ABSTRACT
The AcrAB-TolC efflux pump is involved in the organic solvent tolerance of Escherichia coli. Most E. coli strains are highly sensitive to organic solvents such as n-hexane and cyclohexane. Here, a recombinant E. coli transformed with an expression plasmid containing acrAB and tolC became tolerant to n-hexane and cyclohexane. The levels of AcrA, AcrB, and TolC in the recombinant increased by 3- to 5-fold compared to those in the control strain without the plasmid for acrAB or tolC. To investigate the usability of the recombinant as a biocatalyst in an aqueous-organic solvent two-phase system, we further introduced xylMA xylene monooxygenase genes from Pseudomonas putida mt-2 into the recombinant and examined the production of styrene oxide from styrene. The resulting recombinant produced 1.8 mg and 1.0 mg styrene oxide mL−1 of medium in a medium overlaid with a 25% volume of n-hexane and cyclohexane containing 10% (wt vol−1) styrene, respectively.
GRAPHICAL ABSTRACT
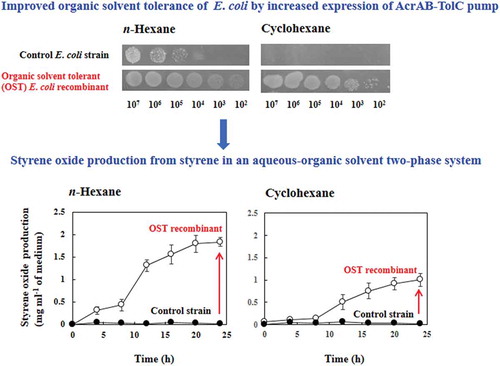
A recombinant Escherichia coli with enhanced AcrAB-TolC efflux pump level increased production of styrene oxide from styrene in an aqueous-organic solvent two-phase system.
Whole-cell biocatalysts have been employed for the bioproduction of fine chemicals requiring multi-step reaction pathways and internal cofactor regeneration such as NAD(P)H. One of the major problems in the application of whole-cell biocatalysts is the growth-inhibitory effect of substrates and products toward microbial cells. A two-phase bioproduction system consisting of an aqueous nutrient medium and an extractive organic solvent phase has advantages for sustaining low concentrations of toxic lipophilic compounds in the aqueous phase and can reduce inhibitory effects on cell viability [Citation1–Citation3]. When organic solvent-sensitive microorganisms are used in a two-phase system, productivity levels are often lowered due to the toxicity of the organic solvent, and thus the range of available organic solvents is limited. Organic solvent-tolerant microorganisms are expected to expand the usefulness of bioproduction in the presence of a wide range of solvents. When bacteria grow in an aqueous-organic solvent two-phase system, the extent of growth inhibition is inversely correlated with the log POW value of the solvent [Citation4]. Hydrophobic organic solvents with a log POW of 2 to 5 can be toxic to most bacteria. It has been shown that energy-dependent efflux systems are necessary for the maintenance and acquisition of solvent tolerance [Citation5–Citation7]. In E. coli, the resistance/nodulation/cell division (RND) family transporter [Citation8] AcrAB-TolC efflux pump plays an important role in intrinsic tolerance to organic solvents [Citation7]. An increase in the AcrAB-TolC efflux pump level leads to the improvement of organic solvent tolerance in E. coli.
Xylene monooxygenase (XylMA) encoded by the TOL plasmid pWW0 of P. putida mt-2 oxidizes toluene and xylenes to (methyl)benzyl alcohols [Citation9]. The enzyme consists of two different subunits encoded by xylMA. XylA is a cytoplasmic NADH:acceptor reductase component that transfers reducing equivalents from NADH to XylM [Citation10]. XylM is a membrane-bound component that functions as a terminal hydroxylase. XylMA has a broad substrate specificity. E. coli recombinant expressing XylMA oxidizes styrene to optically active (S)-styrene oxide with an enantiomeric excess of 95% [Citation11,Citation12]. Optically active styrene oxide derivatives are chiral building blocks that are useful for the synthesis of a variety of pharmaceutical products, and they can be used as key intermediates for the synthesis of more complex chiral organic compounds. One obstacle to the production of styrene oxide from styrene is the toxicity of styrene and styrene oxide to the producers. Therefore, a two-phase bioproduction system has been employed for the production of styrene oxide from styrene to maintain low concentrations of styrene and styrene oxide in the aqueous phase [Citation2,Citation12–Citation15]. In these studies, relatively less toxic solvents such as n-octane (logPow, 4.9) [Citation12], n-dodecane (logPow, 7.0) [Citation2], hexadecane (logPow, 8.8) [Citation13], and bis(2-ethylhexyl)phthalate (logPow, 8.6) [Citation14,Citation15] have been employed as organic solvent layers.
In this study, we constructed a solvent-tolerant E. coli recombinant expressing XylMA and investigated the production of styrene oxide from styrene in an aqueous-organic solvent two-phase system. The results showed that the solvent-tolerant recombinant can expand the range of available organic solvents in the two-phase bioproduction system.
Materials and methods
Bacterial strains and plasmids
Pseudomonas putida mt-2 (ATCC33015) harboring the TOL plasmid pWW0 was used for the source of xylMA xylene monooxygenase genes. The E. coli strains and plasmids used in this study are summarized in . The vector plasmid pMW218 was purchased from Nippon Gene (Tokyo, Japan). pBAD-TOPO vector containing an ampicillin resistance marker for TA cloning was purchased from Thermo Fisher Scientific (Carlsbad, CA, USA). E. coli DH5α and E. coli TOP10 were used as the hosts for the construction of plasmids derived from pMW218 and pBAD-TOPO, respectively. pBADacrABtolC, a pBAD-TOPO derivative containing acrAB and tolC cloned from E. coli W3110 [Citation16], was constructed in this study. pMWxylMA, a pMW218 derivative containing xylMA, was also constructed in this study. An E. coli BW25113-based araA gene knockout mutant (strain JW0061) was supplied by the National Bioresource Project: E. coli (NIG, Mishima, Japan) [Citation17]. The plasmid pCP20 was also obtained from NIG, Japan. E. coli JA300ΔaraA was constructed from strain JA300 [Citation18] as described below.
Table 1. E. coli strains and plasmids used in this study.
Culture conditions
The organisms were grown aerobically at 30°C in LBGMg medium consisting of 1% Bacto Tryptone (Difco Laboratories, Detroit, MI, USA), 0.5% Bacto Yeast Extract (Difco), 1% NaCl, 0.1% glucose, and 10 mM MgSO4 [Citation18]. When necessary, this medium was solidified with 1.5% (wt vol−1) agar and supplemented with ampicillin (50 μg mL−1) and/or kanamycin (25 μg mL−1). Recombinant strains were grown in LBGMg medium containing 0.2 mM isopropyl-β-D-thiogalactopyranoside (IPTG), 0.01% (wt vol−1) L-arabinose, ampicillin, and kanamycin. This medium was named LBGMg(IPTG, Ara, Amp, Km) medium.
Construction of JA300ΔaraA
Since pBAD-TOPO is an arabinose-inducible expression vector, the arabinose degradation-deficient mutant JA300ΔaraA was constructed from JA300 by P1 transduction of kanamycin resistance with BW25113ΔaraA as the donor. The KmR cassette in JA300ΔaraA was eliminated with pCP20 [Citation17]. Elimination of this cassette in the araA region was confirmed by PCR analysis. The combination of primers for araA disruption was araA-S and araA-AS ().
Table 2. Primers used in this study.
Cloning of the acrAB, tolC, and xylMA genes
The region containing acrAB or tolC was amplified by PCR using AccuPrime Taq DNA Polymerase (Thermo Fisher Scientific) with high fidelity and W3110 chromosomal DNA as the template. The primers used were designed according to the genome sequence of W3110 deposited in GenBank (accession number AC_000091.1). The combination of primers for acrAB was acrAB-S and acrAB-AS, and that for tolC was tolC-S and tolC-AS (). The amplified fragments containing both acrAB and tolC were digested with XbaI and ligated to form a fragment containing tandem-arrayed acrAB and tolC genes. The resulting fragment containing acrAB and tolC was ligated into the TA cloning site of pBAD-TOPO. The direction of the insert was confirmed by PCR analysis using a combination of primers of pBAD-F and tolC-AS. A plasmid containing acrAB and tolC under the same direction as the araBAD promoter on pBAD-TOPO was selected and designated pBADacrABtolC. The pBADacrABtolC, digested with NcoI and PmeI, was blunt-ended with T4 DNA polymerase and then self-ligated to create an acrAB and tolC deletion plasmid (pBADN), which was used as a control vector.
TOL plasmid was isolated from P. putida mt-2 harboring the TOL plasmid pWW0 by the method of Hansen and Olsen [Citation19]. The isolated TOL plasmid was digested with SalI and HindIII to obtain a 2.4-kb SalI-HindIII fragment containing xylMA [Citation20,Citation21]. The 2.4-kb fragment was inserted into the SalI-HindIII sites of vector pMW218 under the same direction as Plac. The resulting plasmid was designated pMWxylMA.
Antibodies against AcrA, AcrB, and TolC
Antibodies against AcrA, AcrB, and TolC were obtained as described previously [Citation22].
Immunoblotting analyses
E. coli recombinant strains were grown in LBGMg (IPTG, Ara, Amp) medium. The cells were harvested during the exponential phase of growth by centrifugation (5,000 × g for 10 min at 4°C), when optical density at 660 nm (OD660) reached about 0.6. The cells were suspended in cold 50 mM phosphate buffer (pH 7.0) and broken by sonication in an ice-water bath. The lysate was then centrifuged in the same manner. The supernatant was used for the immunoblotting analyses as previously described [Citation22].
Measurement of the organic solvent tolerance of E. coli
The organic solvent tolerance of E. coli was examined as described previously [Citation22]. Briefly, cultures of E. coli strains in LBGMg medium (OD660, 0.4 to 0.6) were diluted with 0.9% saline by serial 10-fold dilutions. For the recombinant E. coli, ampicillin (50 μg mL−1) was added to the medium. Each suspension was plated on LBGMg agar containing 0.2 mM IPTG and 0.01% (wt vol−1) L-arabinose. This medium was named LBGMg(IPTG, Ara) agar medium. The surface of the agar was overlaid with an organic solvent. The frequency at which the cells formed colonies on the agar was estimated after 48 h incubation at 25°C.
Minimum inhibitory concentrations (MICs) of styrene and styrene oxide against E. coli
The MICs of styrene and styrene oxide were determined by a sequential dilution method [Citation23]. LBGMg medium liquid cultures containing different concentrations of styrene or styrene oxide and 103 freshly grown cells of E. coli strains were incubated at 37°C for 3 h. The MIC was defined as the lowest concentration of styrene or styrene oxide that completely inhibited growth.
Measurement of styrene and styrene oxide
Recombinant E. coli was grown at 30°C in 4 mL of the LBGMg(IPTG, Ara, Amp, Km) medium overlaid with 1 mL of an organic solvent containing 5%(wt vol−1), 10%(wt vol−1), or 20%(wt vol−1) styrene. Screw-capped tubes with a butyl rubber sealant were used for cultivation and were sealed tightly to prevent evaporation of the organic solvent. The cultures were shaken at 150 oscillations min−1 with 4-cm strokes at 30°C. The organic solvent phase solution also contained 0.05 mg mL−1 diphenylmethane used as an internal standard for HPLC analysis. To extract styrene and styrene oxide, 20 mL chloroform was added to the whole two-phase culture. The extracted samples were analyzed by reversed-phase chromatography on a column of octadecylsilica gel (ODS-1251-N; Senshu Science, Tokyo, Japan) attached to the HPLC apparatus. When the styrene concentration in water phase was examined, the samples recovered from the water phase were analyzed by reversed-phase chromatography as described above. The column was eluted with acetonitrile-water (7:3, vol vol−1) at a flow rate of 1.0 mL min−1. The elution was monitored by measurement of A254 to measure the styrene and styrene oxide.
Results
Organic solvent tolerance of recombinant E. coli strains
The organic solvent tolerance of JA300ΔaraA(pBADacrABtolC) was compared to those of control strain JA300ΔaraA(pBADN) and the wild-type strain JA300 by measuring the colony-forming efficiency of each on LBGMg(IPTG, Ara) agar medium overlaid with n-hexane (logPow, 3.9), cyclohexane (logPow, 3.4), and a mixture of p-xylene (logPow, 3.1) and cyclohexane (1:1 vol vol−1 mixture) ()). All strains formed colonies in all spots on the plate without any solvent ()). JA300ΔaraA(pBADN) formed a few colonies only in the spots containing 104 cells in the presence of n-hexane and did not form any colonies on the plate overlaid with cyclohexane ()). The organic solvent tolerance of JA300ΔaraA(pBADN) was similar to that of JA300. In contrast, JA300ΔaraA(pBADacrABtolC) exhibited about 103-fold higher colony-forming efficiency in the presence of n-hexane ()). In addition, JA300ΔaraA(pBADacrABtolC) was able to form colonies in all spots even in the presence of cyclohexane ()). The solvent mixture of cyclohexane and p-xylene (1:1 vol vol−1 mixture) is more toxic than n-hexane and cyclohexane. None of the strains grew on the medium with a mixture of p-xylene and cyclohexane (1:1 vol vol−1) ()).
Figure 1. Colony-forming efficiency of E. coli strains in the presence of organic solvents. JA300, JA300ΔaraA(pBADN), and JA300ΔaraA(pBADacrABtolC) grew on LBGMg(IPTG, Ara) agar overlaid with or without an organic solvent. Colony-forming efficiency of strains was examined on the agar medium in the absence of an organic solvent (a) and in the presence of n-hexane (b), cyclohexane (c), or cyclohexane and p-xylene (1:1 vol vol−1 mixture) (d). Each strain was spotted at a tenfold dilution and incubated at 25°C for 48 h. The spots contained approximately 107, 106, 105, 104, 103, and 102 cells.

Levels of AcrA, AcrB, and TolC in the recombinant E. coli
Expression levels of AcrA, AcrB, and TolC in JA300ΔaraA(pBADN) and JA300ΔaraA(pBADacrABtolC) were investigated by immunoblotting analysis using anti-AcrA, AcrB, and TolC antibodies, respectively ()). The levels of AcrA, AcrB, and TolC in JA300ΔaraA(pBADacrABtolC) were 3.3-, 5.4-, and 3.4-fold higher than those in JA300ΔaraA(pBADN), respectively ()). Thus, the expression levels of the AcrAB-TolC pump in JA300ΔaraA(pBADacrABtolC) increased remarkably compared to those in JA300ΔaraA(pBADN).
Figure 2. Western blot analysis of AcrA, AcrB, and TolC expression. The organisms were aerobically grown in LBGMg (IPTG, Ara, Amp) medium at 30°C. Cell lysates were prepared from cells in the exponential growth phase. Total cell lysate containing 15 μg protein of JA300ΔaraA(pBADN) and JA300ΔaraA(pBADacrABtolC) were separated by a 0.1% sodium dodecyl sulfate-12.5% polyacrylamide gel electrophoresis. Protein was detected by staining with Coomassie brilliant blue R-250 (a) or by using polyclonal anti-AcrA (b), AcrB (c), or TolC (d) antibodies. The expression ratio compared to the level of JA300ΔaraA(pBADN) is shown below each lane. Lanes: M, molecular mass markers (kDa); 1, JA300ΔaraA(pBADN); 2, JA300ΔaraA(pBADacrABtolC).
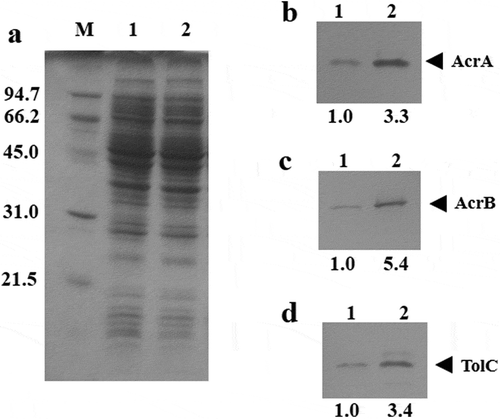
MICs of styrene and styrene oxide against recombinant E. coli
The MICs of styrene or styrene oxide against JA300ΔaraA(pBADN) and JA300ΔaraA(pBADacrABtolC) were investigated with LBGMg medium containing various concentrations of styrene or styrene oxide. The MICs of styrene against JA300ΔaraA(pBADN) and JA300ΔaraA(pBADacrABtolC) were both 0.3 mg mL−1. On the other hand, the MICs of styrene oxide against these two strains were both 1.2 mg mL−1. Thus, enhanced expression of the AcrAB-TolC pump seemed to hardly influence the tolerance of E. coli against styrene and styrene oxide.
Production of styrene oxide from styrene by recombinant E. coli in the two-phase culture system
To evaluate the efficacy of E. coli JA300ΔaraA(pBADacrABtolC) as a biocatalyst in the presence of organic solvents, we used this strain to produce styrene oxide from styrene in the two-liquid-phase culture system. For this purpose, JA300ΔaraA(pBADacrABtolC) was transformed with the plasmid pMWxylMA containing xylMA xylene monooxygenase genes. Production of styrene oxide by JA300ΔaraA(pBADacrABtolC+pMWxylMA) in the presence of organic solvents was compared to that by JA300ΔaraA(pBADN+pMWxylMA) (). These two strains grew in in 4 mL of LBGMg(IPTG, Ara, Amp, Km) medium overlaid with 1 mL of hexadecane, n-hexane, or cyclohexane containing 5% (wt vol−1), 10% (wt vol−1), or 20% (wt vol−1) styrene. In this two-phase cultivation system, the concentration of styrene in the water phase was about 0.02 to 0.2 mg mL−1 (Table S1). Both strains produced 1.6 to 2.1 mg styrene oxide mL−1 of medium in the medium overlaid with 1 mL of hexadecane containing 5% (wt vol−1) and 10% (wt vol−1) styrene. Production of styrene oxide was somewhat decreased in hexadecane containing 20% (wt vol−1) styrene. JA300ΔaraA(pBADN+pMWxylMA) hardly produced styrene oxide in the presence of n-hexane and cyclohexane due to the suppression of growth as described below. In contrast, JA300ΔaraA(pBADacrABtolC+pMWxylMA) produced 0.7 to 1.8 mg styrene oxide mL−1 of medium even in the presence of n-hexane and cyclohexane containing 5% (wt vol−1) or 10% (wt vol−1) styrene. JA300ΔaraA(pBADacrABtolC+pMWxylMA) did not produce styrene oxide in the presence of cyclohexane containing 20%(wt vol−1) styrene. In this condition, the styrene concentration in water phase was close to the MIC (Table S1).
Table 3. Styrene oxide production by recombinant E. coli strains in the two-phase system.
The time courses of styrene oxide production by JA300ΔaraA(pBADN+pMWxylMA) and JA300ΔaraA(pBADacrABtolC+pMWxylMA) in the presence of organic solvents containing 10% (wt vol−1) styrene were examined ()). In the presence of hexadecane, these two recombinants produced similar amounts of styrene oxide, which reached about 2 mg mL−1 of medium in 24 h ()). In the presence of n-hexane and cyclohexane, JA300ΔaraA(pBADN+pMWxylMA) did not grow or produce styrene oxide ()). In contrast, JA300ΔaraA(pBADacrABtolC+pMWxylMA) produced 1.8 mg and 1.0 mg styrene oxide mL−1 of medium after 24 h in the presence of n-hexane and cyclohexane, respectively, although the production of styrene oxide in the presence of n-hexane and cyclohexane was delayed compared to that in the presence of hexadecane ()).
Figure 3. Production of styrene oxide from styrene in the presence of organic solvents. JA300ΔaraA(pBADN+pMWxylMA) (●) and JA300ΔaraA(pBADacrABtolC+pMWxylMA) (○) were grown at 30°C in 4 mL of the LBGMg(IPTG, Ara, Amp, Km) medium overlaid with 1 mL of hexadecane (a), n-hexane (b), or cyclohexane (c) containing 10% (wt vol−1) styrene. At times, chloroform was added to the culture to extract styrene oxide, and the extract was analyzed by HPLC. Values indicate the means and standard deviations of the results of three independent experiments.
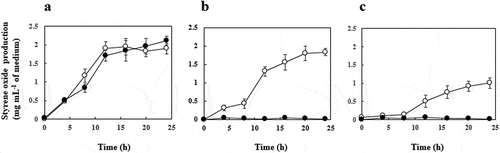
Cell growth during the time course of styrene oxide production was also examined (). The viable cell densities of both recombinants increased to 1.7 to 2.9 × 108 mL−1 after 24 h incubation without any solvent and with hexadecane. The growth of JA300ΔaraA(pBADN+pMWxylMA) was highly suppressed by n-hexane and cyclohexane. Viable cells of JA300ΔaraA(pBADN+pMWxylMA) were not detected after 1 h incubation in the presence of n-hexane and cyclohexane. Although JA300ΔaraA(pBADN) formed colonies at low frequency on the agar medium in the presence of n-hexane ()), JA300ΔaraA(pBADN+pMWxylMA) did not grow in the liquid LBGMg(IPTG, Ara, Amp, Km) medium in the presence of n-hexane containing styrene, probably due to the additional toxicity of styrene. In the case of JA300ΔaraA(pBADacrABtolC+pMWxylMA), the growth rate was decreased by n-hexane and a lag phase was observed in the presence of cyclohexane. However, the cell density of the recombinant increased to 1.1 × 108 mL−1 after 20 h in the presence of n-hexane and to 4.7 × 107 mL−1 after 16 h in the presence of cyclohexane.
Figure 4. Growth of recombinant E. coli strains in the two-phase system. Growth of recombinant E. coli strains was investigated during the course of styrene oxide production. JA300ΔaraA(pBADN+pMWxylMA) (black symbols) and JA300ΔaraA(pBADacrABtolC+pMWxylMA) (white symbols) were grown in 4 mL of LBGMg(IPTG, Ara, Amp, Km) medium overlaid with hexadecane (triangle), n-hexane (square), or cyclohexane (diamond) containing 10% (wt vol−1) styrene as described in Materials and Methods. Growth of these strains in 4 mL of medium without any solvent or styrene (circle) was also examined. Periodically, samples were taken from the water phase in the cultures and the viable cells were counted in terms of the number of colonies that grew on LBG agar medium. Values indicate the means and standard deviations of the results of three independent experiments. Viable cells of JA300ΔaraA(pBADN+pMWxylMA) were not detected after 1 h incubation in the presence of n-hexane and cyclohexane. Therefore, symbols of black square and black diamond are not shown in the figure.
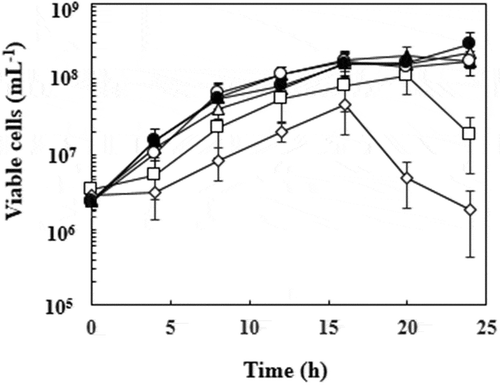
Discussion
The AcrAB-TolC efflux pump is essential for maintaining the intrinsic level of tolerance of E. coli to organic solvents [Citation7]. In the present study, the organic solvent tolerance of E. coli was improved by the introduction of genes coded for AcrA, AcrB, and TolC. In general, the growth of E. coli strains is partially or completely suppressed by organic solvents with logPow ≦ 3.9, including n-hexane and cyclohexane [Citation16]. Our results showed that the colony-forming efficiency and the AcrAB-TolC pump expression of JA300ΔaraA(pBADacrABtolC) increased significantly compared to those of JA300ΔaraA(pBADN).
acrAB and tolC are marA/soxS/rob regulon genes [Citation24]. MarA protein is a transcriptional activator that controls the expression of marA/soxS/rob regulon genes. Overexpression of MarA increases the expression of the AcrAB-TolC efflux pump and raises the organic solvent tolerance of E. coli [Citation23,Citation25]. In the present study, JA300ΔaraA(pBADacrABtolC) formed colonies in spots containing 102 to 107 cells in the presence of cyclohexane. This colony-forming efficiency in the presence of cyclohexane is 104-fold higher than that of JA300 overexpressing the marA gene [Citation25]. We previously reported on E. coli mutant JA300 acrRIS marR carrying a nonsense mutation in marR and found that an insertion of IS5 in acrR exhibited a high level of organic solvent tolerance [Citation22]. The tolerance level of JA300ΔaraA(pBADacrABtolC) was similar to that of JA300 acrRIS marR.
In the present study, we investigated the production of styrene oxide from styrene in the two-phase system. Styrene is a highly toxic compound that completely inhibits E. coli cell growth when supplied as a bulk nonpolar phase [Citation2]. Our results showed that the MICs of styrene and styrene oxide against JA300ΔaraA(pBADN) and JA300ΔaraA(pBADacrABtolC) were both 0.3 mg mL−1. Styrene oxide is also toxic to E. coli strains [Citation14]. The MICs of styrene oxide against these strains were both 1.2 mg mL−1. Thus, there is no difference in the MICs of these compounds between JA300ΔaraA(pBADN) and JA300ΔaraA(pBADacrABtolC). Although the AcrAB-TolC pump recognizes and exports a broad spectrum of chemically dissimilar compounds, it seemed not to be involved in the efflux of styrene and styrene oxide. The concentration of styrene in the water phase was kept low by the addition of organic solvents (Table S1). When the styrene concentration in the water phase was close to the MIC (0.3 mg mL−1), JA300ΔaraA(pBADacrABtolC+pMWxylMA) did not produce styrene oxide (). This recombinant strain produced significant amounts of styrene oxide when the styrene concentration in the water phase was in the range of 0.024 to 0.150 mg mL−1. The styrene oxide production by this recombinant was the highest when it grew in the medium overlaid with 1 mL of hexadecane containing 10% (wt vol−1) styrene. Under this condition, the concentration of styrene oxide in the water phase was about 0.03 mg mL−1 and thus was lower than the MIC of styrene oxide (1.2 mg mL−1).
The bioproduction of styrene oxide from styrene by recombinant E. coli strains in the two-phase system has been studied [Citation2,Citation12–Citation15]. In these studies, relatively low toxic organic solvents with logPow ≧ 4.9 have been used as organic solvent phases. Bioproduction of styrene oxide by E. coli strains in the presence of an organic solvent with a logPow value below 4 has not been reported due to the toxicity of organic solvents. In this study, we succeeded in the bioproduction of styrene oxide in the presence of n-hexane (logPow, 3.9) and cyclohexane (logPow, 3.4) by improving the organic solvent tolerance of E. coli. JA300ΔaraA(pBADacrABtolC+pMWxylMA) produced 0.7 to 1.8 mg styrene oxide mL−1 of medium in the presence of n-hexane or cyclohexane containing 5% (wt vol−1) or 10% (wt vol−1) styrene. There is a tendency that an organic solvent with lower logPow value has fewer carbon numbers and a lower boiling point. The boiling points of n-hexane (68°C) and cyclohexane (81°C) were lower than that of hexadecane (287°C). It is easier for n-hexane and cyclohexane to be removed than hexadecane. Thus, the use of n-hexane and cyclohexane is advantageous for the product recovery.
In the biotransformation in two-phase systems, one of the important criteria for solvent selection is biocompatibility [Citation26]. The selection of appropriate organic solvents generally improves productivity in a two-phase system. Microorganisms with less organic solvent tolerance are not beneficial for a two-phase bioproduction system because the range of available organic solvents is limited. In this study, we showed that the range of available organic solvents for a two-phase bioproduction system can be expanded by using the solvent-tolerant E. coli strain to enhance the expression of the AcrAB-TolC pump.
Author contributions
N. Doukyu designed the experiments and wrote the manuscript. S. Iida and N. Doukyu performed the experiments. All authors reviewed and approved the manuscript.
Supplemental_material.pdf
Download PDF (106 KB)Acknowledgments
This work was supported in part by a Grant-in-Aid for Scientific Research (C) from the Japan Society for the Promotion of Science (JSPS) and by a Grant for the Program for the Strategic Research Foundation at Private Universities S1101017 organized by the Ministry of Education, Culture, Sports, Science, and Technology (MEXT), Japan. We would like to thank Takuma Ise, Yuki Kawabuchi, and Tadashi Sasajima for their technical support.
Disclosure statement
The authors declare no competing financial interests.
Supplementary material
Supplemental data for this article can be accessed here.
Additional information
Funding
References
- Schwartz RD, McCoy CJ. Epoxidation of 1,7-octadiene by Pseudomonas oleovorans: fermentation in the presence of cyclohexane. Appl Environ Microbiol. 1977;34(1):47.
- Wubbolts MG, Favre-Bulle O, Witholt B. Biosynthesis of synthons in two-liquid-phase media. Biotechnol Bioeng. 1996;52(2):301–308.
- Heipieper HJ, Neumann G, Cornelissen S, et al. Solvent-tolerant bacteria for biotransformations in two-phase fermentation systems. Appl Microbiol Biotechnol. 2007;74(5):961–973.
- Inoue A, Horikoshi K. A Pseudomonas thrives in high concentrations of toluene. Nature. 1989;338:264.
- White DG, Goldman JD, Demple B, et al. Role of the acrAB locus in organic solvent tolerance mediated by expression of marA, soxS, or robA in Escherichia coli. J Bacteriol. 1997;179(19):6122–6126.
- Ramos JL, Duque E, Gallegos MT, et al. Mechanisms of solvent tolerance in gram-negative bacteria. Annu Rev Microbiol. 2002;56(1):743–768.
- Tsukagoshi N, Aono R. Entry into and release of solvents by Escherichia coli in an organic-aqueous two-liquid-phase system and substrate specificity of the AcrAB-TolC solvent-extruding pump. J Bacteriol. 2000;182(17):4803–4810.
- Paulsen IT, Brown MH, Skurray RA. Proton-dependent multidrug efflux systems. Microbiol Rev. 1996;60(4):575–608.
- Worsey MJ, Williams PA. Metabolism of toluene and xylenes by Pseudomonas putida (arvilla) mt-2: evidence for a new function of the TOL plasmid. J Bacteriol. 1975;124(1):7–13.
- Shaw JP, Harayama S. Purification and characterisation of the NADH: acceptor reductase component of xylene monooxygenase encoded by the TOL plasmid pWWO of Pseudomonas putida mt-2. Eur J Biochem. 1992;209(1):51–61.
- Mermod N, Harayama S, Timmis KN. New route to bacterial production of Indigo. Bio/Technology. 1986;4(4):321–324.
- Wubbolts MG, Hoven J, Melgert B, et al. Efficient production of optically active styrene epoxides in two-liquid phase cultures. Enzyme Microb Technol. 1994;16(10):887–894.
- Panke S, Meyer A, Huber C, et al. An alkane-responsive expression system for the production of fine chemicals. Appl Environ Microbiol. 1999;65(6):2324–2332.
- Volmer J, Schmid A, Bühler B. The application of constitutively solvent-tolerant P. taiwanensis VLB120ΔCΔttgV for stereospecific epoxidation of toxic styrene alleviates carrier solvent use. Biotechnol J. 2017;12(7):1600558.
- Kuhn D, Buhler B, Schmid A. Production host selection for asymmetric styrene epoxidation: Escherichia coli vs. solvent-tolerant Pseudomonas. J Ind Microbiol Biotechnol. 2012;39(8):1125–1133.
- Aono R, Tsukagoshi N, Yamamoto M. Involvement of outer membrane protein TolC, a possible member of the mar-sox regulon, in maintenance and improvement of organic solvent tolerance of Escherichia coli K-12. J Bacteriol. 1998;180(4):938–944.
- Baba T, Ara T, Hasegawa M, et al. Construction of Escherichia coli K-12 in-frame, single-gene knockout mutants: the Keio collection. Mol Syst Biol. 2006;2(1):2006.0008.
- Aono R, Aibe K, Inoue A, et al. Preparation of organic solvent-tolerant mutants from Escherichia coli K-12. Agric Biol Chem. 1991;55(7):1935–1938.
- Hansen JB, Olsen RH. Isolation of large bacterial plasmids and characterization of the P2 incompatibility group plasmids pMG1 and pMG5. J Bacteriol. 1978;135(1):227–238.
- Harayama S, Leppik RA, Rekik M, et al. Gene order of the TOL catabolic plasmid upper pathway operon and oxidation of both toluene and benzyl alcohol by the xylA product. J Bacteriol. 1986;167(2):455–461.
- Suzuki M, Hayakawa T, Shaw JP, et al. Primary structure of xylene monooxygenase: similarities to and differences from the alkane hydroxylation system. J Bacteriol. 1991;173(5):1690–1695.
- Watanabe R, Doukyu N. Improvement of organic solvent tolerance by disruption of the lon gene in Escherichia coli. J Biosci Bioeng. 2014;118(2):139–144.
- Asako H, Nakajima H, Kobayashi K, et al. Organic solvent tolerance and antibiotic resistance increased by overexpression of marA in Escherichia coli. Appl Environ Microbiol. 1997;63(4):1428–1433.
- Barbosa TM, Levy SB. Differential expression of over 60 chromosomal genes in Escherichia coli by constitutive expression of MarA. J Bacteriol. 2000;182(12):3467–3474.
- Shimizu K, Hayashi S, Kako T, et al. Discovery of glpC, an organic solvent tolerance-related gene in Escherichia coli, using gene expression profiles from DNA microarrays. Appl Environ Microbiol. 2005;71(2):1093–1096.
- Bruce LJ, Daugulis AJ. Solvent selection strategies for extractive biocatalysis. Biotechnol Prog. 1991;7(2):116–124.