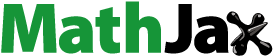
Abstract
Nanoparticles (NP) of 12.7 nm in diameter of the poly(methyl methacrylate (MMA)-co-methacrylic acid (MAA)) copolymer were prepared. 13C-NMR results showed a MMA:MAA molar ratio of 0.64:0.36 in the copolymer, which is similar to the poly(MMA-co-MAA) commercially known as the FDA approved Eudragit S100 (0.67:0.33). The NP prepared in this study were loaded at pH 5 with varying amounts (from 0.54 to 6.91%) of doxorubicin (DOX), an antineoplastic drug. 1H-NMR results indicated the electrostatic interactions between the ionized carboxylic groups of the MAA units in the copolymer and the proton of the glycosidic amine in DOX. Measurements by QLS and TEM indicated that the loading destabilizes the NP, and that for increase stability, they aggregate in a reversible way, forming aggregates with a diameter up to 99.5 nm at a DOX load of 6.91%. The analysis of drug release data at pH 7.4 showed that loaded NP with at least 4.38% DOX release the drug very slowly and follows the Higuchi model; the former suggests that they could remain for long periods in the bloodstream to reach and destroy cancer cells.
1. Introduction
DOX is used as a first-line treatment for a wide spectrum of cancers, such as: breast, ovarian, testicles, lung, bladder, and stomach; also, it is used for clinical treatments such as: lymphomas, acute leukemia, solid tumors, Kaposi's sarcoma and soft tissue sarcoma [Citation1, Citation2]. One of the main problems with this anticancer agent is that it does not specifically target cancer cells also affecting healthy tissue, causing toxicity and side effects that limit its maximum tolerable dose [Citation2–5]. To avoid these complications, an attempt has been made to load DOX into various nanostructures, some of them made of polymers [Citation6–19], trying to improve its pharmacokinetics and pharmacodynamics in order to achieve greater efficiency in cancer treatment with a minimum of adverse side effects. Bellow we present a brief analysis of some of the most important reports on the preparation of DOX-loaded polymeric NP.
In 2001, Janes et al. [Citation6] reported the formation of chitosan NP loaded with DOX, despite the cationic characteristic of both materials. These NP showed a mean diameter between 250 and 300 nm with a 4% DOX content. The cytotoxicity study indicated that there was no great difference between that of the free DOX and that loaded on the NP. In 2007, Tian et al. [Citation7] published the preparation of a polymeric chain composed of poly(acrylic acid) (PAA) attached on Pluronic P85, which was loaded with DOX through the electrostatic interactions between the cationic groups of this drug and the anions from ionized acidic groups of PAA. Once loaded with DOX at pH 7.2 the chains assembled forming NP with diameters from100 to 200 nm. Furthermore, an accelerated release of DOX at pH 5 was observed, which could be advantageous if the drug is to be released into the lysosomes of cancer cells. Another interesting report is the one published by Betancourt et al. [Citation8] These authors loaded DOX in NP of poly(lactic-co-glycolic) acid to obtain nanostructures with 230 nm in mean diameter and up to 5% drug content. These NP showed similar cytotoxicity to that of free DOX on MDA-MB-231 cancer cells. The publication of Du et al. [Citation9] is also worth mentioning. They reported the preparation of pH responsive DOX-loaded NP of a polymersome containing a block of PAA. According to this report a drug loading content of 15.9% was achieved with a relatively small mean diameter of 64 nm. Interestingly, these nanostructures exhibited high anti-tumor activity, similar to that of free DOX. Shixian et al. [Citation10] reported the preparation of NP made of an amphiphilic anionic copolymer, containing up to 21.7% DOX and a mean diameter of 140 nm. Evaluated in preclinical studies in mice model, these loaded NP showed significant antitumor activity, reduced side effects, and an enhanced tumor accumulation as a result of their prolonged circulation in blood. More recently, the obtention of a pH sensitive DOX-loaded polyurethane NP was reported [Citation13]. The mean diameter of the loaded NP was around 180 nm with a DOX content of 14.1%. According to the authors, these nanostructures showed a higher level of cellular internalization and higher inhibitory effects on the proliferation of MCF-7 cancer cells than that of pure DOX. Interestingly, this last result would imply a synergy between DOX and NP, nevertheless, it was not discussed by the authors.
According to the information presented above, it is common to prepare NP made from polymers approved for use in humans, with DOX loadings varying from a few percentage units to more than 50% and diameters ranging from a little more than 60 to 300 nm. With these characteristics, in general, it is possible to say that DOX-loaded polymeric NP would show greater cytotoxicity than DOX. However, the scarce interest in the loading of DOX in NP, with diameters less than 50 nm, made with polymers approved by the FDA is remarkable. This is surprising as it is known that NP smaller than 50 nm in diameter would reduce the risk of being detected by the immunological system [Citation20, Citation21] and nanostructures larger than 100 nm are more rapidly removed from the blood stream by the immunological systems as well as the liver and the spleen [Citation22, Citation23]. Trying to create options in this area of opportunity, our group undertook the study of preparation of sub-50 nm NP, loaded with DOX, by using an FDA approved copolymer. This material is the poly(methyl methacrylate (MMA)-co-methacrylic acid (MAA)) copolymer or P(MMA-co-MAA), in a MMA:MAA molar ratio of 0.64:0.36, which is similar to Eudragit S100 (ES 100), a polymer widely used in the elaboration of drug-loaded pills due to its water solubility at pH >7 [Citation24]. Although the preparation of ES 100 NP with mean diameters ranging from 50 to 700 nm, loaded with different drugs, have been documented [Citation25–31], obtaining NP from a copolymer similar to ES 100 loaded with drugs and with diameters less than 50 nm has only been reported by our group [Citation32, Citation33].
It is reported herein the preparation of P(MMA-co-MAA) in a MMA:MAA molar ratio of 0.64:0.36 NP using a method named semicontinuous heterophase polymerization (SCHP) developed by us [Citation34]. Loading of NP with DOX and the corresponding release study at pH 7.4 are also included.
2. Materials and methods
2.1. Materials
Sodium dodecyl sulfate (SDS) (98.5%), sodium bis (2-ethylhexil) sulfosuccinate (AOT) (96%) and ammonium persulfate (APS) (99%) from Sigma-Aldrich were used as received. MMA and MAA, also from Sigma-Aldrich, were distilled under reduced pressure and stored at 4 °C. Doxorubicin hydrochloride (99.47%) from MedChemExpress was used as received. Tetrahydrofuran (THF, HPLC grade), methanol (anhydrous), (trimethylsilyl)diazomethane (2.0 M in hexane) and phosphate buffered saline (PBS) were purchased from Sigma-Aldrich and used as received. De-ionized and triple-distilled (ultra-pure) water with conductivity less than 6 µS/cm was used.
2.2. Methods
2.2.1. Polymerization
The P(MMA-co-MAA) NP were prepared by means of SCHP using SDS and AOT as surfactants and APS as initiator, following a similar recipe and conditions than those of our previous report [Citation34].
2.2.2. Drug loading
The latex obtained through SCHP was dialyzed for removing the surfactants as well as residual monomers. Then, a dispersion containing 1 g of NP in 20 g of de-ionized water (pH adjusted to 5) in a round-bottom glass flask and a solution containing the DOX hydrochloride in 20 g of de-ionized water in a glass vial were prepared. To proceed with the loading the drug solution was added to the NP dispersion by means of a peristaltic pump at a flow rate of 0.07 mL/min until the dispersion being added is depleted, while maintaining vigorous agitation during the 12 h that the process lasts. The experiments, with different percentage loads in weight of DOX hydrochloride with respect to NP (0.6, 1.2, 1.8, 2.4, 3.0, 5.0 and 10.0) were carried out by duplicate in the dark to avoid the drug photo-degradation. A part of the resulting latex was lyophilized to be fully characterized.
Samples were subjected to an ultracentrifugation step (50,000 rpm during 3 h) in order to estimate the content of DOX incorporated in the polymeric NP by means of analysing the supernatant in an UV-vis equipment and comparing the absorbance with a calibration curve of the pure DOX. Drug loading content (DLC) and drug loading efficiency (DLE) were calculated following EquationEquations 1(1)
(1) and Equation2
(2)
(2) , respectively:
(1)
(1)
(2)
(2)
where WDOXo is the DOX weight that was added in each formulation, WDOXsup is the DOX weight that was obtained in the supernatant and WNP in the weight of NP in the loading system.
2.2.3. Drug release
DOX release tests in the loaded systems were done in triplicate following the methodology reported by Diaz Duarte-Rodriguez et al. [Citation35]; 10 mg of DOX loaded NP were dispersed in 3 mL of PBS solution (pH 7.4) employing an ultrasound bath for 1 h. Then the dispersion was added to a dialysis tube (Spectra/Por® MWCO: 3.5 kDa, diameter 11.5 mm, from Spectrum Chemical). The dialysis tube was introduced into 50 mL of release medium inside an amber glass bottle. The bottle was placed inside a shaking bath operating at 37 °C and at a shaking speed of 70 cycles per minute. 3 mL aliquots of the medium were taken out at different times and replaced by 3 mL of fresh PBS at every sampling point. The released fraction of DOX was calculated from UV measurements at λmax = 483 nm and then quantified by using a calibration curve of DOX in PBS.
2.2.4. Characterization
2.2.4.1. Quasielastic light scattering (QLS)
Measurements by QLS in a Malvern Zetasizer Nano-ZS90 apparatus at 25 °C were carried out to determine the number-average diameter (Dn) of the latexes before and after the loading process. To eliminate multiple scattering and particle interactions, the latexes samples were diluted 50 times with water.
2.2.4.2. Zeta potential
This property was measured in a Zeta-Check Particle Charge Reader (Microtrac) at room temperature.
2.2.4.3. Gel permeation chromatography (GPC)
The dialyzed latex was subjected to three freezing-and-thaw cycles to coagulate the copolymer, which was then filtered, washed with de-ionized water and dried. The copolymer was dissolved in a mixture of tetrahydrofuran/methanol (50/50 v/v) to be treated with a few drops of (trimethylsilyl)diazomethane solution in hexane to methylate the MAA units of the copolymer. The dried methylated copolymer was dissolved in THF and analysed in a Hewlett-Packard instrument (HPLC series 1100) equipped with a refractive index detector. A set of three PLGel columns connected in series was used. Calibration was carried out with poly(methyl methacrylate) standards (from Agilent) and THF was used as eluent at a flow rate of 1 mL/min.
2.2.4.4. Nuclear magnetic resonance (NMR)
The unloaded copolymer NP was analysed by 13C-NMR (12,000 scans) in a Bruker-400 MHz spectrometer in order to calculate the copolymer molar composition. On the other hand, the loaded copolymer NP were analysed by 1H-NMR (16 scans) in the same equipment in order to demonstrate the presence of DOX in the loaded systems. A mixture of deuterated chloroform (99.8 atom % D from Sigma-Aldrich) and deuterated trifluoroacetic acid (99.5 atom % D from Sigma-Aldrich) (50/50 v/v) was used as solvent and the analyses were performed a room temperature.
2.2.4.5. Transmission electron microscopy (TEM)
The NP diameters were also observed by TEM in a transmission electron microscope Titan-300 kV for which samples were prepared by dispersing on drop of the latex containing the loaded NP in 50 g of de-ionized water. Then a drop of the resulting dispersion was deposited on a copper grid (Lacey Carbon 300 mesh) and analysed after water evaporation.
2.2.4.6. Ultraviolet-visible spectroscopy (UV-vis)
A spectrometer UV-visible Varian Cary 100 (Agilent Technologies) was used to determine the DOX content in the supernatant obtained in the loading experiments. For this, a calibration curve was elaborated ranging from 10 to 150 ppm in DOX concentration by dissolving the required quantities of the drug in de-ionized water and reading the absorbance at λmax = 483 nm. UV-vis DOX determination in the PBS used in the release study was carried out in the UV-vis equipment mentioned before. The corresponding calibration curve was made using a PBS solution, 0.1 M, at pH = 7.4 and in a DOX concentration range of 3 to 100 ppm. All UV-vis tests were performed at room temperature.
3. Results and discussion
3.1 P(MMA-co-MAA) NP
3.1.1. Characterization
3.1.1.1. Particle size
The size distribution of the particles obtained in the polymerization, measured by QLS, is shown in . The Dn value determined by this method is 12.7 nm, which matches very well with the sizes previously reported using SCHP under similar recipe and conditions [Citation32–34].
3.1.1.2. Molecular weight
shows the molecular weight distribution of the copolymer prepared in this study. The number- (Mn) and weight- (Mw) average molecular weights were 2.3 × 105 and 5.1 × 105 g/mol, respectively; this indicates that the molecular weight of the copolymer obtained in our study is much greater than that of ES 100, whose Mn and Mw value were reported as 2.4 × 104 and 4.0 × 104 g/mol, respectively [Citation33].
3.1.1.3. Molar composition
A 13C-NMR analysis was carried out to the copolymer that forms the NP; the corresponding carbonyl region of the spectrum is shown in . From this spectrum, the MMA/MAA molar ratio in the copolymer was calculated using the integration of the signals corresponding to the carbonyl groups, which are approximately located at 180 ppm for the MMA and at 184 ppm for the MAA. The MMA:MAA molar ratio thus obtained for the copolymer was 0.64:0.36, which is close to the one reported for ES 100 (0.67:0.33). The P(MMA-co-MAA) obtained in this study has a slightly higher content of MAA units than those contained in the ES100, suggesting that the chains in the copolymer reported here would be more quickly solubilized in organic fluids at pH higher than 7, as compared to those forming the commercial material. However, the higher molecular weight of the P(MMA-co-MAA) could counteract this expected behavior.
3.2. DOX loading in copolymer NP
3.2.1. DOX loading
and show the DOX loading results of the NP prepared in this study. In general, the DLE values are high, although the relatively low value reached with the highest DOX load is notable. Nevertheless, in accordance with the measurements by QLS, the most interesting result is the great increase in the Dn values as the drug load increases, which can be seen in . A similar behavior was found by our group in the study of preparation of aspirin-loaded copolymer NP [Citation33]. This was explained as a result of the coating of the NP surface with aspirin molecules, which does not provide sufficient protection against aggregation as that given by the anions of the carboxyl groups of MAA.
Figure 4. Variation of Dn measured by QLS of loaded nanostructures with different concentrations of DOX hydrochloride used in the loading experiments.
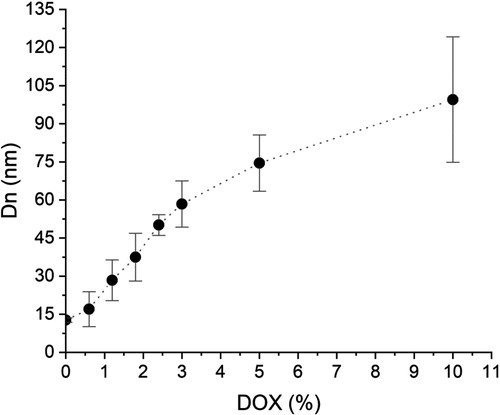
Table 1. Results obtained in the loading experiments.
In the NP loading experiment with 0.6% DOX hydrochloride, a DLC of 0.54% was obtained, but Dn grew up from 12.7 to 17 nm, according to QLS measurement. This augment in size gives an increase ratio in Dn of 1.34, which corresponds to a volume ratio of 2.41. Assuming, for simplicity, that the copolymer and DOX have similar densities, we would have a weight ratio of loaded to non-loaded NP equal to 2.41. A simple calculation shows that in order to attain a 17 nm NP diameter, the DOX-loaded NP should contain about 58.5% by weight drug. Similar calculations for the other loading experiments yield equally unrealistic DOX contents in the loaded NP. To stress the point, consider the results of the loading experiment with 10% DOX hydrochloride. In this case, the Dn obtained by QLS shows a value of 99.5 nm, corresponding to the size of NP containing 99.8% DOX, which is a much higher value than the 6.91% experimentally obtained. Confirming this interpretation of the results, shows a micrograph of DOX-loaded NP from the loading experiment with 10% DOX hydrochloride showing that these loaded NP roughly retain a much smaller than 20 nm diameter. Indeed, in accordance with the corresponding calculations, the Dn value for NP containing 6.91% DOX should be about 13 nm.
It is suggested from the previous discussion, that the presence of DOX causes instability to loaded NP leading to their reversible aggregation. For example, the 17 nm nanostructure diameter obtained from the loading experiment with 0.6% DOX hydrochloride, could result from the aggregation of 2 to 3 12.7 nm loaded NP. On the other hand, the result obtained from the loading experiment with 10% DOX hydrochloride can be explained by the aggregation of about 480 loaded NP of 12.7 nm diameter, which would give rise to the 99.5 nm diameter NP observed. This behavior could be explained taking into account that only a fraction of the carboxyl groups in the MAA units contained in the copolymer forming a nanoparticle is close to their surface. Moreover, due to the fact that the value of pKa is 6 for the carboxyl groups in MAA [Citation36] as well as that the drug loading was carried out at pH 5, less than 50% of the carboxyl groups close to the NP surface will be ionized. This is very important, because it is believed that the anions resulting from carboxyl groups ionization give stability to the copolymer NP after dialysis [Citation33]. In this way, if some of these anions are neutralized as a consequence of their electrostatic interaction with the ionized glycosidic amine in DOX [Citation6] (Scheme 1), the loaded NP will become unstable.
Scheme 1. Representation of electrostatic interaction between the ionized carboxylic group in copolymer and the ionized glycosidic amine in DOX.
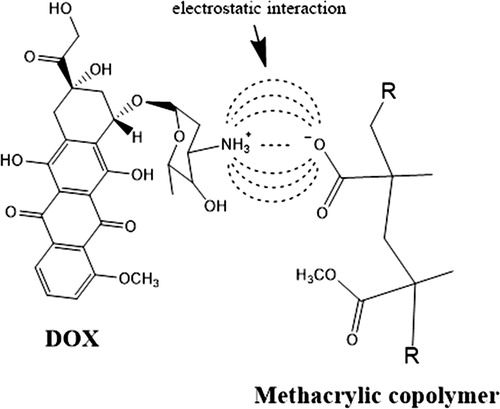
According to , the instability of the NP became more intense as the DOX load increased. NP would adsorb DOX on their surface until they became unstable, then they would group with other loaded NP to reduce their area/volume ratio and become more stable, but as DOX is available in the medium, the loaded NP aggregates would continue to adsorb drug to become unstable again, grouping with other loaded NP or loaded NP aggregates to re-stabilize. As long as DOX is available in the aqueous dispersion this cycle would repeat. At the end of the loading process, there would be aggregates composed of DOX-loaded NP (Scheme 2).
As confirmation that the DOX adsorbs on the surface of the NP causing a decrease in their stability, the zeta potential of the NP was measured before and after being loaded in the loading experiment with 5% DOX hydrochloride. Before the drug loading, the NP showed a zeta potential value of −35.8 mV at pH 5, while the DOX-loaded NP exhibited a value of −25 mV at the same pH. As is well known, a decrease in the absolute value of the zeta potential of a colloidal dispersion is indicative of a decrease in its stability.
3.2.2. Chemical characterization of loaded NP
In the δ interval between 0.5 and 2 ppm of the 1H-NMR spectra shown in , the signals corresponding to backbone chains of the P(MMA-co-MAA) copolymer can be seen. Likewise, in the range from 6 to 8.4 ppm, the characteristic signals of aromatic protons present in the DOX molecule are identified; this range is amplified in for a better observation of the signals at 8.1, 7.9 and 7.5 ppm, which correspond to protons that are part of the aromatic ring of the drug [Citation37, Citation38]. When comparing the spectra of the loading experiments with 3, 5 and 10% DOX hydrochloride as a whole, the expected increase in the intensity of these signals was evident, as well as the disappearance of the broad signal close to 8 ppm (top right in ), corresponding to the proton of glycosidic amine (NH3+) [Citation1]. The absence of the broad signal at 8 ppm suggests the occurrence of electrostatic interactions between the ionized carboxylic groups of the copolymer and the proton of the glycosidic amine, affecting its appearance in the various spectra due to the electron density of the neighboring groups. This effect could lead to a small shift in the signals of the H-1 and H-2 protons that are part of the first aromatic ring of anthracycline group, as shown in that includes the DOX structure with the protons numbering. Taking into account the absence of water as a diffusion medium within the NP and as a consequence the difficulty of DOX molecules to diffuse into the NP, the aforementioned electrostatic interactions can only occur involving the carboxylic groups close to the surface of the NP. On the other hand, the presence of singlets at 6.7 and 6.5 ppm could be attributed to the displacement of other signals, such as the protons of OH groups positioned in the aromatic rings [Citation39].
Figure 6. 1H-NMR spectra of polymeric material forming the loaded NP from the loading experiments with, 3, 5 and 10% DOX hydrochloride.
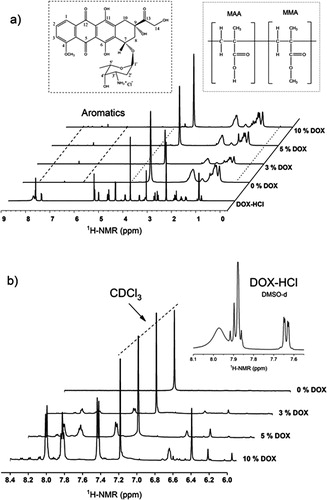
Table 2. Signals displacements in the NMR spectra of P(MMA-co-MAA) NP loaded with DOX hydrochloride.
3.3. DOX release study
3.3.1. Release profiles
The DOX release profiles of the loaded NP obtained in the loading experiments with 3, 5 and 10% drug are shown in . From it can be seen that the load of DOX in NP achieved in these experiments were 2.75, 4.38 and 6.91%, respectively. also shows how the loaded NP from the loading experiment with 3% DOX hydrochloride, released in the first 9 h about 60% of their total load, while, those from the loading experiments with 5 and 10% drug, released in the same first 9 h about 14 and 9% of their total load, respectively. At the end of the release time considered in the study it was found that only about 70, 30 and 20% of total drug in the loaded NP from the experiments with 3, 5 and 10% DOX hydrochloride, respectively were released. These results clearly indicate that DOX is released much more slowly from the loaded NP prepared in the loading experiments with 5 and 10% compared to those from the experiment with 3% drug.
Figure 7. DOX release profiles from the nanostructures obtained in the loading experiments with different DOX hydrochloride percentages.
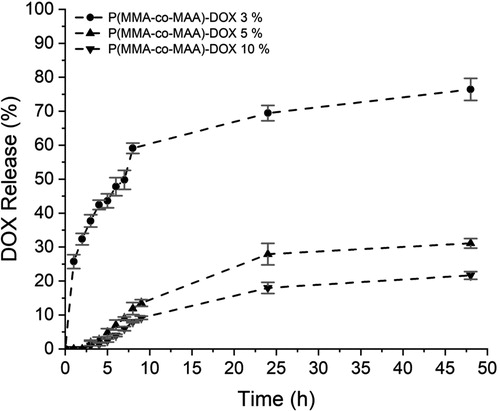
Moreover, in previous studies on the release of free DOX at pH 7.4 it was reported that above 70 and 80% of this drug was released in the first 6 [12] and 7.5 h [Citation13], respectively, which is slightly faster than that observed in the release profile of the loaded NP from the loading experiment with 3% DOX hydrochloride.
A possible explanation for this behavior is based on the fact that DOX remains on the surface of the NP as result of the electrostatic interaction between the anions of the carboxyl groups of the MAA units, and the cations of the glycosidic amine in DOX. Then, when the loaded NP aggregate to increase their stability, a fraction of the DOX is confined within the aggregates. Due to the decrease in the area/volume ratio, this fraction is higher as the size of the aggregates is bigger. Since the DOX on the surface of the aggregates is released first and then, more slowly, the drug adsorbed on the surface of the NP inside the aggregates, the loaded NP from the experiment with 3% DOX release a greater proportion of their load in the first 9 h.
3.3.2. Release models
In order to investigate the DOX release mechanism from the loaded NP a series of models were tested. The release experimental data were fitted to straight lines in accordance with the linearized equations of the Zero order, First order, Higuchi and Korsmeyer–Peppas models [Citation40], which can be seen in . The results of these fits, shown in , indicate that the data from the experiment with 3% DOX hydrochloride fit the Korsmeyer-Peppas model very well, while those from experiments with 5 and 10% drug fit very well the Higuchi model. In accordance to literature [Citation40] Higuchi and Korsmeyer-Peppas models are represented by Equations 3 and 4, respectively:
(3)
(3)
where Q is the cumulative % drug released at time t and kH is the dissolution constant.
(4)
(4)
where C is the cumulative % drug release at time t, K is the rate constant and n is the release exponent, which characterizes different release mechanisms.
Figure 8. Release models for DOX released from the nanostructures obtained in the loading experiments with 3 (●), 5 (▲) and (10 (▼) % DOX hydrochloride. Zero order (a), first order (b), Higuchi (c) and Korsmeyer–Peppas (d).
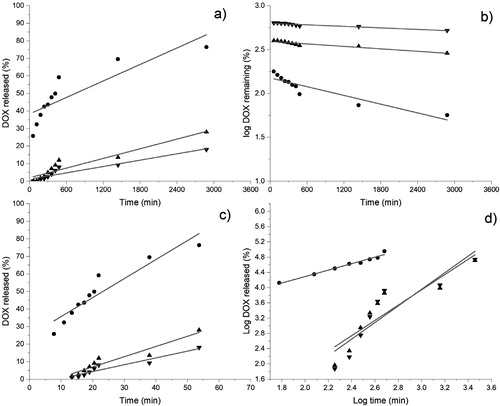
Table 3. R2 values obtained from the fit of release experimental data to different release models.
The fact that DOX is released from the loaded NP according to Korsmeyer-Peppas model indicates that the release mechanism is not a fickian type, which is confirmed by the value of 0.7 for n [Citation40]. The explanation is that the dissolution of copolymer chains affects the diffusion of the DOX molecules, due to the aggregates obtained in the experiment with 3% DOX hydrochloride are composed of 97 NP, value obtained following the same calculation procedure as in Section 3.2.1. In this case, a great fraction of the DOX molecules would be on the surface of the aggregates, whose release would be influenced by the dissolution of the chains in the NP forming the exterior of the aggregates. On the other hand, the DOX released from loaded NP prepared in the experiments with 5 and 10% DOX hydrochloride followed the Higuchi model, which implies a fickian type diffusion mechanism. This means that the effect of the copolymer chains dissolution on the DOX molecules diffusion would be negligible. This consideration is understandable, taking into account that the copolymer chains in the NP on the aggregates exterior would be the most susceptible to dissolving. But, unlike the NP aggregates from the loading experiment with 3% DOX hydrochloride, the NP on the aggregates’ exterior obtained in the loading experiments with higher amounts of drug would represent a low proportion of the total NP, taking into account the decrease of the area/volume ratio as the aggregates’ size increases. In this case the aggregates from the loading experiments with 5 and 10% drug would be composed of about 200 (value obtained following the same calculation procedure as in Section 3.2.1) and 480 NP, for respectively. Thus, most of the loaded NP located inside the aggregates would remain insoluble during the release period, requiring the DOX molecules to diffuse from the interior of the aggregates through an insoluble porous matrix.
The consequences of the behavior just described are very important for the possible application of this nanosystem for DOX delivery in malignant tumors. The main implication is that the aggregates of loaded NP with at least 4.38% DOX would retain a large part of their original size despite the prevailing pH 7.4 in the blood, at which the dissolution of copolymer chains would be expected. This conclusion is suggested by the slow release of the drug in the case of the loaded NP from the loading experiments with 5 and 10% DOX hydrochloride. An important factor to consider in this behavior is the larger size of the chains of our material compared to the chains of the ES 100 [Citation33], which would contribute to slow down its dissolution rate. If the chains had dissolved completely and rapidly, the release of the drug would have been similar to the free DOX [Citation12, Citation13]. However, it is believed that the aggregation of the NP is very important, since those on the outside delay the dissolution of those located on the inside. Thus, the aggregates of the loaded NP from the loading experiments with 5 ad 10% drug could be expected to circulate in the bloodstream for a sufficiently long time, enabling them to reach and penetrate tumors.
Once the aggregates of loaded NP, or even the individual loaded NP, were endocytosed by the malignant cells, in the event that the protonation of phenolic hydroxyl group of DOX [Citation8] at the prevailing acidic pH in these compartments is sufficiently intense, there is the possibility that they escape from the endosomes or lysosomes via the “proton sponge” effect [Citation41]. In the cytosol, which pH is close to 7.4, DOX would be released to exert its cytotoxic action.
Our short-term research on this topic includes experimentation in animal models to investigate the in vivo behavior of DOX-loaded NP. The information about pharmacokinetics and pharmacodynamics that would be obtained from that experimentation would allow us to identify the required modifications to the loaded NP to improve their behavior. Moreover, because the material of our NP is approved by the FDA, their application could be extended to the new field of nanoantioxidants, to be used in the preparation of antioxidant-loaded NP for prevention and treatment of toxicities induced by environmental pollutants [Citation42].
4. Conclusions
DOX was loaded in NP of copolymer P(MMA-co-MAA), in a MMA:MAA molar ratio of 0.64:0.36 with an initial Dn of 12.7 nm. QLS measurements showed an increase in the size of the loaded nanostructures size which does not correspond to the expected increase considering the amount of drug loaded. The analysis of the data suggests that the adsorption of DOX on the surface of the NP causes their instability, prompting their reversible aggregation in order to improve stability. As a result of this process, it was found that the aggregates of loaded NP with at least 4.38% DOX release the drug more slowly than that reported for free drug. The characteristics of the NP loaded with DOX described in this study suggest that they could remain for long periods in the bloodstream to reach and destroy cancer cells.
Acknowledgements
The authors are grateful to Judith Cabello Romero, Carmen Alvarado Canché, Ricardo Mendoza Carrizalez, María Guadalupe Méndez and Daniel Alvarado Medrano for their technical assistance; we also acknowledge to National Council of Science and Technology (CONACyT) for the PhD scholarship assigned to R. Lopez-Muñoz and to the Laboratorio Nacional de Materiales Grafénicos for the use of some of its analytical infrastructure.
Disclosure statement
No potential conflict of interest was reported by the authors.
Additional information
Funding
References
- Florey K. Analytical profiles of drug substances. In: Vigevani A, Williamson MJ, editors. Doxorubicin. Vol. 9. New York: Academic Press; 1981. p. 245–274.
- Deepa K, Singha S, Panda T. Doxorubicin nanoconjugates. J Nanosci Nanotechnol. 2014;14(1):892–904.
- Speth PAJ, van Hoesel QGCM, Haanen C. Clinical pharmacokinetics of doxorubicin. Clin Pharmacokinet. 1988;15(1):15–31.
- Prados J, Melguizo C, Ortiz R, et al. Doxorubicin-loaded nanoparticles: new advances in breast cancer therapy. Anticancer Agents Med Chem. 2012;12(9):1058–1070.
- Rivankar S. An overview of doxorubicin formulations in cancer therapy. J Cancer Res Ther. 2014;10(4):853–858.
- Janes KA, Fresneau MP, Marazuela A, et al. Chitosan nanoparticles as delivery systems for doxorubicin. J Control Release. 2001;73(2-3):255–267.
- Tian Y, Bromberg L, Lin SN, et al. Complexation and release of doxorubicin from its complexes with pluronic P85-b-poly(acrylic acid) block copolymers. J. Control Release. 2007;121(3):137–145.
- Betancourt T, Brandon B, Brannon-Peppas L. Doxorubicin-loaded PLGA nanoparticles by nanoprecipitation: preparation, characterization and in vitro evaluation. Nanomedicine. 2007;2(2):219–232.
- Du Y, Chen W, Zheng M, et al. pH-sensitive degradable chimaeric polymersomes for the intracellular release of doxorubicin hydrochloride. Biomaterials. 2012;33(29):7291–7299.
- Lv S, Li M, Tang Z, et al. Doxorubicin-loaded amphiphilic polypeptide-based nanoparticles as an efficient drug delivery system for cancer therapy. Acta Biomater. 2013;9(12):9330–9342.
- Cunningham AJ, Robinson M, Banquy X, et al. Bile acid-based drug delivery systems for enhanced doxorubicin encapsulation: comparing hydrophobic and ionic interactions in drug loading and release. Mol Pharm. 2018;15(3):1266–1276.
- Ma X, Shi X, Bai S, et al. Acid-activatable doxorubicin prodrug micelles with folate-targeted and ultra-high drug loading features for efficient antitumor drug delivery. J Mater Sci. 2018;53(2):892–907.
- Huang D, Zhou Y, Xiang Y, et al. Polyurethane/doxorubicin nanoparticles based on electrostatic interactions as pH-sensitive drug delivery carriers. Polym Int. 2018;67(9):1186–1193.
- Kayal S, Ramanujan RV. Doxorubicin loaded PVA coated iron oxide nanoparticles for targeted drug delivery. Mater Sci Eng C. 2010;30(3):484–490.
- Kievit FM, Wang FY, Fang C, et al. Doxorubicin loaded iron oxide nanoparticles overcome multidrug resistance in cancer in vitro. J Control Release. 2011;152(1):76–83.
- Ying XY, Cui D, Yu L, et al. Solid lipid nanoparticles modified with chitosan oligosaccharides for the controlled release of doxorubicin. Carbohyd Polym. 2011;84(4):1357–1364.
- Zou Y, Liu P, Liu CH, et al. Doxorubicin-loaded mesoporous magnetic nanoparticles to induce apoptosis in breast cancer cells. Biomed Pharmacother. 2015;69:355–360.
- Mosafer J, Teymouri M. Comparative study of superparamagnetic iron oxide/doxorubicin co-loaded poly (lactic-co-glycolic acid) nanospheres prepared by different emulsion solvent evaporation methods . Artif Cells Nanomed Biotechnol. 2018;46(6):1146–1155.
- Hosseini SH, Zohreh N, Karimi N, et al. Magnetic nanoparticles double wrapped into cross-linked salep/PEGylated carboxymethyl cellulose; a biocompatible nanocarrier for pH-triggered release of doxorubicin. Int J Biol Macromol. 2020;158:994–1006.
- Tartaj P, Morales MP, Veintemillas-Verdaguer S, et al. The preparation of magnetic nanoparticles for applications in biomedicine. J Phys D: Appl Phys. 2003;36(13):R182–R197.
- Elsabahy M, Wooley KL. Design of polymeric nanoparticles for biomedical delivery applications. Chem Soc Rev. 2012;41(7):2545–2561.
- Kissel T, Roser M. Influence of chemical surface-modification on the phagocytic properties of albumin nano-particles. In: Kellaway IW, editor. Proceedings of the 18th International Symposium Controlled Release Bioactive Materials; 1991 Jul 8-11; Amsterdam (Netherlands): Controlled Release Society; 1991. p. 275–276.
- Sunasee R, Adokoh CK, Darkwa J, et al. Therapeutic potential of carbohydrate-based polymeric and nanoparticle systems. Expert Opin Drug Deliv. 2014;11(6):867–884.
- Sonje A, Chandra A. Comprehensive review on Eudragit polymers. Int Res J Pharm. 2013;4(5):71–74.
- Liu Y, Fang Z, Chen Y, et al. The pharmacokinetic profile of freeze-dried cyclosporine A-Eudragit S100 nanoparticle formulation in dogs. Nano BioMed Eng. 2011;3(1):53–56.
- Yoo JW, Giri N, Lee CH. pH-sensitive Eudragit nanoparticles for mucosal drug delivery. Int J Pharm. 2011;403(1-2):262–267.
- Hu D, Liu L, Chen W, et al. A novel preparation method for 5-aminosalicylic acid loaded Eudragit S100 nanoparticles. Int J Mol Sci. 2012;13(5):6454–6468.
- Prakash SEL, Manavalan R. Development, characterization and/toxicity evaluation of nanoparticles of andrographolide. Int J Pharm Pharm Sci. 2012;4:497–501.
- Subramanian L, Ganesan V, Venkateshan N, et al. Design, formulation and evaluation of ofloxacin nanoparticles. Int J Biol Pharm Res. 2012;3(5):659–662.
- Sutar PS, Joshi VG. Gemcitabine loaded PLGA nanoparticles – an approach for colorectal cancer. Univers J Pharm. 2013;2:135–141.
- Vutpala S, Sailaja AK. Preparation and characterization of ibuprofen loaded polymeric nanoparticles by solvent evaporation technique. Int J Pharm Pharm Sci. 2014;6(8):416–421.
- Saade H, Diaz de León-Gómez R, Enríquez-Medrano FJ, et al. Preparation of ultrafine poly(methyl methacrylate-co-methacrylic acid) biodegradable nanoparticles loaded with ibuprofen. J Biomater Sci Polym Ed. 2016;27(11):1126–1138.
- López-Muñoz R, Treviño ME, Morales G, et al. Ultrafine nanoparticles of poly(methyl methacrylate-co-methacrylic acid) loaded with aspirin. J Nanomater. 2019;2019:1–9.
- Saade H, Guillén MdL, Romero JC, et al. Biocompatible and biodegradable ultrafine nanoparticles of poly(methyl methacrylate-co-methacrylic acid) prepared via semicontinuous heterophase polymerization: kinetics and product characterization. Int J Polym Sci. 2016;2016:1–8.
- DiazDuarte-Rodriguez M, Cortez-Lemus NA, Licea-Claverie A, et al. Dual responsive polymersomes for gold nanorod and doxorubicin encapsulation: nanomaterials with potential use as smart drug delivery systems. Polymers. 2019;11(6):939. [23 pages].
- Guo W, Hu N. Interaction of myoglobin with poly(methacrylic acid) at different pH in their layer-by-layer assembly films: an electrochemical study. Biophys Chem. 2007;129(2-3):163–171.
- Zhang J, Lin X, Liu J, et al. Sequential thermo-induced self-gelation and acid-triggered self-release process of drug-conjugated nanoparticles: a strategy for the sustained and controlled drug delivery to tumors. J Mater Chem B. 2013;1(36):4667–4677.
- Li X, Gao C, Wu Y, et al. Combination delivery of adjudin and doxorubicin via integrating drug conjugation and nanocarrier approaches for the treatment of drug-resistant cancer cells. J Mater Chem B. 2015;3(8):1556–1564.
- Piorecka K, Stanczyk W, Florczak M. NMR analysis of antitumor drugs: doxorubicin, daunorubicin and their functionalized derivatives. Tetrahedron Lett. 2017;58(2):152–155.
- Shoaib MH, Tazeen J, Merchant HA, et al. Evaluation of drug release kinetics from ibuprofen matrix tablets using HPMC. Pak J Pharm Sci. 2006;19:119–124.
- Liu CG, Han YH, Kankala RK, et al. Subcellular performance of nanoparticles in cancer therapy. Int J Nanomedicine. 2020;15:675–704.
- Eftekhari A, Maleki Dizaj S, Chodari L, et al. The promising future of nano-antioxidant therapy against environmental pollutants induced-toxicities. Biomed Pharmacother. 2018;103:1018–1027.