ABSTRACT
Mollusk shell is a product of biomineralization with excellent mechanical properties, and the shell matrix proteins (SMPs) have important functions in shell formation. A vWA domain-containing protein (VDCP) was identified from the shell of Mytilus coruscus as a novel shell matrix protein. The VDCP gene is expressed at a high level in specific locations in the mantle and adductor muscle. Recombinant VDCP (rVDCP) showed abilities to alter the morphology of both calcite and aragonite, induce the polymorph change of calcite, bind calcite, and decrease the crystallization rate of calcite. In addition, immunohistochemistry analyses revealed the specific location of VDCP in the mantle, the adductor muscle, and the myostracum layer of the shell. Furthermore, a pull-down analysis revealed eight protein interaction partners of VDCP in shell matrices and provided a possible protein–protein interaction network of VDCP in the shell.
GRAPHICAL ABSTRACT
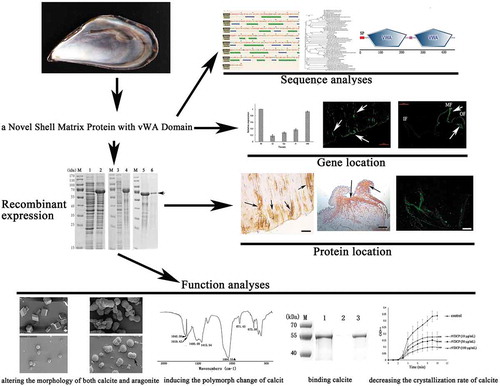
Sequence characterization, recombinant expression, biomineralization-related function, and localization of a vWA domain-containing protein (VDCP) identified from Mytilus corusus shell.
Mollusk shell has been important in the fields of bioengineering and bionic research [Citation1,Citation2]. The shell of mollusks usually consists of an outer uncalcified periostracum and inner mineral layers, among which the periostracum constitutes the “skin” covering the outside of the entire shell [Citation3,Citation4]. The shell mineral layer with excellent mechanical properties is mainly composed of calcium carbonate crystals [Citation5]. In addition, the shell contains small amounts (<5%) of organic matrices consisting principally of shell matrix proteins (SMPs). SMPs play important roles in the formation and the mechanical properties of the shell, although the underlying mechanisms have not yet been elucidated in detail [Citation6]. Under the control of SMPs, calcium carbonate crystals are deposited, form nanostructures with different morphologies and polymorphs, and assemble into a complete shell during biomineralization [Citation6,Citation7]. Studies of the structure and function of SMPs will help researchers understand the process of shell formation and the molecular mechanism underlying the excellent mechanical properties of the shells.
Mytilus coruscus is a mussel with important economic value in the Eastern China Sea. The shell is composed of nacre, myostracum, and fibrous prism layers, and more than 60 SMPs were previously identified in the shell of M. coruscus using transcriptomic and proteomic strategies [Citation8]. Among the shell proteome, 8 SMPs were detected from the myostracum layer, including a vWA domain-containing protein (VDCP, GenBank accession number: AKS48142.1) [Citation8]. The myostracum is buried in the shell nacre (or folia), and, on the other hand, exposed on the inner surface of the shell located at the attachment of the adductor muscle, forming the adductor muscle scar (AMS) of each shell valve [Citation8,Citation9]. The myostracum layer plays an important role in muscle-shell attachment by controlling the closure of shells. However, studies on the organic matrix and the structural roles of the myostracum layer are limited.
VDCP exhibited a sequence similarity to collagens of other species, with a sequence identity <40%. Collagens and collagen-like proteins had been identified from the shells of various mollusks and the function of this protein group remains unclear [Citation10,Citation11]. In general, collagens are structural proteins with a wide distribution in organisms that play important roles in skin, tendon, bone, and cartilage. In vertebrates, collagens have been confirmed to be involved in the biomineralization and hardness of hard tissues, such as bones and teeth, where the main mineral is calcium phosphate rather than calcium carbonate [Citation12–Citation15]. VDCP has two von Willebrand type A (vWA) domains, showing a similarity of domain composition with some reported SMPs, such as Pif [Citation16] and BMSP [Citation17] with vWA domain and chitin-binding domain. Pif and BMSP had been reported with biomineralization-related functions, such as calcium carbonate binding and induction of aragonite crystal formation [Citation16–Citation18]. VDCP, Pif, and BMSP have no similarity in their primary structures, but still share the characteristics of having vWA domain. In addition, VDCP has only vWA domain without other domains, such as chitin-binding domain contained in Pif and BMSP [Citation16,Citation17]. This feature makes VDCP a molecule model for exploring the function of vWA domain in biomineralizaton. Therefore, the recombinant VDCP protein was expressed and the functions of this recombinant production were investigated to explore the mechanisms of VDCP in biomineralization. Furthermore, the location of VDCP in the mantle, adductor muscle, and shell surface, and the possible protein interaction partners of VDCP were analyzed in this study. Our findings provide clues and offer deeper insights into the molecular mechanisms of vWA domain proteins in biomineralization.
Materials and methods
Analysis of the sequence and expression of the VDCP gene in M. coruscus tissues
The full-length cDNA sequence of VDCP was screened out from the transcriptomic data of M. coruscus mantle based on the LC-MS/MS data [Citation8]. The cDNA sequence of VDCP was confirmed using PCR with primers – CCATATTGTAAGACCAGGATTG – (forward primer) and – ACCATTGATAGCAGTAAGCA – (reverse primer), and further verified by PCR production sequencing. The sequence of VDCP was analyzed using conventional bioinformatics tools, including ORF Finder, BLAST, MEGA 7.0, SignalP Server, SMART domain prediction, Phyre secondary structure prediction, and SWISS MODEL tertiary structure prediction software.
Total RNA was extracted from various tissues of six individual mussels, including the mantle, adductor muscle, gill, blood, and gonad, using TRIzol reagent. The first strand of cDNA templates was synthesized using the PrimeScriptTM RT kit (Takara). The quantitative real-time PCR (qRT-PCR) analyses were performed in three independent replicates using SYBR® Premix Ex Taq™ (Takara) on a MX3000P Real-Time PCR System (Stratagene, US). qRT-PCR was performed with specific primers derived from the sequence of VDCP, including VDCP/F (ATCACCGATGCTAACTTCC) and VDCP/R (TAATCCCTTCCCTCTTGG). The relative expression levels were determined using the 2−ΔΔCt method [Citation19], with β-actin serving as an internal reference. The cycling conditions were 3 min at 94°C followed by 40 cycles of 94°C for 10 s, 60°C for 40 s, and 72°C for 30 s. Statistical analysis of differences was performed by SPSS 16.0 with one-way ANOVA followed by Tukey’s multiple range tests. Differences were considered significant at P < 0.05.
In situ hybridization of VDCP
In situ hybridization was performed to determine the locations at which the VDCP mRNA was expressed in the mantle and the adductor muscle. The fixed tissues were dehydrated through a series of ethanol solutions and then incubated in a xylene bath prior to embedding in paraffin. Paraffin blocks were sectioned at a thickness of 5 μm. After treatment with proteinase K at 37°C for 20 min, the tissue sections were washed with a freshly prepared solution of 0.1 M glycine for 1 min and PBS for 2 min, respectively. The tissue sections were immediately fixed with 4% paraformaldehyde for 10 min, followed by an incubation with a FAM-labeled probe (5ʹ-FAM-ACCAUUGAUAGCAGUAAGCACAUCUGUC-3ʹ) at 65°C for 48 h. The tissue sections were washed with formamide in 4X SSC at 60°C. The signals for control samples were visualized with a substrate 4ʹ,6-diamidino-2-phenylindole (DAPI) reagent and the positive signals were visualized by the excitation wavelength (492 nm) and the emission wavelength (518 nm).
Expression and purification of recombinant VDCP
The VDCP gene encoding the mature peptide was codon-optimized and synthesized for expression in an E. coli system. BamH I and Xho I restriction sites were attached to the 5ʹ and 3ʹ ends of the optimized sequence, respectively. The synthetic codon-optimized genes were excised by BamH I and Xho I digestion and ligated into a pET/32α expression vector. The construct was designed to yield a recombinant protein product with a molecular weight (MW) of about 70 kD that was fused in frame with a His6 tag, Trx tag, and enterokinase cleavage site.
The recombinant VDCP (rVDCP) was expressed in E. coli strain BL21 (DE3). E. coli cells containing rVDCP/pET/32α were grown in liquid Luria-Bertani (LB) medium (Sangon Biotech, Shanghai, China) supplemented with 10 μg/mL ampicillin at 37°C. Isopropyl-D-thiogalactopyranoside (IPTG) was added at a final concentration of 1 mM to induce the expression of recombinant VDCP. The induced cells were grown for 4 h, harvested and centrifuged at 1 000 × g for 15 min at 4°C, and the pellets were stored at −20°C until further use.
The cell pellets were dissolved in ice-cold lysis buffer (10 mM imidazole, 50 mM PBS, 100 mM NaCl, and 1 M EDTA, pH 8.0) and homogenized with a sonicator at 4°C. The inclusion bodies were harvested by centrifugation (8 000 × g, 10 min, 4°C) and dissolved in a buffer (10 mM imidazole, 8 M urea, 100 mM NaCl, and 100 mM PBS, pH 8.0) overnight at 4°C. Using a Ni-NTA column (Sangon Biotech, Shanghai, China), rVDCP was isolated and eluted with elution buffer (300 mM imidazole, 8 M urea, 100 mM NaCl, and 100 mM PBS, pH 8.0). The isolated rVDCP protein was refolded in a buffer containing oxidized and reduced glutathione (GSH/GSSG), and dialyzed against a graded of concentrations of urea (0–8 M) according to a previously reported protocol [Citation20].
After refolding, rVDCP was digested with enterokinase to remove the translated vector sequence at the N-terminus of rVDCP. The digestion was performed in a buffer (250 mM Tris-HCl, 500 mM NaCl, and 2 mM CaCl2, pH 7.6) containing 2 IU of enterokinase for 16 h at room temperature. Digested rVDCP was isolated using high-performance liquid chromatography (HPLC, Waters 650E, USA) with a reverse-phase C4 column (4.6 mm×250 mm, 300 Å, Agilent). The protein fraction eluted from the HPLC column was lyophilized and stored at −20°C until use. SDS-PAGE was performed using a 12% polyacrylamide gel, and the protein bands were visualized by staining the gel with Coomassie Brilliant Blue R-250.
Analysis of rVDCP functions
In vitro crystal growth experiments [Citation21] were performed to test the effects of rVDCP on the morphology of calcite and aragonite crystals. The rVDCP protein was incubated with a freshly prepared saturated solution of calcium carbonate [Citation22] on a siliconized cover glass with or without magnesium chloride (40 mM). Crystallization experiments were conducted with various concentrations of rVDCP (10, 50, and 100 μg/mL). The changes in the morphology of calcium carbonate crystals induced by rVDCP were observed with a Nova nano 450 (FEI, USA) scanning electron microscope (SEM). Polymorphs of calcium carbonate crystal were further identified using FTIR spectroscopy (Nicolet Nexus 670).
Crystal binding experiments were performed to test the interaction between rVDCP and calcite or aragonite calcium carbonate. The calcite and aragonite crystals were freshly prepared using the methods described by Yan, et al. [Citation22]. The rVDCP protein was dissolved (1 mg/mL, as sample I) and incubated with calcite or aragonite crystals at room temperature for 1 h. After centrifugation (10 000 × g for 15 min), the supernatants were collected as sample II. The sediments were decalcified and centrifuged (10 000 × g for 15 min), and the supernatants were dialyzed (1 kD cut off) and designated as sample III. Samples I–III were analyzed using SDS-PAGE.
The ability of rVDCP to inhibit calcium carbonate precipitation was determined using the methods described by Liang, et al. [Citation21], with minor modification. Briefly, 10 mM calcium chloride containing various concentrations of rVDCP (10, 50, and 100 μg/mL) was dropped in a 96-well plate, and the plate was placed in a closed desiccator. Solid ammonium carbonate was added to the desiccator to induce calcium carbonate precipitation. The turbidity of the calcium chloride solution was monitored every minute for 10 min by measuring the absorbance at 630 nm with a microplate reader (Synergy H1, BioTec).
Polyclonal antibody preparation and immunohistochemistry analysis
The purified rVDCP protein was concentrated and submitted to HuaAn Biotechnology Co., Ltd. (Hangzhou, China) to produce a polyclonal antibody. Briefly, the polyclonal antibody was prepared by immunizing New Zealand rabbits with 0.5 mL of rVDCP (1 mg/mL) along with an equal volume of complete adjuvant. Three booster injections each containing 0.5 mL of rVDCP (1 mg/mL) plus incomplete adjuvant were subsequently administered at 1-week intervals. The antiserum was collected through the carotid artery 7 d after the last immunization and further purified on a Protein A/G column.
The specificity of the antibodies was assayed by performing western blotting of soluble and insoluble matrices extracted from three layers (nacre, myostracum, and fibrous prism) of M. coruscus shell. Shell matrices were extracted from the shell as described previously [Citation8] and isolated using SDS-PAGE. The SDS-PAGE gel was then transblotted onto a PVDF membrane. The anti-rVDCP polyclonal antibody (1:2 000) was used as the primary antibody, and a horseradish peroxidase-labeled goat anti-rabbit IgG (1:10 000; HuaAn Biotechnology Co., Ltd.) served as the secondary antibody. Blots were visualized using a stabilized 3, 3ʹ, 5, 5ʹ-tetramethylbenzidine substrate.
The tissues (mantle and adductor muscle) of M. coruscus were collected, fixed with 10% formaldehyde overnight, and then dehydrated through ascending grades of ethanol solutions to determine the tissue distribution of endogenous VDCP. Sections (4 μm) were cut with a microtome and collected on coated slides for immunohistochemistry. After de-waxing and rehydration, the slides were incubated with the anti-rVDCP antibody (1:200) supplemented with 1% BSA overnight at 37°C. The primary antibodies were detected using a peroxidase-conjugated antibody against rabbit IgG and stained with a DAB solution. The sections were examined and photographed using a microscope (DFC450C, Leica, Germany).
Using the anti-rVDCP antibody, the location of VDCP on the inner surface of the shell was determined by performing immunofluorescence staining according to the technique described by Kong et al. [Citation23], with some modifications. Briefly, the shell was cut into pieces of ~1 cm2 containing the adductor muscle scar. The shell samples were washed and sonicated in 5% NaOH to remove remaining adductor muscles and organic contaminants on the shell surface, after which the sample was soaked in a stationary liquid containing 10% formaldehyde and 4% formic acid for 24 h. Then, the shell pieces were cleaned, treated with 0.25% Triton X-100 for 30 min, and blocked with 10% negative goat serum for 1 h at 37°C. Immunostaining was performed by sequentially incubating the shell samples with the anti-rVDCP polyclonal antibody (1:50) and Alexa Fluor 488-conjugated goat-anti-rabbit antibody (1:500). Stained sections were examined with a fluorescence microscope (DMIL LED FLUO, Leica, Germany) equipped with a DFC450C digital imaging system (Leica, Germany). The deproteinized shell samples treated with 20% NaOH at 60°C for 1 h were used as negative controls.
His tag affinity pull-down
The rVDCP protein contains a His6 tag in its sequence, and Ni-NTA beads (Sangon, China) were used to bind the tagged rVDCP protein. After binding to rVDCP, the Ni-NTA beads were washed with binding buffer (20 mM Tris-HCl, 150 mM NaCl, and 10 mM imidazole, pH 8.0) incubated with total proteins extracted from the shell of M. coruscus [Citation8] for 4 h at 4°C, and then washed with elution buffer (20 mM Tris-HCl, 300 mM NaCl, and 300 mM imidazole, pH 8.0). Eluted protein samples were analyzed using LC-MS/MS after digestion with trypsin. LC-MS/MS experiments were performed on a Q Exactive Plus MS coupled with an Easy nLC (Thermo Scientific). The MS data were analyzed using MaxQuant software (version 1.6.1.0) and searched against the mantle transcriptome database of M. coruscus (accession number: SRX792025) [Citation8]. The results retrieved from the database search were filtered and exported with a < 1% false discovery rate (FDR) at the peptide-spectrum-matched level, and protein level, respectively. The Ni-NTA beads without binding to rVDCP were used as a control, and the identified nonspecific proteins pulled down by blank Ni-NTA beads were removed accordingly from the list of identified protein partners of rVDCP.
Results
Features of the VDCP sequence
According to the bioinformatics analysis, the full-length sequence of the native VDCP cDNA was 1 640 bp, and the open reading frame (1 363 bp) encodes a 453 amino acid (AA) precursor containing a 22-AA signal peptide (), a theoretical molecular weight of 47.7 kD and an isoelectric point (pI) of 7.42 for the mature region without the signal peptide. Two vWA domains (residues 35–223 and 259–440, respectively) were detected in the VDCP precursor (). The secondary structure of VDCP is primarily composed of 34% α-helices and 18% β-sheets (). In the predicted tertiary structure of VDCP, the β-sheets are mainly located inside the molecule and surrounded by the α-helices, presenting structural characteristics of a typical Rossmann fold [Citation24]. A protein Blast search revealed that VDCP shares low sequence (25 ~ 40%) identity with collagens and matrilins from other species (Supplementary Table 1), and the amino acid composition of VDCP is characterized by abundant of hydrophobic (Val, Leu, and Thr) and charged amino acids (Asp, Arg, Lys, and Glu) (Supplementary Table 2). Forty representative homologous, together with 17 vWA domain-containing SMPs, were selected to build a phylogenetic tree. In the phylogenetic tree, three conspicuous branches, including martrilin/cartilage matrix proteins, collagens, and Pif/BMSP branches, were present (). The clade of VDCP is located closely with collagen XIV, and further grouped with collagen (VI), BMSP, and Pif branches ().
Figure 1. Alignment of the cDNA sequence with the deduced amino acid sequence of VDCP (GenBank AKS48142.1). The signal peptide was underlined, and the termination codon was denoted by an *.
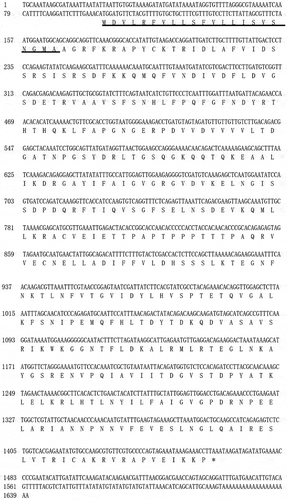
Figure 2. The structural features of VDCP. a: Domains of VDCP were predicted by SMART and the signal peptide (SP) and two VWA domains were showed. b: The secondary structure of VDCP predicted by Phyre. The regions adopting putative α-helix and β-sheet are represented as spiral and arrow, respectively.
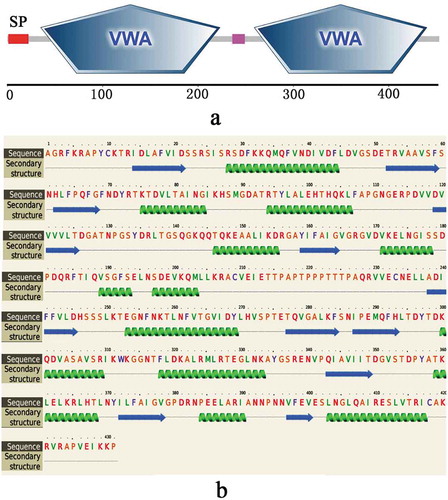
Figure 3. Phylogenetic tree of VDCP. The phylogenetic tree was constructed by Neighbor-joining method using MEGA 7.0 with bootstrap of 1000. Homologous proteins included in the phylogenetic tree were retrieved from NCBI nr database with high BLAST score and Pif/BMSP from mollusks. The VDCP was denoted by a circle. The BLAST information of selected sequences are shown in Supplementary Table 1.
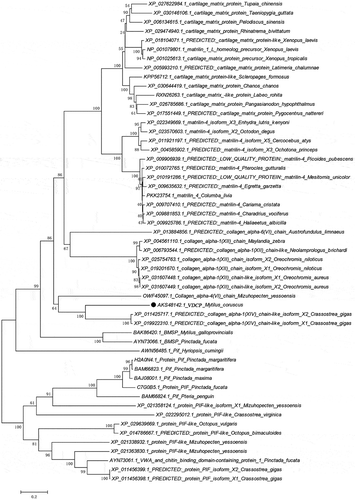
Tissue expression and in situ hybridization
The tissue-specific expression of VDCP was investigated using qRT-PCR. The VDCP mRNA was detected in all five tested tissues, including the mantle, adductor muscle, gill, blood, and gonad. VDCP was expressed at the highest level in the mantle, followed by the adductor muscle ( a). Furthermore, using FAM-labeled VDCP-specific probes, strong signals were mainly detected at the bottom of the adductor muscle near the shell inner surface ()), and the edge of the middle fold and the outer fold of the mantle ()).
Figure 4. Tissue-specific expression (a) and in situ hybridization (b–e) of VDCP. M, mantle; Gi, gill; Go, gonad; H, hemolymph; AM, adductor muscle; F, Foot. Values for qPCR are means ± SD of three replicates. *, p < 0.05; **, p < 0.01. b: Control sample for the adductor muscle; c: expression of VDCP (green color, denoted by arrows) in the adductor muscle; d: control sample for the mantle; e: expression of VDCP (green color, denoted by arrows) in the mantle. IF, inner fold. MF, middle fold. OF, outer fold. The scale bar, 500 μm.
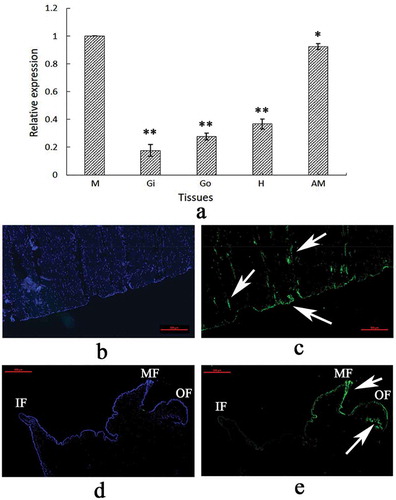
Expression and purification of recombinant VDCP
The codon-optimized VDCP gene was successfully expressed in an E. coli system after induction with IPTG. The results of SDS-PAGE revealed that the rVDCP protein was mainly expressed in inclusion bodies, with an expected theoretical MW of ~66 kD for the tags-containing protein (). The rVDCP protein was isolated with a Ni column, refolded, and then digested with enterokinase to remove the N-terminal tags from rVDCP. As shown in , the MW of rVDCP without the N-terminal tags is ~55 kD, similar to the MW of native VDCP, indicating that the tags were removed successfully. Using HPLC, the digested rVDCP protein was further purified, and high purity was achieved.
Figure 5. Recombinant expression and purification of VDCP. Lane M, Protein marker; Lane 1, negative control (without IPTG induction); Lane 2, recombinant expression of rVDCP with the induction of IPTG; Lane 3, the supernatant of cell lysate; Lane 4, debris of cell lysate; Lane 5, eluted sample of rVDCP with imidazole at concentration of 300 mM; Lane 6, eluted sample of rVDCP with imidazole at concentration of 500 mM; The protein band with ~70 kDa (indicated by an arrow) corresponds to the rVDCP.
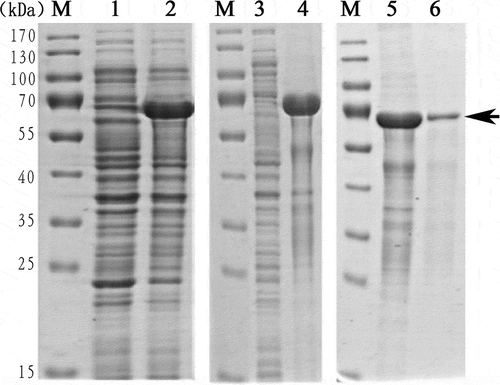
Figure 6. Enterokinase digestion and HPLC purification of rVDCP after digestion. a: Lane M, Protein marker; lane 1, rVDCP before enterokinase digestion; lane 2, rVDCP after enterokinase digestion. The protein band with ~55 kDa (indicated by an arrow) corresponds to the target protein. b: Elution profile of enterokinase digested rVDCP on HPLC with a reverse-phase C4 column. The arrow indicates the target peak and the band of purified rVDCP with ~55 kDa.
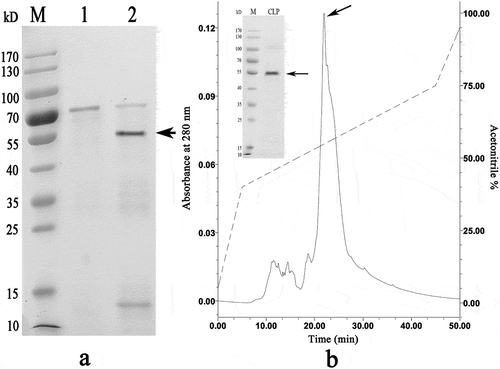
The functions of rVDCP
An in vitro calcium carbonate crystallization assay was performed and the results revealed the effects of rVDCP on the morphology of both calcite and aragonite crystals (, ). For the negative control calcite groups, the crystals were typical rhombohedra without protein induction or with 50 μg/mL BSA induction ()). When rVDCP was added at increasing concentrations, the morphology of calcite crystals changed from an irregular sphere (10 μg/mL rVDCP) to an elliptical sphere (50 μg/mL rVDCP) and a dumbbell shape (100 μg/mL rVDCP). For the aragonite crystals grown in the presence of magnesium, rVDCP also exerted significant effects on the crystal morphology. As shown in , the natural globular morphology of the aragonite crystals changed with the addition of increasing concentrations of rVDCP from a globular splitting morphology to a peanut shape. FTIR was used to characterize the polymorphs of induced crystals. As shown in , the carbonate ions in the mineral were demonstrated by the internal vibration modes of the CO32− ions: 700, 711(υ4)–856, 875(υ2)–1082(υ1), and 1488(υ3) cm−1, and the splitting of υ4 (700) is characteristic of the aragonite structure [Citation9,Citation25]. In addition, υ2 (858) and υ1(1082) are also aragonite-specific peaks [Citation26]. While the single band at 711 cm−1 and the band of 875 cm−1 are characteristic of the calcite structure [Citation27]. Comparing to the FTIR spectrum of control calcite (upper panel of )), the rVDCP-induced crystals showed significant changes in the region of 1415 ~ 1642 cm−1, 1064 cm−1, and 564 ~ 669 cm−1 (lower panel of )), indicating the effects of rVDCP on calcite polymorph. The strong IR bands at 1619 and 1642 cm-1, which correspond to the amide I (C-O bond) and/or amide II (C-N bond) groups of the proteins [Citation28], suggested the binding of rVDCP to the crystals (lower panel of )). For the aragonite crystals, no changes in the polymorph were detected before and after rVDCP was added ()), indicating that rVDCP did not affect the polymorph of aragonite crystals.
Figure 7. SEM images of in vitro calcite crystallization in the presence of rVDCP at increasing concentrations. a: Calcite crystals grown without protein induction. b: Crystals grown with 50 μg/mL BSA induction. c: Crystals grown with 10 μg/mL rVDCP. d: crystals grown with 50 μg/mL rVDCP. e: Crystals grown with 100 μg/mL rVDCP. f: enlarged image of e.
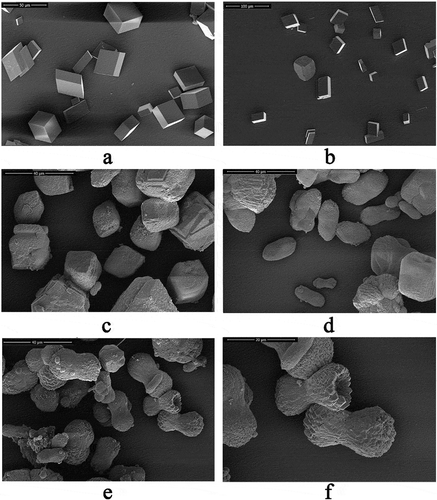
Figure 8. SEM images of in vitro aragonite crystallization in the presence of rVDCP at increasing concentrations. a: Aragonite crystals grown without protein induction. b: Aragonite crystals grown with 50 μg/mL BSA induction. c: Crystals grown with 10 μg/mL rVDCP. d: Crystals grown with 50 μg/mL rVDCP. e: Crystals grown with 100 μg/mL rVDCP. f: Enlarged image of e.
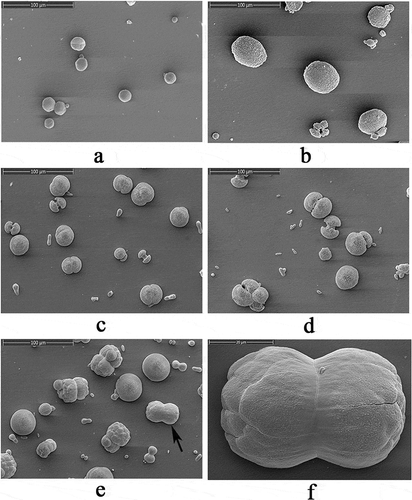
Figure 9. FTIR spectra of the rVDCP (100 μg/mL) induced crystals. a: The control (upper panel) and the rVDCP induced (lower panel) calcite crystals; b: the control (upper panel) and the rVDCP induced (lower panel) aragonite crystals. The regions of calcite polymorph variation induced by rVDCP were denoted by dash frames.
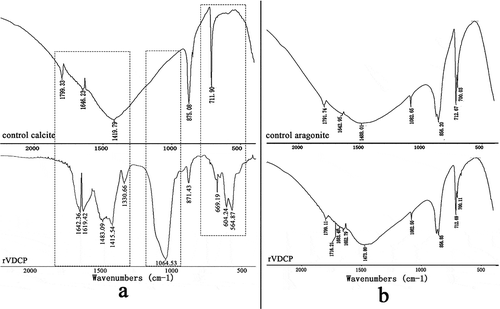
SDS-PAGE was used to detect the possible interactions between rVDCP and calcium carbonate crystals. In ), lanes 1, 2, and 3 represent the control rVDCP solution, the supernatant of the solution after rVDCP was precipitated with calcium carbonate crystals, and the rVDCP released from the precipitate of calcium carbonate crystals, respectively. For the calcite crystal binding experiment, the intensity of the protein band in lane 2 was much less than the intensity of the band in lane 1 ()), revealing the binding of rVDCP to the calcite crystals, which was further confirmed by the reappearance of the protein band in lane 3. For the aragonite crystal binding experiment, the band for the rVDCP protein was observed in both lanes 2 and 3 ()), suggesting much weaker binding of rVDCP to aragonite crystals than calcite crystals.
Figure 10. Binding ability of rVDCP with calcite (a) and aragonite (b), and the crystallization rate inhibition of rVDCP in calcite (c) and aragonite (d), respectively. Lane M, protein marker; lane 1, the control rVDCP; Lane 2, the supernatant of the solution after that the rVDCP was precipitated by calcium carbonate crystals; Lane 3, the rVDCP released from the precipitate of calcium carbonate crystals; The Data of c and d represent mean ± SD (n = 3). *, p < 0.05; **, p < 0.01.
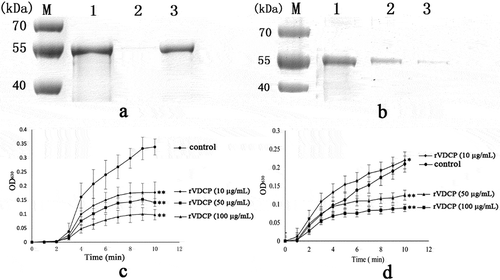
The crystallization rate of calcium carbonate was measured by monitoring the absorbance at 630 nm. As shown in ), rVDCP significantly reduced the crystallization rate of calcite crystals in a dose-dependent manner, and the highest absorbance value observed in the solution with 100 μg/mL of rVDCP was no more than 0.1 compared with a value of the control group of 0.35. Regarding the crystallization rate of aragonite, the rCLP exerted complex effects ()) characterized by inhibition at high concentration (50 and 100 μg/mL) and slight promotion at a low concentration (10 μg/mL).
Localization of VDCP in the shell and tissues of M. coruscus
Using the polyclonal anti-rVDCP antibody prepared in this study, the location of VDCP in the three shell layers was assessed using western blotting. Shell proteins were extracted from the nacre, myostracum, and fibrous prismatic layers of M. coruscus and divided into two parts, the acid-soluble and acid-insoluble fractions. As shown in ), VDCP was detected with the expected MW in both the acid-soluble and the acid-insoluble matrices from the myostracum layer. In addition, VDCP was also detected from the total proteins extracted from the mantle and the adductor muscle ()).
Figure 11. Western blot and immunohistochemistry analysis of VDCP. a: Western blot by anti-rVDCP antibody in shell matrices. Lane M, protein marker; Lane 1, insoluble fraction from the nacre layer; lane 2, insoluble fraction from the myostracum layer; lane 3, insoluble fraction from the fibrous prismatic layer; lane 4, soluble fraction from the nacre layer; lane 5, soluble fraction from the myostracum layer; lane 6, soluble fraction from the fibrous prismatic layer. b: Western blot by anti-rVDCP antibody (left panel) and anti-β-actin antibody (right panel), respectively, against total proteins extracted from the mantle (Ma) and the adduction muscle (AD). c: Immunohistochemistry analysis with the control group of adductor muscle performed using only the second antibody showed no significant signals; d: detection of VDCP in the adductor muscle and the positive signals are indicated by arrows. e: The control group of the mantle, showing the inner fold (IF) and the middle fold (MF); f: detection of VDCP in the mantle and the positive signal is indicated by arrows. g: The control group of the mantle, showing the middle fold (MF) and the outer fold (OF); h: detection of VDCP in the mantle and the positive signals are indicated by arrows. Scale bar, 50 μm.
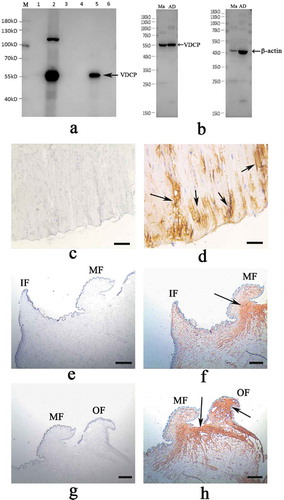
Immunohistochemistry further revealed that VDCP was mainly expressed in the muscle fibers close to the bottom of posterior adductor muscle, which connected with the shell surface at the shell muscle scar ()). In addition, VDCP was expressed in the mantle and produced strong signals in the outer fold and the middle fold ()), consistent with the results from the in situ hybridization experiment.
The microstructural location of VDCP on the shell surface was detected using immunofluorescence staining (). As shown in , the VDCP signal was mainly detected in the myostracum layer of the decalcified shell inner surface, and no signal was detected in the nacre and the fibrous prismatic layers or in deproteinized shell samples.
Figure 12. Immunofluorescence location of native VDCP on the inner surface of decalcified shell samples of M. coruscus. Images of decalcified the nacre (N), myostracum (M), and fibrous prism (FP) layer with anti-rVDCP polyclonal antibody as the first antibody, and Alexa Fluor 488-conjugated goat-anti-rabbit antibody as the second antibody. Images observed under white light and 488 nm, respectively. The positive signals were denoted by arrows. The deproteinized shell samples were used as negative controls. The scale bar, 100 μm.
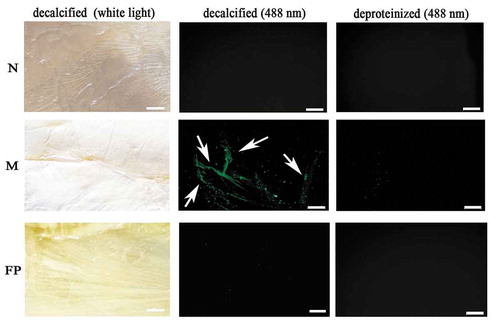
Identification of the protein interaction partners of VDCP in the shell matrices
Using Ni-coupled pull-down technology, the rVDCP protein with a His6 tag was used as a bait and the partner proteins were pulled down from the shell matrices. After a LC-MS/MS analysis, a total of 89 proteins identified with an FDR<0.01 (Supplementary Table 3) and 106 proteins were also identified from the negative control sample (Supplementary Table 4). After removing those nonspecific proteins, 8 proteins with more than 2 matching unique peptides are listed in , including VDCP itself.
Table 1. LC-MS/MS identification of the protein partners (with FDR<0.01 and more than 2 matched peptides) of the rVDCP in the shell matrices extracted from the shell of M. coruscus by affinity adsorption using Ni-column pull down.
Discussion
SMPs have been reported as the main organic components that play crucial roles in Mollusca shell formation. More than 1 000 SMPs with very different structures have been identified from many mollusk shells, and most of those proteins were identified using a proteomic approach. However, only a few individual SMPs have been characterized to date, because of the extremely small amounts of the matrix proteins in the shell and the difficulty in obtaining sufficient protein samples. In the present study, a recombinant shell protein with vWA domains was expressed, and the function and the location of this protein were analyzed to explore the possible mechanism of this protein in the shell formation.
VDCP contains two vWA domains (), a functional domain involved in molecular adhesion through protein interactions. vWA domains are present in various protein sequences, including collagens, copines, integrins, von Willebrand factor, complement factors B and C2, and matrilins [Citation29]. Of the vWA domain-containing proteins, collagen has been identified from various mollusk shells as a biomineralization-related matrix protein [Citation8,Citation11,Citation30–Citation35]. However, the roles of collagens in shell formation are not completely understood. The vWA domain is involved in protein–protein interaction and is common in structural proteins and extracellular matrix proteins [Citation29]. SMPs containing vWA domain had been reported as non-collagens and nacre-associated in previous works, including Pif (Pif 97 and Pif 80) and its homologues from Pinctada [Citation16,Citation36,Citation37], and BMSP (blue mussel shell protein) from Mytilus [Citation17] (Supplementary Figure 1). These results revealed the conservation of this domain in SMPs from different Bivalves and the important roles of vWA domain in shell formation. Furthermore, similar to the Pif protein [Citation16], VDCP of M. coruscus also contains a high proportion of charged amino acids (106, 23.4%) in its sequence (Supplementary Table 2). The charged amino acids had been reported to play important roles in biomineralization, either by forming a “calcium bridge” with calcium ions, or interacting with negatively charged carbonate ions [Citation21,Citation38–Citation40]. Homology searching identified VDCP with sequence similarity to collagens (Supplementary Table 1). Although VDCP showed low homology with Pif/BMSP (with sequence identity <15%, Supplementary Figure 2) due to the different sequence length and the low sequence similarity outside of the vWA domain, the phylogenetic analysis revealed the affiliation of VDCP with Pif and BMSP (), indicating that these proteins might work in a similar manner to regulate calcium carbonate crystallization.
The highest expression level of VDCP observed in the mantle indicates possible roles for VDCP in biomineralization, consistent with the expression of other SMPs. VDCP expression was further detected in the epithelial cells of the middle fold and the outer fold of mantle using in situ hybridization (), implying functions for VDCP in the prismatic layers [Citation41–Citation43]. In addition, the high expression level of the VDCP gene in the adductor muscle indicated a possible function for VDCP in this tissue. In situ hybridization confirmed the expression of VDCP at the bottom and the myofiber of the adductor muscle (). The localization of the VDCP gene in the mantle and adductor muscle was further confirmed by the results of the immunohistochemistry analysis using an anti-rVDCP antibody (). Moreover, VDCP was exclusively detected by western blot in the shell matrices extracted from the myostracum layer () and the immunofluorescence signal of VDCP was exclusively detected on the surface of the myostracum layer (). Our data confirmed a previous speculation that VDCP is a myostracum-specific shell protein, and suggested multiple functions for VDCP, such as in biomineralization, muscle-shell attachment, and muscle attraction.
In the present study, VDCP was successfully expressed using a codon optimization strategy and prokaryotic recombinant expression system. After isolation, renaturation, enterokinase digestion, and final purification, the rVDCP protein with a sequence very similar to the native VDCP protein was collected and the functional analyses were performed, including in vitro calcium carbonate crystal induction, binding, and inhibition of crystallization. The rVDCP altered the morphologies of both calcite and aragonite crystals (, ), altered the polymorph of calcite crystals (), bound to calcite crystals ()), and decreased the crystallization rate of calcite crystals ()). SMPs bind to the surface of calcium carbonate crystals and slow the growth of calcium carbonate crystals to finally promote the formation of crystals with different morphologies and polymorphs [Citation44]. This finding partially explains the effects of VDCP on calcite crystallization, but further studies are required to obtain additional details. Although VDCP weakly binds to aragonite crystal and exhibits a slight inhibition/promotion of the crystallization rate of aragonite crystals, calcite crystals may be the primary target of VDCP during shell formation. This speculation is based on the fact that the myostracum layer is completely composed of aragonite calcium carbonate crystals [Citation8], and on the hypotheses proposed in previous studies [Citation45,Citation46] that the formation of aragonite shell layers results from the transformation of the calcite crystals under the guidance of SMPs.
As highlighted previously, the assembly of a biochemical framework is essential for shell formation [Citation47]. In the present study, eight proteins were identified using the pull-down technique combined with LC-MS/MS analysis (), providing a set of candidate proteins that interact with VDCP (). Most of the interacting proteins had been identified from the shell proteome of M. coruscus [Citation8] or other mollusks [Citation11,Citation30–Citation32]. Among the identified proteins, shell mytilin (also named Mytilus uncharacterized shell protein, MUSP) is the top protein with the highest score. MUSP had been identified as a Mytilus-specific shell protein from M. galloprovincialis, M. edulis [Citation48], and M. coruscus shell [Citation8]. A homologue or recognized domain for MUSP has not been identified in the database. Thus, the role of MUSP in shell formation remains unclear. Although more detailed studies are necessary to explore the real interactions between VDCP and the identified proteins, the results of the VDCP pull-down assay provide a clue for studies of the shell protein–protein interactions aiming to understand the supramolecular chemistry that contributes to shell formation.
In summary, VDCP is a novel SMP with vWA domain, and the rVDCP affected the morphology, polymorph, and crystallization rate of calcium carbonate crystals. The specific localization of VDCP in the mantle, adductor muscle, and the shell inner surface implied functions for this protein in biomineralization and muscle-shell attachment. We are fully aware that the functional analysis of a single shell matrix protein is not sufficient to explain the whole process of shell fabrication. However, we consider that the characterization of individual biomineralization-related proteins will identify the complete biochemical framework required to precisely analyze the formation of the shell.
Author contributions
Qi Sun and Yuting Jiang conducted experiments and analyzed data. Meihua Fan and Zhi Liao conceived and designed the research. Xiaolin Zhang collected the mussel and conducted the PCR analysis. Huanzhi Xu conducted the FTIR analysis. Zhi Liao and Qi Sun wrote the manuscript. All authors have read and approved this manuscript.
Supplementary_Table_4_YT.docx
Download MS Word (41.1 KB)Supplementary_Table_3_YT.docx
Download MS Word (37.8 KB)Supplementary_Table_2_YT.docx
Download MS Word (16.5 KB)Supplementary_Table_1_YT.docx
Download MS Word (22.3 KB)Supplementary_Figure_2.pdf
Download PDF (1.8 MB)Supplementary_Figure_1.pdf
Download PDF (237.6 KB)Acknowledgments
Thanks Dr Dandan Song of Zhejiang University for SEM analysis. This work was supported by National Natural Science Fund of China under Grant No. 31671009; The Project of Zhoushan Science and Technology Bureau under Grant No. 2019F12004.
Disclosure statement
No potential conflict of interest was reported by the authors.
Supplementary material
Supplemental data for this article can be accessed here.
Additional information
Funding
References
- Li XW, Ji HM, Yang W, et al. Mechanical properties of crossed-lamellar structures in biological shells: a review. J Mech Behav Biomed Mater. 2017;74:54–71.
- Barthelat F. Nacre from mollusk shells: a model for high-performance structural materials. Bioinspir Biomim. 2010;5(3):035001.
- Wählisch FC, Peter NJ, Abad OT, et al. Surviving the surf: the tribomechanical properties of the periostracum of Mytilus sp. Acta Biomater. 2014;10(9):3978–3985.
- De Paula SM, Silveira M. Studies on molluscan shells: contributions from microscopic and analytical methods. Micron. 2009;40:669–690.
- Arivalagan J, Yarra T, Marie B, et al. Insights from the shell proteome: biomineralization to adaptation. Mol Biol Evol. 2017;34(1):66–77.
- Kocot KM, Aguilera F, McDougall C, et al. Sea shell diversity and rapidly evolving secretomes: insights into the evolution of biomineralization. Front Zool. 2016;13:23.
- Meenakshi VR, Blackwelder PL, Hare PE, et al. Studies on shell regeneration. I. Matrix and mineral composition of the normal and regenerated shell of Pomacea paludosa. Comp Biochem Physiol A Comp Physiol. 1975;50(2):347–351.
- Zhi L, Linfei B, Meihua F, et al. In-depth proteomic analysis of nacre, prism, and myostracum of Mytilus shell. J Proteomics. 2015;122:26–40.
- Lee SW, Jang YN, Kim JC. Characteristics of the aragonitic layer in adult Oyster shells, Crassostrea gigas: structural study of myostracum including the adductor muscle scar. Evid Based Complement Alternat Med. 2011;2011:742963.
- Liu X, Pu J, Zeng S, et al. Hyriopsis cumingii Hic52-A novel nacreous layer matrix protein with a collagen-like structure. Int J Biol Macromol. 2017;102:667–673.
- Du X, Fan G, Jiao Y, et al. The pearl oyster Pinctada fucata martensii genome and multi-omic analyses provide insights into biomineralization. Gigascience. 2017;6(8):1–12.
- Nudelman F, Lausch AJ, Sommerdijk NA, et al. In vitro models of collagen biomineralization. J Struct Biol. 2013;183(2):258–269.
- Saito T, Arsenault AL, Yamauchi M, et al. Mineral induction by immobilized phosphoproteins. Bone. 1997;21(4):305–311.
- Fratzl P, Fratzl-Zelman N, Klaushofer K. Collagen packing and mineralization. An x-ray scattering investigation of turkey leg tendon. Biophys J. 1993;64(1):260–266.
- Fratzl P, Paris O, Klaushofer K, et al. Bone mineralization in an osteogenesis imperfecta mouse model studied by small-angle X-ray scattering. J Clin Invest. 1996;97(2):396–402.
- Suzuki M, Saruwatari K, Kogure T, et al. An acidic matrix protein, Pif, is a key macromolecule for nacre formation. Science. 2009;325(5946):1388–1390.
- Suzuki M, Iwashima A, Tsutsui N, et al. Identification and characterisation of a calcium carbonate-binding protein, blue mussel shell protein (BMSP), from the nacreous layer. Chembiochem. 2011;12(16):2478–2487.
- Du YP, Chang HH, Yang SY, et al. Study of binding interaction between Pif80 protein fragment and aragonite. Sci Rep. 2016;6:30883.
- Livak KJ, Schmittgen TD. Analysis of relative gene expression data using real-time quantitative PCR and the 2(T) (−Delta Delta C) method. Methods. 2001;25:402–408.
- Varnerin JP, Chung CC, Patel SB, et al. Expression, refolding, and purification of recombinant human phosphodiesterase 3B: definition of the N-terminus of the catalytic core. Protein Expr Purif. 2004;35(2):225–236.
- Liang J, Xu G, Xie J, et al. Dual roles of the lysine-rich matrix protein (KRMP)-3 in shell formation of pearl oyster, Pinctada fucata. PLoS One. 2015;10(7):e0131868.
- Yan Z, Jing G, Gong N, et al. N40, a novel nonacidic matrix protein from pearl oyster nacre, facilitates nucleation of aragonite in vitro. Biomacromolecules. 2007;8(11):3597–3601.
- Kong J, Liu C, Wang T, et al. Cloning, characterization and functional analysis of an alveoline-like protein in the shell of Pinctada fucata. Sci Rep. 2018;8(1):12258.
- Rossmann MG, Moras D, Olsen KW. Chemical and biological evolution of a nucleotide- binding protein. Nature. 1974;250(463):194–199.
- Balmain J, Hannoyer B, Lopez E. Fourier transform infrared spectroscopy (FTIR) and X-Ray diffraction analyses of mineral and organic matrix during heating of mother of pearl (nacre) from the shell of the mollusc Pinctada maxima. J Biomed Mater Res. 1999;48(5):749–754.
- Sabbides TG, Koutsoukos PG. The crystallization of calcium carbonate in artificial seawater; role of the substrate. J Cryst Growth. 1993;133:13–22.
- Ross SD. Inorganic infrared and raman spectra. London: McGraw–Hill; 1972.
- Dauphin Y, Marin F. The compositional analysis of recent cephalopod shell carbohydrates by Fourier transform infrared spectrometry amperometric detection. Experentia. 1995;51:278–283.
- Whittaker CA, Hynes RO. Distribution and evolution of von Willebrand/integrin a domains: widely dispersed domains with roles in cell adhesion and elsewhere. Mol Biol Cell. 2002;13(10):3369–3387.
- Gao P, Liao Z, Wang XX, et al. Layer-by-layer proteomic analysis of mytilus galloprovincialis shell. PLoS One. 2015;10(7):e0133913.
- Zhang G, Fang X, Guo X, et al. The oyster genome reveals stress adaptation and complexity of shell formation. Nature. 2012;490(7418):49–54.
- Marie B, Arivalagan J, Mathéron L, et al. Deep conservation of bivalve nacre proteins highlighted by shell matrix proteomics of the Unionoida, Elliptio complanata, and Villosa lienosa. J R Soc Interface. 2017;14(126):pii:20160846.
- Liu X, Zeng S, Dong S, et al. A novel matrix protein Hic31 from the prismatic layer of hyriopsis cumingii displays a collagen-like structure. PLoS One. 2015;10(8):e0135123.
- Sleight VA, Thorne MA, Peck LS, et al. Characterization of the mantle transcriptome and biomineralisation genes in the blunt-gaper clam, Mya truncate. Mar Genomics. 2016;27:47–55.
- Arivalagan J, Marie B, Sleight VA, et al. Shell matrix proteins of the clam, Mya truncata: roles beyond shell formation through proteomic study. Mar Genomics. 2016;27:69–74.
- Joubert C, Piquemal D, Marie B, et al. Transcriptome and proteome analysis of Pinctada margaritifera calcifying mantle and shell: focus on biomineralization. BMC Genomics. 2010;11:613–625.
- Berland S, Marie A, Duplat D, et al. Coupling proteomics and transcriptomics for the identification of novel and variant forms of mollusk shell proteins: a study with P. margaritifera. Chembiochem. 2011;12:950–961.
- Addadi L, Weiner S. Interactions between acidic proteins and crystals: stereochemical requirements in biomineralization. Proc Natl Acad Sci U S A. 1985;82(12):4110–4114.
- Addadi L, Weiner S. Interactions between acidic macromolecules and structured crystal surfaces. Stereochemistry and biomineralization. Mol Cryst Liq Cryst. 1986;134(1):305–322.
- Shen X, Belcher AM, Hansma PK, et al. Molecular cloning and characterization of lustrin A, a matrix protein from shell and pearl nacre of haliotis rufescens. J Biol Chem. 1997;272(51):32472–32481.
- Sudo S. Structure of mollusc shell framework proteins. Nature. 1997;387(6633):563–564.
- Takeuchi T, Biphasic EK. Dually coordinated expression of the genes encoding major shell matrix proteins in the pearl OysterPinctada fucata. Mar Biotechnol. 2006;8(1):52–61.
- Joubert C, Piquemal D, Marie B, et al. Transcriptome and proteome analysis of Pinctada margaritiferacalcifying mantle and shell: focus on biomineralization. Bmc Genomics. 2010;11(1):613–620.
- Xue Z, Shen Q, Liang L, et al. Mechanism of SCA-1 on a calcite surface: a molecular dynamics study. Langmuir. 2017;33(42):11321–11331.
- Carter JG, Clark GR II. Classification and phylogenetic significance of molluscan shell microstructure. In: Bottjer DJ, Hickman CS, Ward PD, et al., editors. Molluscs, notes for a short course. Tennessee: University of Tennessee; 1985. p. 50–71.
- Taylor J, Kennedy WJ, Hall A. The shell structure and mineralogy of the Bivalvia, Part II. Lucinacea-Clavagellacea conclusions. Bull Br Mus Nat Hist. 1973;22:253–294.
- Nudelman F, Gotliv B, Addadi L, et al. Mollusk shell formation: mapping the distribution of organic matrix components underlying a single aragonitic tablet in nacre. J Struct Biol. 2006;153:176–187.
- Marie B, Le Roy N, Zanella-Cléon I, et al. Molecular evolution of mollusc shell proteins: insights from proteomic analysis of the edible mussel Mytilus. J Mol Evol. 2011;72(51–6):531–546.