, et al. RNA accessibility impacts potency of tough decoy microRNA inhibitors. RNA Biol. 2018;15:1410–1419.